Mechanisms underlying genome instability mediated by formation of foldback inversions in Saccharomyces cerevisiae
Figures
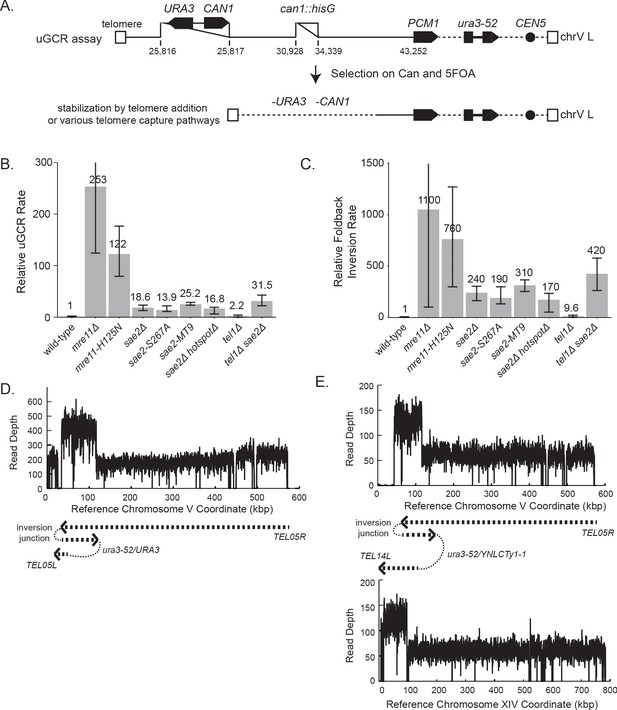
GCRs recovered in uGCR strains with sae2 defects are primarily hairpin-mediated foldback inversions.
(A) Diagram of the left arm of chromosome V (chrV L) containing the uGCR assay. The normal CAN1 locus is deleted by substitution with a hisG fragment and a cassette containing the CAN1 and URA3 genes has been inserted into the YEL068C gene. Coordinates for the modified chrV L are reported as the reference genome coordinates for unmodified regions or reported as positions relative to the centromeric coordinate of the insertion site for inserted elements, for example chrV:34,339–110 is a position in the inserted hisG fragment 110 bases telomeric to the insertion site at chrV 34,339. Simultaneous selection against CAN1 and URA3 by canavanine (Can) and 5-fluoroorotic acid (5FOA) selects for GCRs that ultimately lose both CAN1 and URA3 and are stabilized by addition or capture of a telomere. (B) The relative uGCR rate for strains with mre11 or sae2 defects are displayed with error bars corresponding to the 95% confidence intervals. GCR rates are reported in Supplementary file 1. (C) The relative foldback inversion GCR rate for strains with mre11 or sae2 defects are displayed as in panel B. (D) Example read depth plot determined by WGS for chrV from a foldback inversion GCR resolved by the ura3-53/URA3 homology-mediated rearrangement. Thick-hashed arrows underneath the plot indicate the connectivity between the portions of the GCR that map to the different regions of the reference chromosome. (E) Example read depth plot for chrV and chrXIV from a foldback inversion GCR resolved by a ura3-52/YNLCTy1-1 homology-mediated rearrangement displayed as in panel D, showing the duplication of the region of chrXIV between TEL14L and YNLCTy1-1.
-
Figure 1—source data 1
Complex GCR structures.
GCR structures that are more complicated than those displayed in Figures 1D and 1E, and Figure 1—figure supplement 1 are displayed using the same scheme as those displayed in Figure 1—figure supplement 1. Panels A to U are sorted by the PGSP isolate number. The regions included in the GCR-containing chromosome are displayed using the red dashed arrow (oriented from chrV R to chrV L in the GCR-containing chromosome). The black dotted lines show the connectivity between regions included in the GCR-containing chromosome that are separate in the reference genome. Telomeres added to the chrV L side of the GCR are displayed using black boxes.
- https://cdn.elifesciences.org/articles/58223/elife-58223-fig1-data1-v2.pdf
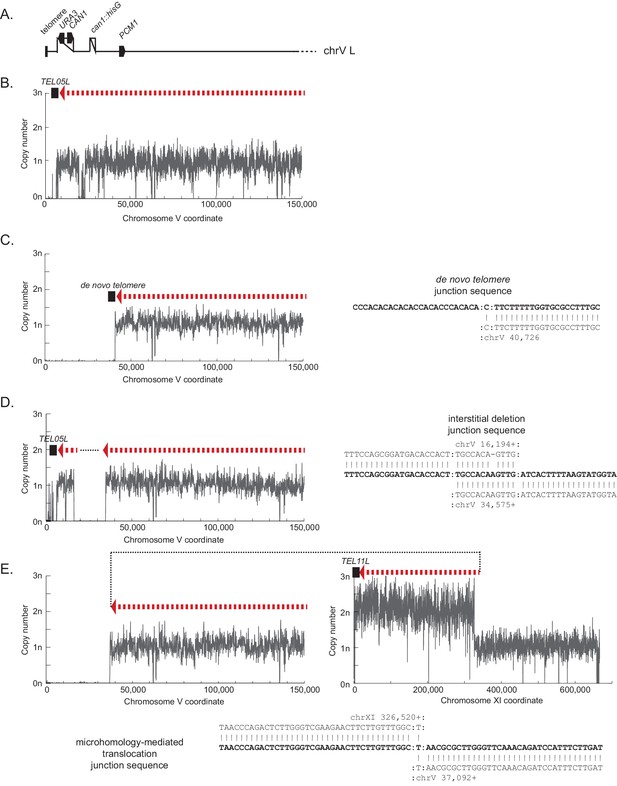
Example copy number plots for simple GCR structures not involving foldback inversions.
The example copy number plots for the foldback inversion GCRs are displayed in Figure 1D and Figure 1E. The regions included in the GCR-containing chromosome are displayed using the red dashed arrow (oriented from chrV R to chrV L in the GCR-containing chromosome). The black dotted lines show the connectivity between regions included in the GCR-containing chromosome that are separate in the reference genome. Telomeres added to the chrV L side of the GCR are displayed using black boxes. (A) The diagram of the uGCR chrV L assay is drawn to scale for the copy number plots below. (B) The copy number plot (read depth at each base in the uniquely mapping regions of the genome divided by the median read depth of the nuclear chromosomes) for chrV L in a parental strain that has not formed a GCR. (C) The copy number plot of an example of a de novo telomere addition GCR (PGSP2215, isolated from a pif1Δ strain). (D) The copy number plot of an example of an interstitial deletion (PGSP5065, isolated from an exo1Δ yku80Δ strain). (E) The copy number plot of an example of a microhomology-mediated translocation with chrV L on left and the duplicated target (in this case chrXI) on the right (PGSP940, isolated from a sgs1Δ strain). Note that the duplication of the region of chrXI L indicates that the translocation-containing GCR is present in addition to an intact chrXI.
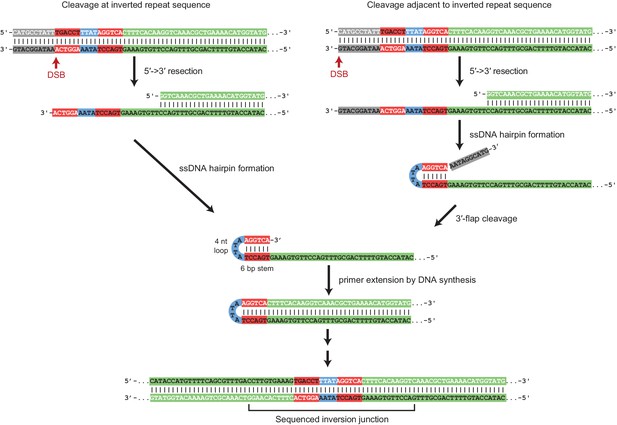
Foldback inversion GCRs are mediated by a ssDNA hairpin intermediate.
Proposed mechanism underlying foldback inversion formation based on the inversion junction sequences recovered. 5’ to 3’ resection from a DSB or other initiating form of DNA damage exposes a ssDNA region predicted to form a ssDNA hairpin. Appropriately positioned DSBs can lead to properly paired hairpin stems, whereas other DSBs will lead to 3’ flaps that require processing before extension by DNA polymerases. Extension of the hairpin leads to the inversion junction sequences observed.
-
Figure 2—source data 1
Predicted ssDNA hairpin structures for observed foldback inversions.
The structures of the key predicted ssDNA hairpin intermediates observed (panels A to BK are sorted by the chromosomal position of the predicted hairpin). The chrV coordinates for each predicted hairpin correspond to the coordinates reported for the foldback inversion-containing GCRs.
- https://cdn.elifesciences.org/articles/58223/elife-58223-fig2-data1-v2.pdf
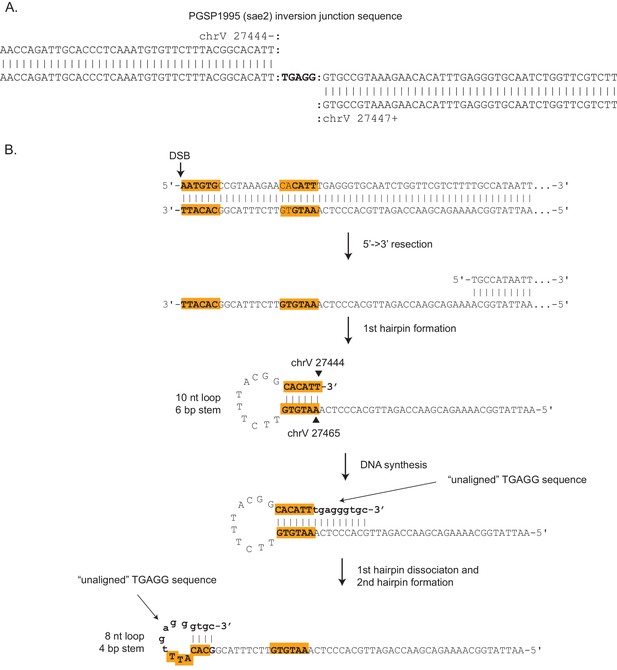
Inversion junction formed by two sequential ssDNA hairpin intermediates.
(A) The inversion sequence junction from PGSP1995 (center line), which was present in a GCR from a sae2Δ mutant, shows an unusual feature relative to other inversion junction sequences recovered as it contains a five base TGAGG sequence that does not align to either inverted sequence from chrV. For most inversion junction sequences, the two alignments to chrV overlap, and the overlap indicates the sequences involved in the ssDNA hairpin stem. Thus, this inversion junction sequence must involve a different mechanism of formation. (B) A mechanism of formation of the PGSP1995 inversion junction that is consistent with the junction sequence identified involves two sequential ssDNA hairpins. After resection from a DSB (or other initiating damage), the first hairpin forms by annealing the stem sequences (highlighted in orange) to generate a chrV:27,444_27,465 hairpin with a 10 nt loop and 6 bp stem. DNA synthesis after formation of the first hairpin adds the unaligned TGAGG sequence and bases required for forming the stem of a second hairpin. Dissociation of the first hairpin and formation of a second hairpin (eight nt loop, 4 bp stem) using the newly synthesized bases generates an intermediate that after extension generates an inversion junction sequence identical to the one shown in panel A.
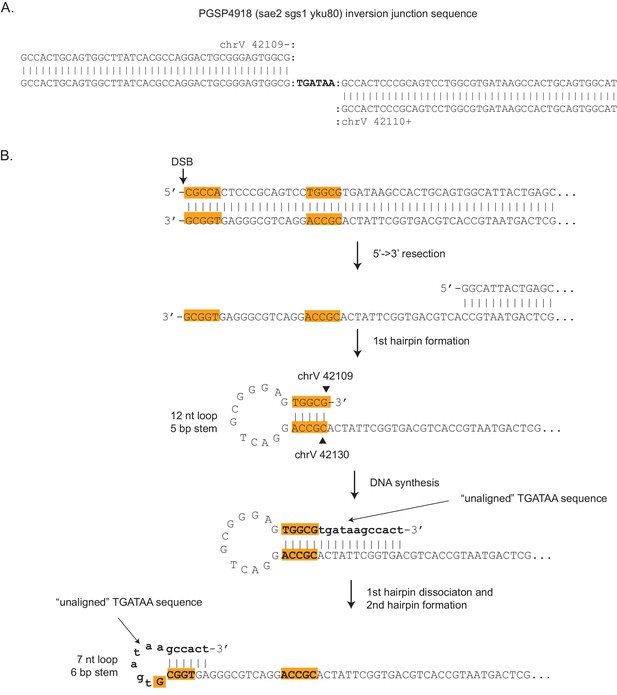
Inversion junction formed by two sequential ssDNA hairpin intermediates.
(A) The inversion sequence junction from PGSP4918 (center line), shows an unusual feature relative to other inversion junction sequences recovered as it contains a six base TGATAA sequence that does not align to either inverted sequence from chrV. For most inversion junction sequences, the two alignments to chrV overlap, and the overlap indicates the sequences involved in the ssDNA hairpin stem. Thus, this inversion junction sequence must involve a different mechanism of formation. (B) A mechanism of formation of the PGSP4918 inversion junction that is consistent with the junction sequence identified involves two sequential ssDNA hairpins.
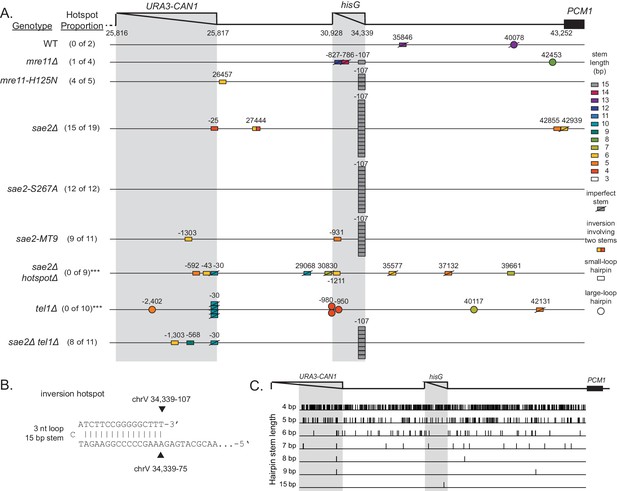
Distribution of inversion junctions in mre11 and sae2 mutants.
(A) Map of the position of the inversion junctions (boxes) reveals that an inversion hotspot in the hisG insertion mediates a large proportion of the foldback inversions in strains with mre11 and sae2 defects. Each observed inversion is represented by a separate box (short loop hairpin) or separate circle (large loop hairpin) at the inversion junction position; for example, the 15 grey boxes corresponding to the inversion hotspot (labeled ‘−107’) on the sae2Δ line correspond to 15 independent GCR-containing strains isolated from the sae2Δ single mutant that have an inversion at the hotspot. The only mutants whose usage of the inversion hotspot relative to other inversion sites was altered relative to the sae2Δ single mutant control were the sae2Δ hotspotΔ and tel1Δ mutants (p=0.0002 and p=5×10−5; Fisher’s exact test). ‘Imperfect stem’ indicates stems predicted to contain one or more mispairs or unpaired bases by MFOLD (Zuker, 2003). (B) The predicted ssDNA hairpin for the inversion hotspot is predicted by MFOLD to form a 15 bp stem with a three nt loop. (C) The inversion hotspot contains the longest stem structure for any of the theoretically predicted sites with a propensity to form ssDNA hairpins in the uGCR chrV L breakpoint region. Predicted hairpin sites were restricted to a loop size of <50 nt and were not allowed to have mismatches in the stems.
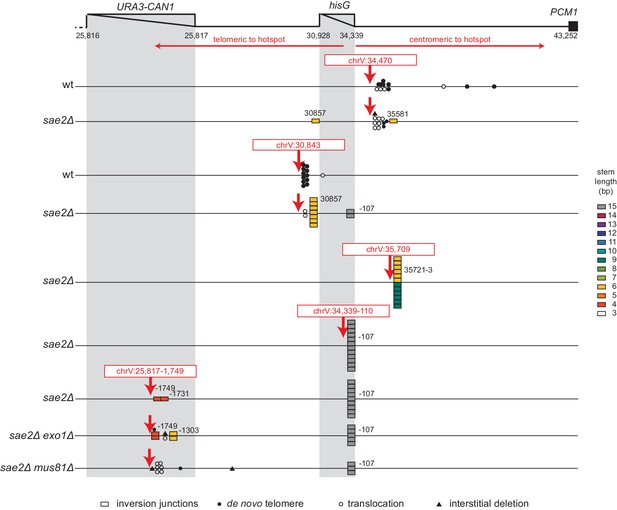
Analysis of GCRs generated by induction of site-specific DSBs.
The position of CRISPR/Cas9 cleavage sites are indicated by red arrows. The sites at which various rearrangements were induced are indicated by different symbols: inversion junctions are shown as boxes, de novo telomere additions as filled circles, microhomology-mediated translocations as open circles, and microhomology-mediated interstitial deletions as filled triangles. The relevant genotype of the strains where the DSBs were induced is indicated on the left side.
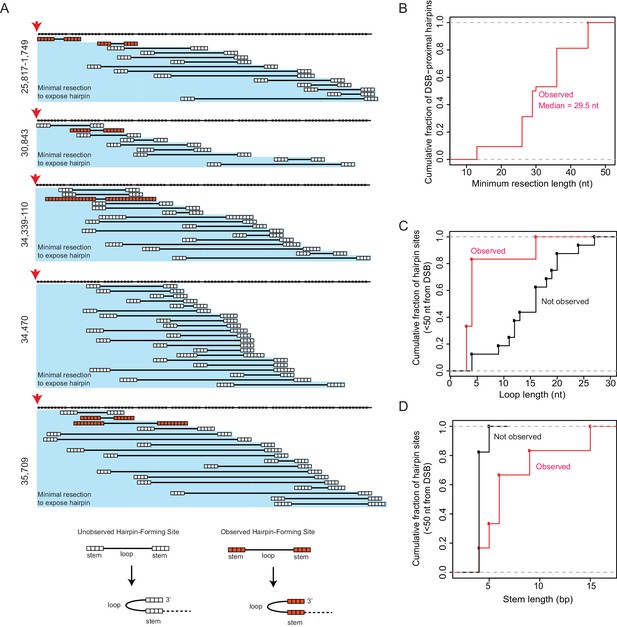
Distribution of potential hairpin-forming sites centromeric to the induced DSBs.
(A) For each of the 5 CRISPR/Cas9-induced DSB sites, the bases centromeric to the DSBs (red arrows) are shown as black squares across the top line; every 10th base from the DSB is not displayed. Below the top line, potential hairpins are shown such that bases associated with the stems are white squares (an unobserved hairpin) or orange squares (an observed hairpin) and bases associated with the loop are shown as solid black lines (see the bottom of the panel). The minimal amount of resection required to expose all of the bases of the potential hairpin are shown with the blue background. Only potential hairpins that are exposed by 100 nt or less of centromeric resection from the induced DSB are shown. (B) A plot of the cumulative distribution of required resection lengths for all of the observed hairpin-forming sites displayed in panel A; the maximum observed resection is less than 50 nt, and the median is 29.5 nt. (C) The cumulative distribution of hairpin loop lengths for observed hairpin-forming sites (red) and hairpin-forming sites that were not observed (black) exposed by 50 nt or less resection on the centromeric side of the induced DSBs. (D) The cumulative distribution of hairpin stem lengths for observed hairpin-forming sites (red) and hairpin-forming sites that were not observed (black) exposed by 50 nt or less resection on the centromeric side of the induced DSBs.
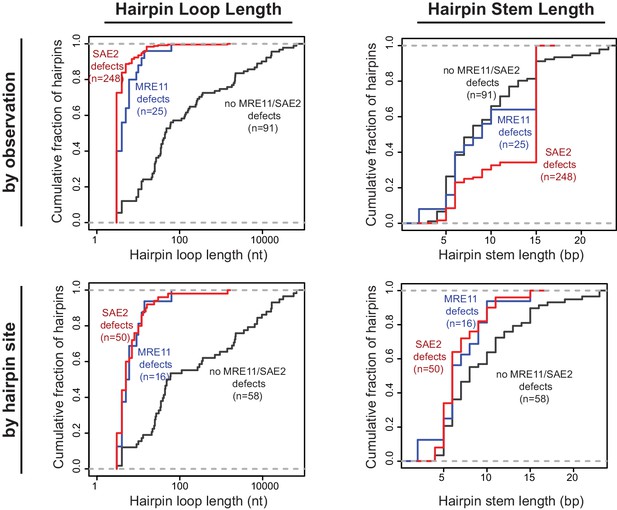
Distribution of the loop and stem sizes of the predicted ssDNA hairpins.
Cumulative distributions for the loop lengths (left) and hairpin stem lengths (right) for the predicted ssDNA hairpins derived from the inversion junction sequences determined. Hairpins from strains proficient for both MRE11 and SAE2 (black), deficient for MRE11 (blue), and deficient for SAE2 (red) are plotted separately. Distributions were calculated by counting each observed rearrangement once (top) and by counting each observed inversion site once (bottom). As an example of the difference between the hairpin loop sizes seen in mutants that give rise to small loop and large loop hairpins, the loop size distributions seen in sae2Δ and tel1Δ mutants are (number of occurrences in parentheses): sae2Δ - 3 nt (16), 4 nt (1), 5 nt (1), 8 nt (1), 10 nt (1); and, tel1Δ - 4 nt (1), 10 nt (4), 25 nt (1), 35 nt (2), 39 nt (1), 44 nt (1).
-
Figure 5—source data 1
Properties of the ssDNA hairpins from observed foldback inversions.
- https://cdn.elifesciences.org/articles/58223/elife-58223-fig5-data1-v2.xlsx
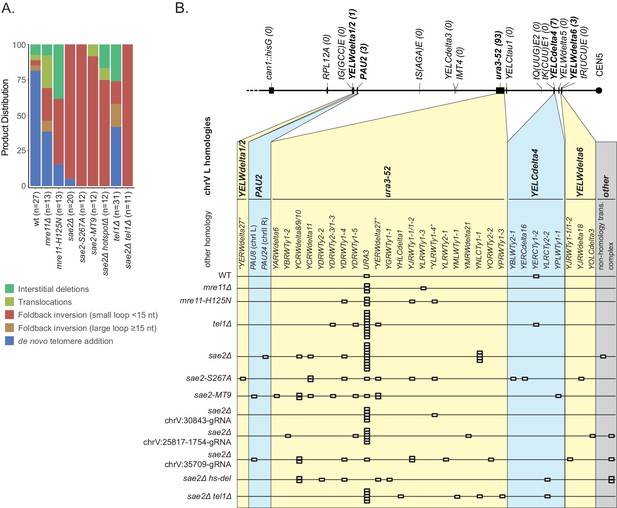
Analysis of the GCRs generated in MRE11, SAE2, and TEL1 mutants.
(A) Comparison of the observed GCR spectra. (B) Distribution of foldback inversion resolution products observed by genotype. Yellow and blue backgrounds distinguish homologies on chrV L involved in the rearrangement, and columns indicate homologies involved in other regions of the genome. Grey background indicates either non-homology-mediated resolution products or those involving multiple steps, ‘complex’.
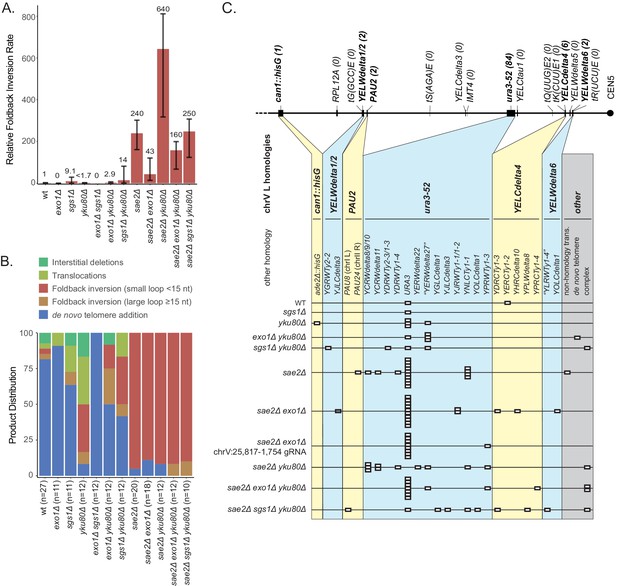
Analysis of GCRs generated in strains with mutations in genes that have potential roles in resection.
(A) Comparison of the foldback inversion GCR rates relative to wild-type; the foldback inversion rate reported for the yku80Δ mutant is the upper bound estimated from the fluctuation results. (B) Comparison of the observed GCR spectra. (C) Distribution of foldback inversion resolution products observed by genotype. Yellow and blue backgrounds distinguish homologies on chrV L involved in the rearrangement, and columns indicate homologies involved in other regions of the genome. Grey background indicates either non-homology mediated resolution products or those involving multiple steps, ‘complex’.
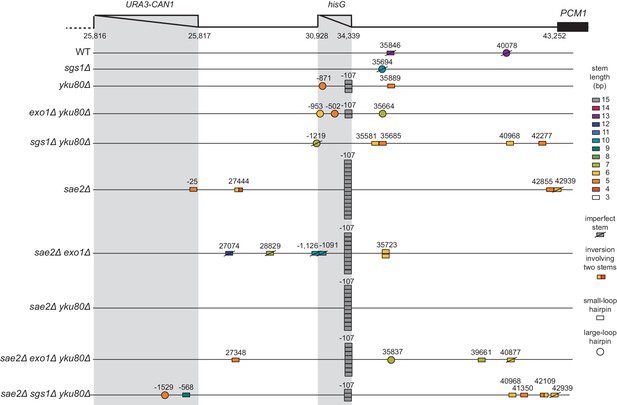
Distribution of inversion junctions identified in strains with defects in resection.
Map of the position of the inversion junctions (boxes). ‘Imperfect stem’ indicates stems predicted to contain one or more mispairs or unpaired bases by MFOLD.
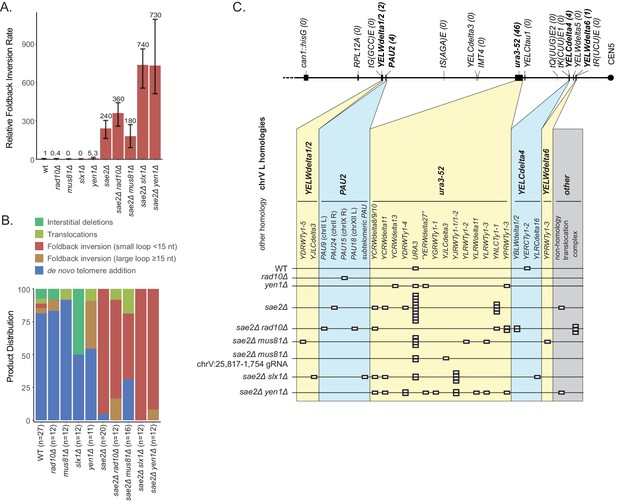
Analysis of GCRs generated in strains with mutations in genes encoding flap endonucleases.
(A) Comparison of the foldback inversion GCR rates relative to wild-type. (B) Comparison of the observed GCR spectra. (C) Distribution of foldback inversion resolution products observed by genotype. Yellow and blue backgrounds distinguish homologies on chrV L involved in the rearrangement, and columns indicate homologies involved in other regions of the genome. Grey background indicates either non-homology-mediated resolution products or those involving multiple steps, ‘complex’.
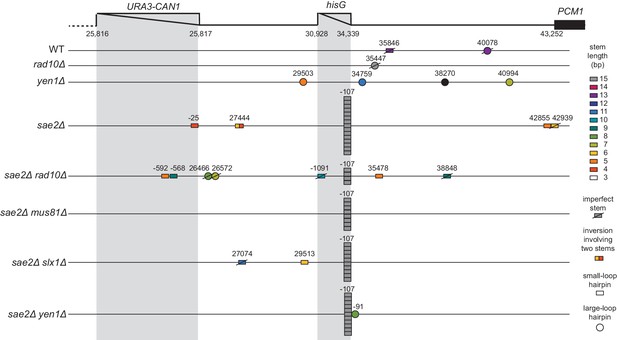
Distribution of inversion junctions identified in strains with defects in genes encoding flap endonucleases.
Map of the position of the inversion junctions (boxes). ‘Imperfect stem’ indicates stems predicted to contain one or more mispairs or unpaired bases by MFOLD.
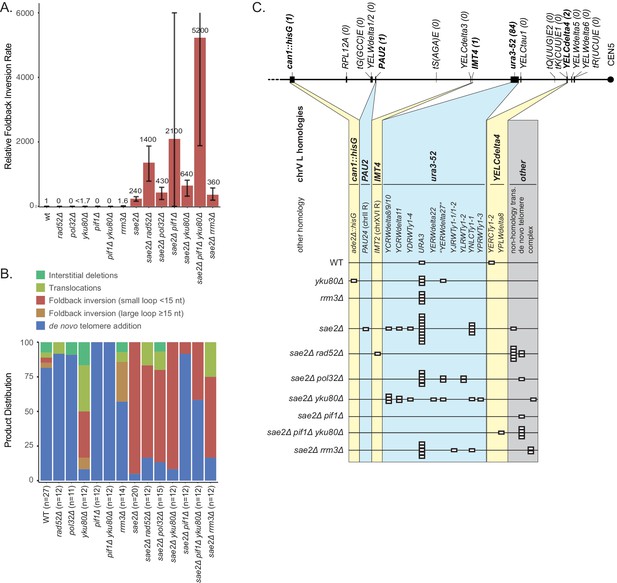
Analysis of GCRs generated in strains with mutations in genes that have potential roles in recombination and BIR.
(A) Comparison of the foldback inversion GCR rates relative to wild-type; the foldback inversion rate reported for the yku80Δ mutant is the upper bound estimated from the fluctuation results. (B) Comparison of the observed GCR spectra. (C) Distribution of foldback inversion resolution products observed by genotype. Yellow and blue backgrounds distinguish homologies on chrV L involved in the rearrangement, and columns indicate homologies involved in other regions of the genome. Grey background indicates either non-homology-mediated resolution products or those involving multiple steps, ‘complex’.
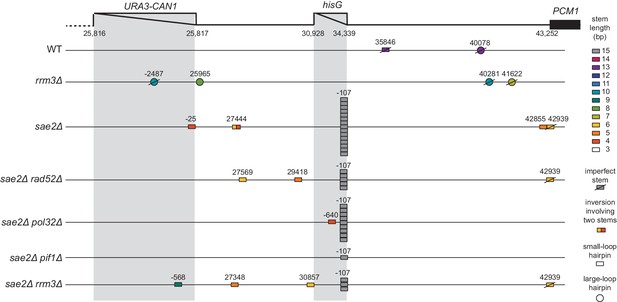
Distribution of inversion junctions identified in strains with defects in break-induced replication.
Map of the position of the inversion junctions (boxes). ‘Imperfect stem’ indicates stems predicted to contain one or more mispairs or unpaired bases by MFOLD.
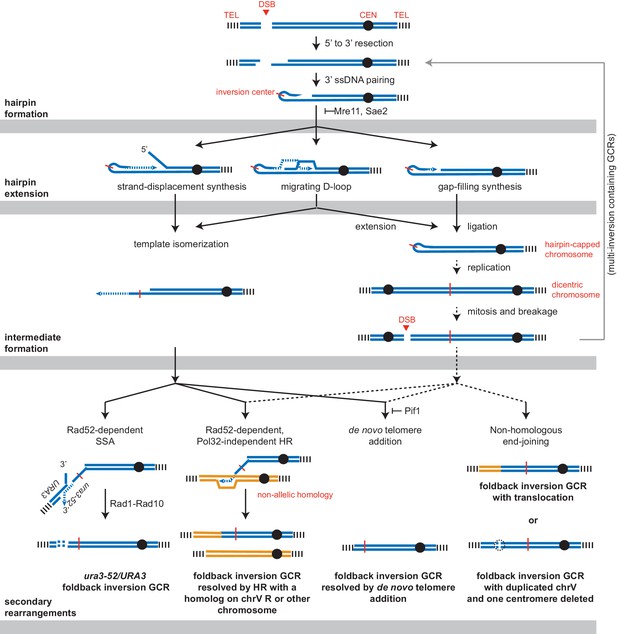
Pathways implicated in the formation of foldback inversion GCRs.
DNA damage, which is not limited to DSBs, can initiate hairpin formation. Hairpin extension by DNA synthesis could occur by several mechanisms: strand-displacement synthesis, a migrating D-loop, or simple gap-filling synthesis. These mechanisms generate two major types of intermediates: a centromere-containing chromosome fragment with a partially extended and dynamically available 3' ssDNA end or, after DNA replication, a dicentric chromosome V which undergoes breakage during mitosis. The 3' ssDNA end or the broken dicentric chromosome can then participate in multiple types of secondary rearrangements ultimately yielding a monocentric GCR with telomeres at both ends. These rearrangements can be formed in a single step if the resulting GCR is monocentric; however, many of the complex GCRs observed are consistent with multiple rounds of rearrangement and likely involve additional dicentric intermediates (not shown). Most resolution products can be generated from either class of intermediate; however, the ura3-52/URA3 product, GCRs with multiple foldback inversions, and GCRs that duplicate all of chrV and delete of one of the two centromeres are suggestive of only one of the two proposed intermediates, as indicated.
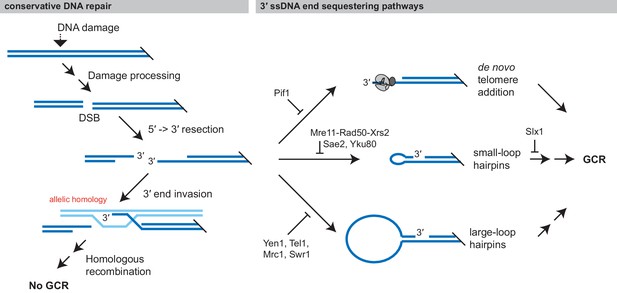
Formation of GCRs is promoted by sequestering 3' ssDNA ends.
Conservative repair of many types of DNA damage involves processing the DNA damage to yield a DSB, which is then resected and undergoes allelic HR with the sister chromatid. Allelic HR requires that the 3' ssDNA ends of the DSB are available to initiate HR. GCRs form when intermediates in conservative repair are acted on by competing DNA processing pathways, particularly if these pathways sequester the 3' ssDNA end from allelic HR. Analysis of the structure of GCRs, including the analysis presented here has identified three major 3' ssDNA sequestering pathways, which are suppressed by distinct gene products: de novo telomere addition, formation of small-loop ssDNA hairpins, and formation of large-loop ssDNA hairpins.
Tables
Reagent type (species) or resource | Designation | Source or reference | Identifiers | Additional information |
---|---|---|---|---|
Strain, strain background (S. cerevisiae) | RDKY6677 | PMID:19641493 | uGCR wild-type | Dr. Richard Kolodner (Ludwig Institute for Cancer Research) |
Strain, strain background (S. cerevisiae) | RDKY6729 | PMID:19641493 | uGCR exo1Δ::HIS3 | Dr. Richard Kolodner (Ludwig Institute for Cancer Research) |
Strain, strain background (S. cerevisiae) | RDKY8032 | PMID:24699249 | uGCR exo1Δ::TRP1 sgs1Δ::HIS3 | Dr. Richard Kolodner (Ludwig Institute for Cancer Research) |
Strain, strain background (S. cerevisiae) | RDKY9771 | This study | uGCR exo1Δ::HIS3 yku80Δ::kanMX4 | Dr. Richard Kolodner (Ludwig Institute for Cancer Research) |
Strain, strain background (S. cerevisiae) | RDKY6686 | PMID:19641493 | uGCR mre11Δ::HIS3 | Dr. Richard Kolodner (Ludwig Institute for Cancer Research) |
Strain, strain background (S. cerevisiae) | HZY2771 | PMID:29505562 | uGCR cir0 mre11-H125N::TRP1 | Dr. Huilin Zhou (Ludwig Institute for Cancer Research) |
Strain, strain background (S. cerevisiae) | RDKY6731 | PMID:19641493 | uGCR mus81Δ::HIS3 | Dr. Richard Kolodner (Ludwig Institute for Cancer Research) |
Strain, strain background (S. cerevisiae) | RDKY6894 | PMID:24699249 | uGCR pif1Δ::HIS3 | Dr. Richard Kolodner (Ludwig Institute for Cancer Research) |
Strain, strain background (S. cerevisiae) | RDKY9773 | This study | uGCR pif1Δ::HIS3 yku80Δ::kanMX4 | Dr. Richard Kolodner (Ludwig Institute for Cancer Research) |
Strain, strain background (S. cerevisiae) | RDKY6703 | PMID:19641493 | uGCR pol32Δ::TRP1 | Dr. Richard Kolodner (Ludwig Institute for Cancer Research) |
Strain, strain background (S. cerevisiae) | RDKY6734 | PMID:19641493 | uGCR rad10Δ::HIS3 | Dr. Richard Kolodner (Ludwig Institute for Cancer Research) |
Strain, strain background (S. cerevisiae) | RDKY6691 | PMID:19641493 | uGCR rad52Δ::HIS3 | Dr. Richard Kolodner (Ludwig Institute for Cancer Research) |
Strain, strain background (S. cerevisiae) | RDKY6735 | PMID:19641493 | uGCR rrm3Δ::HIS3 | Dr. Richard Kolodner (Ludwig Institute for Cancer Research) |
Strain, strain background (S. cerevisiae) | RDKY6687 | PMID:19641493 | uGCR sgs1Δ::HIS3 | Dr. Richard Kolodner (Ludwig Institute for Cancer Research) |
Strain, strain background (S. cerevisiae) | RDKY9445 | This study | uGCR sgs1Δ::HIS3 yku80Δ::kanMX4 | Dr. Richard Kolodner (Ludwig Institute for Cancer Research) |
Strain, strain background (S. cerevisiae) | RDKY6738 | PMID:19641493 | uGCR slx1Δ::kanMX4 | Dr. Richard Kolodner (Ludwig Institute for Cancer Research) |
Strain, strain background (S. cerevisiae) | RDKY6761 | PMID:19641493 | uGCR tel1Δ::HIS3 | Dr. Richard Kolodner (Ludwig Institute for Cancer Research) |
Strain, strain background (S. cerevisiae) | RDKY9506 | This study | uGCR yen1Δ::HIS3 | Dr. Richard Kolodner (Ludwig Institute for Cancer Research) |
Strain, strain background (S. cerevisiae) | RDKY8006 | PMID:24699249 | uGCR yku80Δ::HIS3 | Dr. Richard Kolodner (Ludwig Institute for Cancer Research) |
Strain, strain background (S. cerevisiae) | RDKY6737 | PMID:24699249 | uGCR sae2Δ::TRP1 | Dr. Richard Kolodner (Ludwig Institute for Cancer Research) |
Strain, strain background (S. cerevisiae) | RDKY9734 | This study | uGCR sae2Δ::TRP1 hotspotΔ | Dr. Richard Kolodner (Ludwig Institute for Cancer Research) |
Strain, strain background (S. cerevisiae) | RDKY9472 | This study | uGCR sae2-S267A | Dr. Richard Kolodner (Ludwig Institute for Cancer Research) |
Strain, strain background (S. cerevisiae) | RDKY9496 | This study | uGCR sae2-MT9 | Dr. Richard Kolodner (Ludwig Institute for Cancer Research) |
Strain, strain background (S. cerevisiae) | RDKY8020 | PMID:24699249 | uGCR sae2Δ::TRP1 exo1Δ::HIS3 | Dr. Richard Kolodner (Ludwig Institute for Cancer Research) |
Strain, strain background (S. cerevisiae) | RDKY9777 | This study | uGCR sae2Δ::TRP1 exo1Δ::HIS3 yku80Δ:kanMX4 | Dr. Richard Kolodner (Ludwig Institute for Cancer Research) |
Strain, strain background (S. cerevisiae) | RDKY9392 | This study | uGCR sae2Δ::kanMX4 mus81Δ::HIS3 | Dr. Richard Kolodner (Ludwig Institute for Cancer Research) |
Strain, strain background (S. cerevisiae) | RDKY9447 | This study | uGCR sae2Δ::TRP1 pif1Δ::HIS3 | Dr. Richard Kolodner (Ludwig Institute for Cancer Research) |
Strain, strain background (S. cerevisiae) | RDKY9779 | This study | uGCR sae2Δ::TRP1 pif1Δ::HIS3 yku80Δ::kanMX4 | Richard Kolodner (Ludwig Institute for Cancer Research) |
Strain, strain background (S. cerevisiae) | RDKY9390 | This study | uGCR sae2Δ::kanMX4 pol32Δ::HIS3 | Richard Kolodner (Ludwig Institute for Cancer Research) |
Strain, strain background (S. cerevisiae) | RDKY9123 | This study | uGCR sae2Δ::TRP1 rad10Δ::HIS3 | Richard Kolodner (Ludwig Institute for Cancer Research) |
Strain, strain background (S. cerevisiae) | RDKY9504 | This study | uGCR sae2Δ::TRP1 rad52Δ::HIS3 | Richard Kolodner (Ludwig Institute for Cancer Research) |
Strain, strain background (S. cerevisiae) | RDKY9125 | This study | uGCR sae2Δ::TRP1 rrm3Δ::HIS3 | Richard Kolodner (Ludwig Institute for Cancer Research) |
Strain, strain background (S. cerevisiae) | RDKY9775 | This study | uGCR sae2Δ::TRP1 sgs1Δ::HIS3 yku80Δ::kanMX4 | Richard Kolodner (Ludwig Institute for Cancer Research) |
Strain, strain background (S. cerevisiae) | RDKY9502 | This study | uGCR sae2Δ::TRP1 slx1Δ::HIS3 | Richard Kolodner (Ludwig Institute for Cancer Research) |
Strain, strain background (S. cerevisiae) | RDKY8018 | This study | uGCR sae2Δ::TRP1 tel1Δ::HIS3 | Richard Kolodner (Ludwig Institute for Cancer Research) |
Strain, strain background (S. cerevisiae) | RDKY9500 | This study | uGCR sae2Δ::TRP1 yen1Δ::HIS3 | Richard Kolodner (Ludwig Institute for Cancer Research) |
Strain, strain background (S. cerevisiae) | RDKY9443 | This study | uGCR sae2Δ::TRP1 yku80Δ::kanMX4 | Richard Kolodner (Ludwig Institute for Cancer Research) |
Recombinant DNA reagent | bRA77 | PMID:28405019 | Dr. James Haber (Brandeis University) | |
Recombinant DNA reagent | pRS425-Cas9-2XSapI | Dr. Bruce Futcher (State University of New York, Stoney Brook) | ||
Recombinant DNA reagent | pRS315/sae2-MT9 | PMID:24699249 | Dr. Richard Kolodner (Ludwig Institute for Cancer Research) | |
Recombinant DNA reagent | pRDK1923 | This study | Dr. Richard Kolodner (Ludwig Institute for Cancer Research) | |
Recombinant DNA reagent | pRDK1924 | This study | Dr. Richard Kolodner (Ludwig Institute for Cancer Research) | |
Recombinant DNA reagent | pRDK1929 | This study | Dr. Richard Kolodner (Ludwig Institute for Cancer Research) | |
Recombinant DNA reagent | pRDK1938 | This study | Dr. Richard Kolodner (Ludwig Institute for Cancer Research) | |
Recombinant DNA reagent | pRDK1939 | This study | Dr. Richard Kolodner (Ludwig Institute for Cancer Research) | |
Recombinant DNA reagent | pRDK1940 | This study | Dr. Richard Kolodner (Ludwig Institute for Cancer Research) | |
Recombinant DNA reagent | pRDK1941 | This study | Dr. Richard Kolodner (Ludwig Institute for Cancer Research) | |
Sequence-based reagent | pRDK1923-top | This study | 5′-ATC AAT AGA TCA AAA TCC CCC CC-3′ | |
Sequence-based reagent | pRDK1924-bottom | This study | 5′-AAC GGG GGG GAT TTT GAT CTA TT-3′ | |
Sequence-based reagent | pRDK1924-top | This study | 5′-ATC TTG GCT CTG GTC AAT GAT TA-3′ | |
Sequence-based reagent | pRDK1924-bottom | This study | 5′-AAC TAA TCA TTG ACC AGA GCC AA-3′ | |
Sequence-based reagent | pRDK1924-top | This study | 5′-ATC TGA ACG CAT GAG AAA GCC CC-3′ | |
Sequence-based reagent | pRDK1294-bottom | This study | 5′-AAC GGG GCT TTC TCA TGC GTT CA-3′ | |
Sequence-based reagent | pRDK1929-top | This study | 5′-TCC GTG TTC CAT CCT ACA GAG TTT T-3′ | |
Sequence-based reagent | pRDK1929-bottom | This study | 5′-TCT GTA GGA TGG AAC ACG GAG ATC A-3′ | |
Sequence-based reagent | pRDK1938-top | This study | 5′-TTA CAT GTT CGA CCG TAC CCG TTT T-3′ | |
Sequence-based reagent | pRDK1938-bottom | This study | 5′-GGG TAC GGT CGA ACA TGT AAG ATC A-3′ | |
Sequence-based reagent | pRDK1939-top | This study | 5′-ATA CCT GGA CCC CAG GCA CCG TTT T-3′ | |
Sequence-based reagent | pRDK1939-bottom | This study | 5′-GGT GCC TGG GGT CCA GGT ATG ATC A-3′ | |
Sequence-based reagent | pRDK1940-top | This study | 5′-TCA AAT AGG CAT GAT CTT GTG TTT T-3′ | |
Sequence-based reagent | pRDK1940-bottom | This study | 5′-ACA AGA TCA TGC CTA TTT GAG ATC A-3′ | |
Sequence-based reagent | pRDK1941-top | This study | 5′-TCT TCC GGG GGC TTT TTT TTG TTT T-3′ | |
Sequence-based reagent | pRDK1941-bottom | This study | 5′-AAA AAA AAG CCC CCG GAA GAG ATC A-3′ | |
Sequence-based reagent | sae2-S267A repair fragment | This study | 5′-TGA TAA CTT GAG GAA TAG ATC AAA AGC GCC CCC AGG TTT TGG AAG ACT GGA TTT TCC CTC-3′ | |
Sequence-based reagent | sae2-MT9 amplification forward primer | This study | 5′-TCC ACC ATT CGA GTC TTG AG-3′ | |
Sequence-based reagent | sae2-MT9 amplification reverse primer | This study | 5′-TTC CCC TTT CTG CTT TAC CA-3′ | |
Sequence-based reagent | hotspotΔ repair fragment | This study | 5′-TCA AGA ATT CAG ATC TTC CAG TGG TGC ATG AAC GCA TGA GGG CGC GCG ATA CAG ACC GGT TCA GAC AGG ATA AAG AGG AA-3′ | |
Commercial assay or kit | Gentra Puregene Yeast/Bacteria Kit | Qiagen | 158567 | |
Commercial assay or kit | TruSeq DNA PCR-free LT kit | Illumina | 15037158 | |
Software, algorithm | Bowtie 2.2.1 | PMID:22388286 | http://bowtie-bio.sourceforge.net/bowtie2/index.shtml | |
Software, algorithm | Pyrus 0.7 | PMID:24699249 | https://sourceforge.net/projects/pyrus-seq/ |
Additional files
-
Supplementary file 1
Measured uGCR rates, GCR spectra, and calculated foldback inversion rates for strains.
- https://cdn.elifesciences.org/articles/58223/elife-58223-supp1-v2.xlsx
-
Supplementary file 2
Statistics for Whole Genome Sequencing results.
- https://cdn.elifesciences.org/articles/58223/elife-58223-supp2-v2.docx
-
Supplementary file 3
GCR structures.
- https://cdn.elifesciences.org/articles/58223/elife-58223-supp3-v2.docx
-
Supplementary file 4
De novo telomere addition junction sequences.
- https://cdn.elifesciences.org/articles/58223/elife-58223-supp4-v2.docx
-
Supplementary file 5
Micro- and non-homology-mediated translocation junction sequences.
- https://cdn.elifesciences.org/articles/58223/elife-58223-supp5-v2.docx
-
Supplementary file 6
Interstitial deletion junction sequences.
- https://cdn.elifesciences.org/articles/58223/elife-58223-supp6-v2.docx
-
Supplementary file 7
Saccharomyces cerevisiae strains.
- https://cdn.elifesciences.org/articles/58223/elife-58223-supp7-v2.docx
-
Transparent reporting form
- https://cdn.elifesciences.org/articles/58223/elife-58223-transrepform-v2.docx