Functional partitioning of a liquid-like organelle during assembly of axonemal dyneins
Abstract
Ciliary motility is driven by axonemal dyneins that are assembled in the cytoplasm before deployment to cilia. Motile ciliopathy can result from defects in the dyneins themselves or from defects in factors required for their cytoplasmic pre-assembly. Recent work demonstrates that axonemal dyneins, their specific assembly factors, and broadly-acting chaperones are concentrated in liquid-like organelles in the cytoplasm called DynAPs (Dynein Axonemal Particles). Here, we use in vivo imaging in Xenopus to show that inner dynein arm (IDA) and outer dynein arm (ODA) subunits are partitioned into non-overlapping sub-regions within DynAPs. Using affinity- purification mass-spectrometry of in vivo interaction partners, we also identify novel partners for inner and outer dynein arms. Among these, we identify C16orf71/Daap1 as a novel axonemal dynein regulator. Daap1 interacts with ODA subunits, localizes specifically to the cytoplasm, is enriched in DynAPs, and is required for the deployment of ODAs to axonemes. Our work reveals a new complexity in the structure and function of a cell-type specific liquid-like organelle that is directly relevant to human genetic disease.
Introduction
Motile cilia are microtubule-based cellular projections and their oriented beating generates fluid flows that are critical for development and homeostasis. Ciliary beating is driven by a complex set of axoneme-specific dynein motors that drive sliding of the axonemal microtubule doublets. Based on their relative positions within the axoneme, these motors are classified as either outer dynein arms (ODAs) or inner dynein arms (IDAs)(Figure 1A, upper inset), the former driving ciliary beating generally, and the latter tuning the waveform (Kamiya and Yagi, 2014; King, 2018). Mutations in genes encoding ODA or IDA subunits are the major cause of the motile ciliopathy syndrome known as primary ciliary dyskinesia (PCD; MIM 244400). This genetic disease results in repeated sinopulmonary disease, bronchiectasis, cardiac defects, situs anomalies, and infertility (Horani et al., 2016; Mitchison and Valente, 2017; Wallmeier et al., 2020). Interestingly, PCD can also result from mutations in genes encoding any of several cytoplasmic proteins collectively known as Dynein Axonemal Assembly Factors (DNAAFs)(Desai et al., 2018; Fabczak and Osinka, 2019).
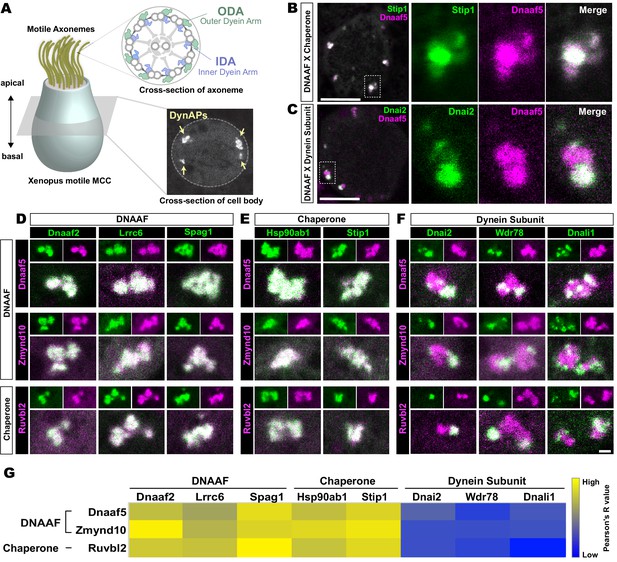
Dynein subunits occupy sub-regions within DynAPs.
(A) Schematic showing an MCC. The upper inset shows a schematic cross section through an axoneme indicating the relative positions of ODAs and IDAs; the lower inset shows a representative en face optical section through the MCC cytoplasm with dynAPs indicated DynAPs. (B) En face optical section through the cytoplasm of a Xenopus MCC expressing GFP-Stip1 (a chaperone, green) and mCherry-Dnaaf5 (a DNAAF, magenta). Note near-perfect co-localization. Dashed box indicates region shown in high magnification, split-channel views at right. Scale bars = 10 µm. (C) GFP-Dnai2 (a dynein subunit, green) co-localizes only partially with mCherry-Dnaaf5. (D, E) Images showing enlarged views of individual DynAPs after pairwise labeling with the indicated tagged DNAAFs or chaperones. Smaller panels show split channels, larger images show the merged channels. Note high degree of co-localization. (F) Similar pair-wise labeling with dynein subunits reveals only partial co-localization. Scale bar = 1 µm for panels D-F. (G) Heatmap showing Pearson correlation for colocalization of GFP fusion proteins with mCherry fusion proteins. Yellow indicates high value (Pearson’s R value = 0.85), while blue indicates low value (Pearson’s R value = 0.45). Exact Pearson’s R values for each protein combination can be found in Figure 1—figure supplement 1.
Axonemal dynein motors were known to be pre-assembled in the cytoplasm before deployment to cilia (Fowkes and Mitchell, 1998), but the first description of DNAAFs came later, with the identification of KTU (aka DNAAF2)(Omran et al., 2008). Studies of motile ciliopathy patients have now defined an array of cytoplasmic DNAAFs that are never part of the axoneme, yet when mutated result in loss of axonemal dyneins, and in turn, defective cilia beating (Diggle et al., 2014; Horani et al., 2012; Horani et al., 2013; Kott et al., 2012; Mitchison et al., 2012; Moore et al., 2013; UK10K Rare Group et al., 2017; Paff et al., 2017; UK10K et al., 2013; Zariwala et al., 2013). These specialized DNAAFs are now known to act in concert with ubiquitous, broadly-acting chaperones of the Heat Shock Protein (Hsp) Family (Cho et al., 2018; Li et al., 2017; Mali et al., 2018; UK10K Rare Group et al., 2017; Omran et al., 2008; Paff et al., 2017; UK10K et al., 2013; Yamaguchi et al., 2018; Yamamoto et al., 2010; Zhao et al., 2013; Zur Lage et al., 2018).
How DNAAFs organize the assembly of axonemal dyneins is a complex question, as each axoneme contains an series of closely related multiprotein complexes (King, 2018). For example, ODAs and IDAs incorporate diverse heavy, intermediate, and light chains encoded by distinct genes; few subunits are shared. Moreover, at least eight distinct IDA sub-types and two ODA sub-types have been described (Dougherty et al., 2016; Fliegauf et al., 2005; King, 2018). Generally, disruption of any single DNAAF has been found to impact both ODAs and IDAs (Fabczak and Osinka, 2019), but a recent study suggests that distinct DNAAFs may be dedicated for the assembly of specific subsets of dyneins (Yamaguchi et al., 2018). Another recent study has suggested that distinct DNAAFs may catalyze specific stages of the dynein assembly process (Mali et al., 2018). This complexity motivates another key question, this one regarding the spatial organization of axonemal dynein assembly.
Several studies indicated that the DNAAFs and chaperones act together in cytosolic foci (Diggle et al., 2014; Horani et al., 2012; Li et al., 2017; Mali et al., 2018), and we recently demonstrated that DNAAFs, chaperones, and axonemal dynein subunits are sequestered in specialized membrane-less organelles that we termed DynAPs (Huizar et al., 2018). DynAPs are multiciliated cell (MCC)-specific organelles that display hallmarks of biological phase separation, with DNAAFs and chaperones fluxing through rapidly while dynein subunits are stably retained (Huizar et al., 2018). Though DynAPs display properties similar to many ubiquitous liquid-like organelles (Banani et al., 2017; Shin and Brangwynne, 2017), little else is known of the cell biology underlying DynAP assembly or function.
We show here that DynAPs contain functionally distinct sub-compartments. Confocal imaging revealed that the organelles defined by enrichment of DNAAFs and/or chaperones are sub-divided into sub-compartments specifically enriched for ODA or IDA subunits. Affinity-purification and mass-spectrometry of IDA and ODA subunits from MCCs identified several novel interactors, including the uncharacterized protein Daap1/C16orf71. We show that Daap1 is a cytoplasmic protein that is enriched in DynAPs in both human and Xenopus MCCs. Moreover, Daap1 localization is restricted to the ODA sub-region of DynAPs, where assays of fluorescence recovery after photobleaching (FRAP) show it is stably retained. Finally, disruption of Daap1 elicits a severe loss of ODAs from motile cilia. These data provide new insights into the structure and function of a still poorly defined, cell-type specific, disease-associated organelle.
Results
Outer and inner arm dynein subunits occupy sub-regions within DynAPs
The Xenopus embryo epidermis is a mucociliary epithelium that serves as a highly tractable model for understanding motile cilia (Hayes et al., 2007; Walentek and Quigley, 2017; Werner and Mitchell, 2012). Using this system, we previously showed that while DNAAFs, chaperones, and dyneins all co-localize in DynAPs with the canonical DNAAF, Ktu/Dnaaf2, dynein subunits displayed relatively more variable co-localization (Huizar et al., 2018). Here, we explored these localization patterns in more detail by pairwise co-expression of several fluorescently tagged DNAAFs, Hsp chaperones, and axonemal dynein subunits. We examined their localization in en face optical sections through the cytoplasm of Xenopus MCCs in vivo using confocal microscopy (Figure 1A, lower inset).
We found, for example, that the Hsp co-chaperone Stip1/Hop displayed essentially total co-localization with the assembly factor Dnaaf5/Heatr2 (Figure 1B), while by contrast, the outer dynein arm subunit Dnai2 clearly displayed only partial overlap with Dnaaf5 (Figure 1C). In fact, pairwise tests of five distinct DNAAFs and three chaperones revealed consistent, strong co-localization in all combinations (Figure 1D,E,G). Conversely, pairwise tests with three different axonemal dynein subunits consistently revealed only partial co-localization (Figure 1F,G). In all cases, IDA or ODA subunits occupied distinct, well-demarcated sub-regions within DNAAF- or chaperone-labeled DynAPs (Figure 1F). Pearson correlations revealed that these differences were statistically significant (Figure 1—figure supplement 1).
We then performed pairwise tests of co-localization of several axonemal dynein subunits. Strikingly, we found that IDAs and ODAs were partitioned into mutually exclusive sub-regions. For example, the ODA subunit Dnai2 displayed near-perfect co-localization with two additional ODA sub-units, Dnai1 and Dnal4 (Figure 2A,B). By contrast, Dnai2 displayed very little colocalization with IDA-f subunit Wdr78 or the IDA-a, c, d subunit Dnali1 (Figure 2C,D; Figure 2—figure supplement 1A,B). Again, Pearson correlations revealed these differences to be highly significant (Figure 2—figure supplement 1C).
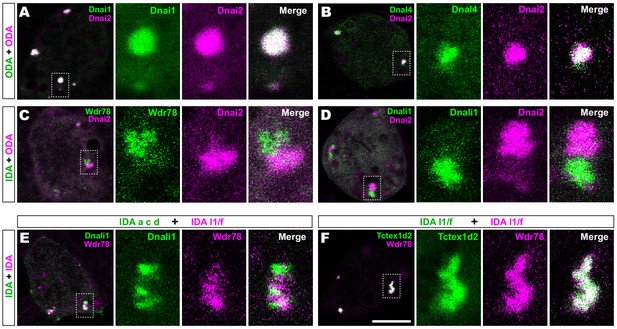
Outer and inner dynein arms localize to distinct sub-regions in DynAPs.
(A) En face optical section of a Xenopus MCC expressing ODA subunits GFP-Dnai1 and mCherry-Dnai2 (as per lower inset in Figure 1A). The dashed box indicates the area shown at higher magnification in the panels to the right. Note near complete co-localization. (B) mCherry-Dnai2 also displays near-total co-localization with another ODA subunit, Dnal4. (C, D) mCherry-Dnai2 shows very little co-localization with the IDA subunit GFP-Wdr78 or GFP-Dnali1. (E) IDA-f subunit mCherry-Wdr78 displays little co-localization with IDA-a, c, d subunit GFP-Dnali1. (F) IDA-f subunits, mCherry-Wdr78 and GFP-Tctex1d2, show complete co-localization. Scale bar = 10 µm.
Specific sub-classes of inner dynein arms are partitioned to distinct sub-compartments within DynAPs
We further observed that the different sub-classes of IDAs were also restricted to discrete sub-regions within DynAPs. For example, the IDA-a, c, d subunit Dnali1 and the IDA-f subunit Wdr78 displayed only very little co-localization (Figure 2E; Figure 2—figure supplement 1D). By contrast, Wdr78 displayed significantly stronger co-localized with another IDA-f subunit, Tctex1d2 (Figure 2F; Figure 2—figure supplement 1D).
Together, these data suggest that axonemal dynein subunits are partitioned into functionally distinct sub-regions; based upon their contents, with ODAs, and at least two sub-classes of IDAs occupying functionally-related restricted spaces within DynAPs. We will refer to these here as ‘ODA sub-DynAPs’ and ‘IDA-f sub-DynAPs,’ etc.
Affinity-purification and mass-spectrometry identifies specific in vivo interactors for vertebrate inner and outer dynein arm subunits in Xenopus MCCs
Given the essential role of cytoplasmic DNAAFs and chaperones in assembly of axonemal dyneins (Desai et al., 2018; Fabczak and Osinka, 2019), we reasoned that sub-DynAPs may also contain regulatory factors with specific roles in the assembly or deployment of either IDAs or ODAs. To test this idea, we sought to determine the interactomes of IDAs and ODAs directly in MCCs. We therefore developed a method to perform affinity-purification and mass-spectrometry (APMS) of in vivo interaction partners using Xenopus mucociliary epithelium and then examined the localization of interaction partners using in vivo imaging.
For this experiment, we took advantage of organotypically cultured explants of pluripotent ectoderm from early Xenopus embryos (so-called ‘animal caps’), which can be differentiated into a wide array of organs and tissues, including mucociliary epithelium (Ariizumi et al., 2009; Walentek and Quigley, 2017; Werner and Mitchell, 2012). We and others have used such explants previously for large-scale genomic studies of ciliogenesis and cilia function (Chung et al., 2014; Kim et al., 2018; Ma et al., 2014; Quigley and Kintner, 2017; Walentek et al., 2016).
As baits for APMS, we used GFP-fusions to either IDA or ODA subunits, expressing them specifically in MCCs using a well-defined MCC-specific α-tubulin promoter (Deblandre et al., 1999; Tu et al., 2018). For each experiment, we excised ~550 animal caps and cultured them until MCCs developed beating cilia (NF stage 23)(Figure 3A, green, blue arrows). Protein was isolated from these tissue explants and APMS was performed using an anti-GFP antibody (Figure 3A). To control for non-specific interactions, each APMS experiment was accompanied by a parallel APMS experiment (i.e. from explants cut from the same clutch of embryos) using un-fused GFP (Figure 3A, gray). The results of the latter experiment were then used to subtract background (Figure 3A, right; see Materials and methods), and we calculated a Z-test, fold-change, and a false discovery rate for differential enrichment of identified proteins (see Materials and methods).
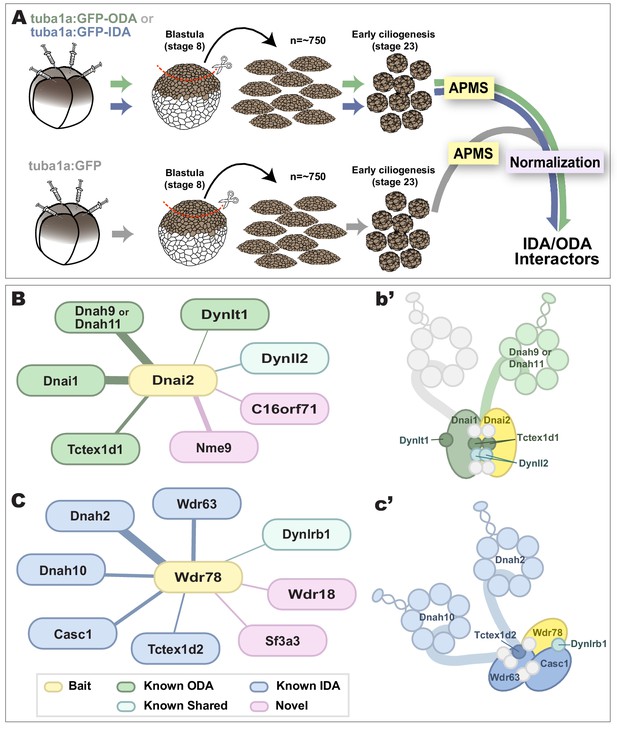
Specific identification of outer and inner arm dynein interactors.
(A) Schematic of APMS workflow for identifying in vivo ODA and IDA interactors. GFP-tagged ODA or IDA driven by MCC-specific alpha-tubulin promoter by plasmid injection into Xenopus embryos and animal caps were isolated at stage 8. The cultured explants were collected at early ciliogenesis stage (stage 23) and subjected to APMS. Unfused GFP was assessed simultaneously and the data subtracted to control for non-specific interactions. (B) Spoke diagram displaying Dnai2 (ODA) interactors, line weight of spokes indicates Log2 fold-change of PSMs. b’. Schematic of outer dynein arm indicating identified Dnai2 preys. (C) Spoke diagram displaying Wdr78 (IDA) interactors. c’. Schematic of inner dynein arm (f type) indicating identified Wdr78 preys.
To identify ODA interactors, we used GFP-Dnai2; for IDAs we used GFP-Wdr78 (King, 2018).
For both proteins, we found that the bait itself was the most strongly enriched hit in the APMS, providing an important positive control (Circled in Figure 3—figure supplement 1C, E; see Supplementary files 1, 2). Dnai2 pulldown strongly enriched for known ODA-specific sub-units, including the intermediate chain Dnai1, the heavy chains Dnah9/11, and the light chain Tctex1d1, among others (Figure 3B; Figure 3—figure supplement 1A–C; Supplementary file 1). Conversely, our Wdr78 sample was enriched for IDA components, specifically the IDA-f subtype, as expected (Figure 3C); these included the axonemal dynein heavy chains Dnah2 and Dnah10, as well as several light and intermediate chains (Figure 3C; Figure 3—figure supplement 1D–F; Supplementary file 2).
Finally, we identified weaker but significant interaction of Dnai2 and Wdr78 with a variety of known chaperones and assembly factors, including Ruvbl1/2, Spag1, and Dnaaf1 (Supplementary files 1, 2), consistent with findings that these proteins are enriched in DynAPs and are essential for axonemal dynein assembly (Fabczak and Osinka, 2019; Huizar et al., 2018). Our data provide new insights into the compositions of ODAs and f-type IDAs in Xenopus MCCs and also serve as positive controls for the specificity of our APMS approach in MCCs.
Identification and localization of novel IDA and ODA interactors
Importantly, our APMS identified not only known interactors, but also several novel interaction partners. For example, we observed interaction between Dnai2 and Nme9 (Figure 3B). Nme9 is highly similar to the Chlamydomonas ODA light chain LC3 and is present in the axonemes of airway cilia and sperm flagella in mice (Sadek et al., 2003). As in mice, Xenopus GFP-Nme9 localized to MCC axonemes (Figure 4A). More importantly, however, we also observed GFP-Nme9 in the cytoplasm, where it colocalized with Dnai2 specifically in ODA sub-DynAPs (Figure 4B,C).
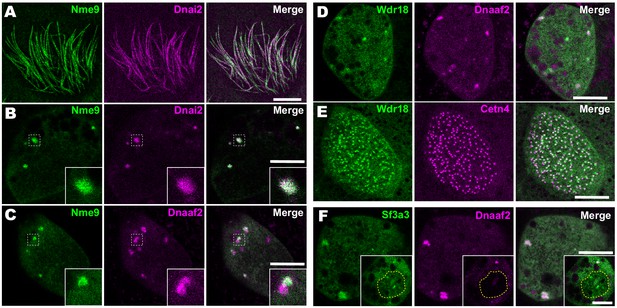
Localization of novel IDA and ODA interactors.
(A) En face optical section just above the apical surface of a Xenopus MCC showing axonemal localization of GFP-Nme9. (B) A similar optical section through the MCC cytoplasm shows near-perfect colocalization of GFP-Nme9 with the ODA subunit mCherry-Dnai2 in DynAPs. Dashed box indicates area shown at higher magnification in the inset. (C) GFP-Nme9 only partially co-localizes with mCherry-Dnaaf2. (D) En face optical section through the cytoplasm shows colocalization of GFP-Wdr18 with mCherry-Dnaaf2 in DynAPs. (E) A similar section just below the MCC apical surface reveals GFP-Wdr18 localization near basal bodies labeled with RFP-Centrin4 (F) GFP-Sf3a3 co-localizes with mCherry-Dnaaf2. Scale bars = 10 µm.
Another notable interaction was that between Wdr78 and Wdr18 (Figure 3C). This result is significant because Wdr18 has been implicated in the control of ciliary beating, but its mechanism of action is entirely unknown (Gao et al., 2011; Silversides et al., 2012). Interestingly, GFP-Wdr18 was not present in the axonemes of motile cilia (not shown) but did localize to DynAPs (Figure 4D). Interestingly, GFP-Wdr18 was also strongly enriched in the apical cytoplasm of MCCs, near the basal bodies (Figure 4E). Similarly, another IDA interactor, Sf3a3 (Figure 3C), was also absent from axonemes (not shown), but did localize in DynAPs (Figure 4F). This protein was previously implicated in splicing (Krämer et al., 2005), so as expected, we also observed GFP-Sf3a3 in nuclear foci in Xenopus MCCs (Figure 4—figure supplement 1). Together, these data demonstrate that our APMS approach in Xenopus effectively identified novel, biologically plausible IDA and ODA-interacting proteins in vivo.
C16orf71/Daap1 is a novel ODA-interacting protein with an interesting evolutionary trajectory
The most interesting interaction we identified in this study was that between Dnai2 and C16orf71 (Figure 3B). Though highly conserved among vertebrates, this protein has yet to be the subject of even a single published study. We first sought to confirm that C16orf71 is a bona fide ODA interactor using a reciprocal APMS experiment, with GFP-tagged Xenopus C16orf71 as the bait. This analysis revealed interaction of C16orf71 not only with Dnai2, but also other ODA subunits such as Dnai1, Dnah9 and Nme9, as well as Ruvbl1 (Figure 5A; Figure 3—figure supplement 1G–I, Supp. File 3). Based on these findings and data described below, we propose to rename this protein Daap1, for Dynein Axonemal-Associated Protein 1.
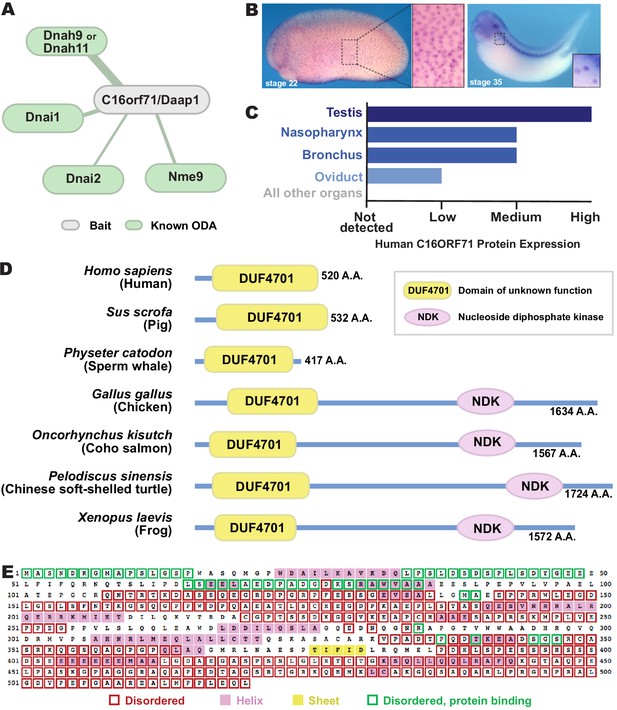
C16orf71/Daap1 is a novel ODA interactor specifically expressed in MCCs.
(A) Spoke diagram displaying C16orf71/Daap1 interactors; line weight indicates Log2 fold- change of PSMs. (B) In situ hybridization of Daap1 in Xenopus reveals expression in epidermal MCCs (left) and MCCs in the nephrostomes (right). (C) Graph showing C16orf71 protein expression levels in human tissues from the Human Protein Atlas (Uhlén et al., 2015). (D) Domain organization of C16orf71/Daap1 orthologs across vertebrates from NCBI Orthologs. (E) Domain prediction with human C16orf71/DAAP1 sequence from the PSIPRED Protein Analysis Workbench for disorder and secondary structure prediction.
In situ hybridization revealed that Xenopus Daap1 expression was enriched in MCCs in the epidermis and the nephrostomes of the pronephros (Figure 5B). Likewise, examination of data from the Human Protein Atlas (Uhlén et al., 2015) revealed that human DAAP1 protein is present exclusively in tissues with motile cilia (Figure 5C). Interestingly, while Daap1 is conserved among vertebrates, it appears to have followed an interesting evolutionary trajectory. In mammals, including humans, orthologs of Daap1 are composed entirely of a domain of unknown function (DUF4701), which phylogenetic analysis suggests is vertebrate-specific (Figure 5D). Our analysis of this sequence suggests it is intrinsically disordered (Figure 5E), a feature commonly associated with liquid-like organelles. By contrast, all non-mammalian vertebrate Daap1 orthologs contain not only the DUF4701 domain, but also a long C-terminal extension harboring, remarkably, an NDK domain similar to those in Nme8 and Nme9 (Figure 5D). We therefore chose this novel ODA interactor for more in-depth study.
Daap1 is a DynAP-specific protein in Xenopus and human MCCs
We next examined the localization of the Daap1 protein in Xenopus MCCs. Intriguingly, no signal was detectable in MCC axonemes as marked by Dnai2, and instead, GFP-Daap1 was localized exclusively to foci in the cytoplasm of MCCs (Figure 6A,B). GFP-Daap1 strongly co-localized with Dnai2 (Figure 6B), suggesting that the interaction we identified by APMS occurs in DynAPs. Indeed, Daap1 displayed a partial co-localization with Dnaaf2 similar to that observed in the cytoplasm for other ODA subunits (Figure 6C). Finally, Daap1 displayed little or no overlap with GFP-Wdr78 (Figure 6D), suggesting that it localized specifically in ODA sub-DynAPs (Figure 6D).
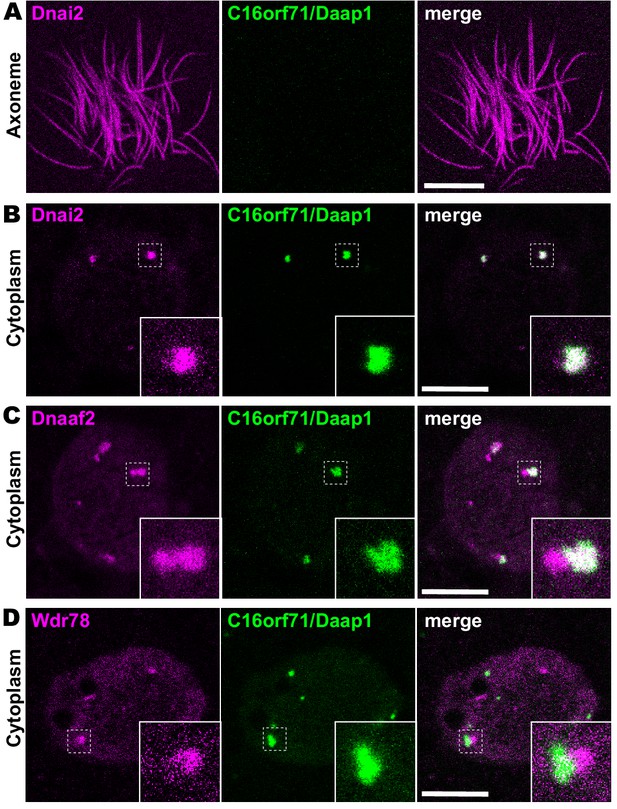
Daap1 is a DynAP-specific protein in Xenopus MCCs.
(A) En face optical section above the apical surface of a Xenopus MCC reveals absence of GFP-Daap1 in axonemes. (B) A similar optical section through the MCC cytoplasm shows near-perfect colocalization of GFP-Daap1 with the ODA subunit mCherry-Dnai2 in DynAPs. Inset shows higher magnification view of dashed box for each panel. (C) GFP-Daap1 only partially co-localizes with mCherry-Dnaaf2. (D) GFP-Daap1 co-localizes only very weakly with the IDA subunit mCherry-Wdr78. Scale bars = 10 µm.
Because human DAAP1 lacks the C-terminal NDK domain present in the Xenopus ortholog (Figure 5D), we were curious to know if the human ortholog also localizes to DynAPs. We therefore performed immunostaining for DAAP1 on sections of human lung. As observed in Xenopus, DAAP1 was not detectable in the axonemes of human MCCs (Figure 7A, brackets), and cytoplasmic staining for DAAP1 was strongly enriched in DynAPs, as indicated by co-labeling for the ODA subunit DNAI1 (Figure 7A, arrowheads).
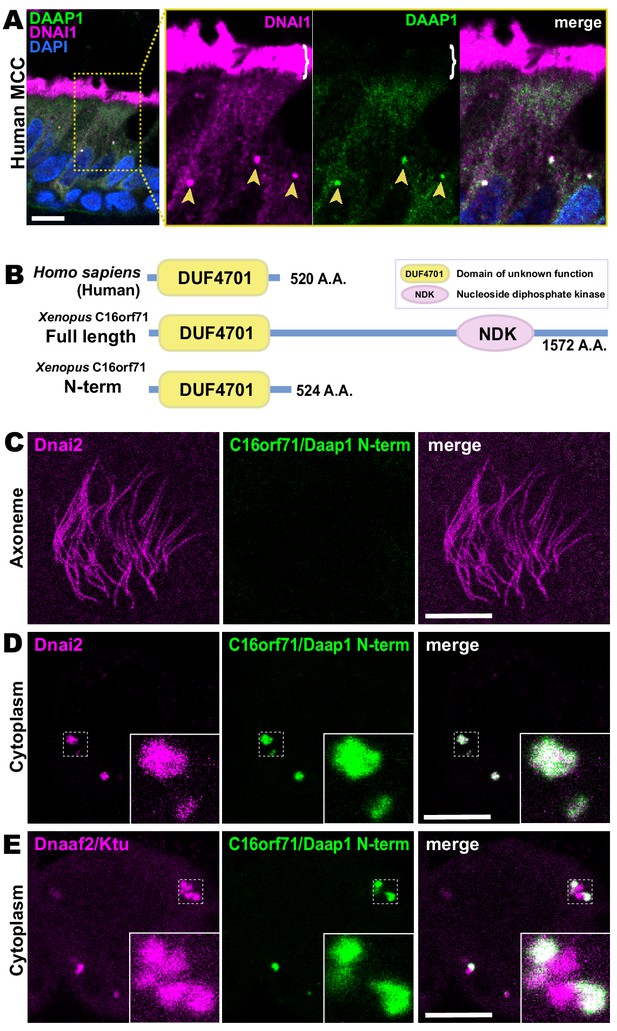
Human DAAP1 is a DynAP-specific protein.
(A) A primary human lung section immunostained for human DAAP1 (green) and the ODA subunit DNAI1 (magenta). Boxed area indicates magnified region shown at right. DNAI1 strongly labels MCC axonemes (bracket) and also DynAPs in the cytoplasm (arrowheads). As in Xenopus, human DAAP1 is not present in axonemes but is strongly enriched in DynAPs. (DAPI (blue) marks nuclei; scale bar = 10 µm) (B) Schematic of C16orf71 constructs: Xenopus Daap1-Nterm is truncated, containing only the DUF4701 domain, similar to human DAAP1. (C) En face optical section above the apical surface of a Xenopus MCC reveals absence of Daap1-Nterm-GFP in axonemes. (D, E) Similar optical sections through the cytoplasm shows near-perfect colocalization of GFP-Daap1-Nterm with the ODA subunit mCherry-Dnai2 and only partial co-localization with Dnaaf2/Ktu. In all cases, inset shows higher magnification view of dashed box for accompanying panels. Scale bars = 10 µm.
This result then prompted us to examine the localization of a deletion construct comprising the isolated N-terminal DUF4701 domain of Xenopus Daap1 (i.e. a Xenopus equivalent of human DAAP1)(Figure 7B). We found that GFP-Daap1-Nterm also localized to ODA sub-DynAPs in Xenopus MCCs (Figure 7C–E). Thus, despite their divergent evolutionary trajectories, both Xenopus and human DAAP1 are DynAP-specific cytoplasmic proteins, and the N-terminal DUF4701 domain is sufficient to direct localization to ODA sub-DynAPs.
Daap1 is stably retained in ODA sub-DynAPs
DynAPs are liquid-like organelles, and we previously found using FRAP that both the DNAAFs and broadly-acting chaperones flux rapidly through these organelles, while both ODA and IDA subunits are stably retained (Huizar et al., 2018). Because Daap1 does not localize to axonemes in either humans or Xenopus, its localization to DynAPs suggests a role in ODA processing or assembly, rather than a direct function in ciliary beating. We therefore expected Daap1 to flux rapidly through DynAPs, similar to other cytoplasmic assembly factors. To our surprise, however, FRAP revealed instead that Xenopus Daap1 was retained in DynAPs in a manner that was similar, but not identical, to dynein subunit Dnai2 (Figure 8). Specifically, Daap1 displayed a somewhat slower initial recovery, but rather than plateauing at around 20 s, the signal continued to slowly recover for at least 60 s (Figure 8B).
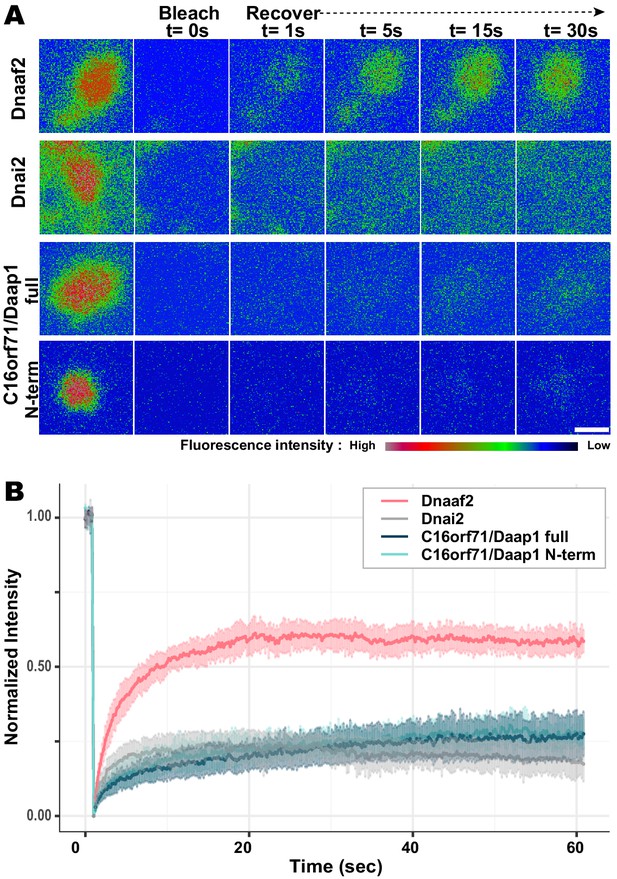
Daap1 is stably retained in DynAPs.
(A) Stills from time-lapse imaging of FRAP for the assembly factor GFP-Dnaaf2, the ODA subunit GFP-Dnai2, GFP-Daap1 and GFP-Daap1-Nterm. Images are color-coded to highlight changes in pixel intensity (see key below images). Scale bar = 1 µm (B) Graphs displaying FRAP curves for GFP-Dnaaf2 (n = 14), GFP-Dnai2 (n = 12) GFP-Daap1 (n = 26), and GFP-Daap1-Nterm (n = 22).
We were also interested to know if human Daap1 displays similar dynamics, but FRAP assays in human MCCs are very challenging. We therefore examined the FRAP kinetics of Xenopus Daap1-Nterm as a proxy of the human protein for use in Xenopus MCCs. In FRAP assays, Daap1-Nterm displayed essentially identical kinetics to full-length Daap1 (Figure 8B). This result is consistent with the localization data above and strongly suggests that the DUF4701 domain is sufficient to drive the retention of Daap1 in ODA sub-DynAPs.
Daap1 is required for deployment of ODAs to the axoneme
We tested this idea using morpholino (MO)-mediated knockdown (KD) to disrupt splicing of the Daap1 transcript in Xenopus (Figure 9—figure supplement 1A). Consistent with its interaction specifically with ciliary beating machinery, Daap1-KD had no impact on ciliogenesis itself, and well-defined ciliary tufts were observed in all morphants. However, Daap1-KD elicited severe loss of ODAs from MCC axonemes (Figure 9A,B, D, E). Both the intermediate chain Dnai2, as well as the light chain Dnal1 were severely reduced, with only small domains of the proximal axoneme labeled by either marker following knockdown (Figure 9A,B). We quantified this phenotype by examining intensity plots for Dnai2 and Dnai1 along the length of motile axonemes and normalizing this against co-expressed membrane-RFP (Figure 9C,F). Importantly, this phenotype was also observed using a second MO targeting a distinct splice site, suggesting that this phenotype is a specific effect of Daap1 depletion. (Figure 9—figure supplement 1B,C).
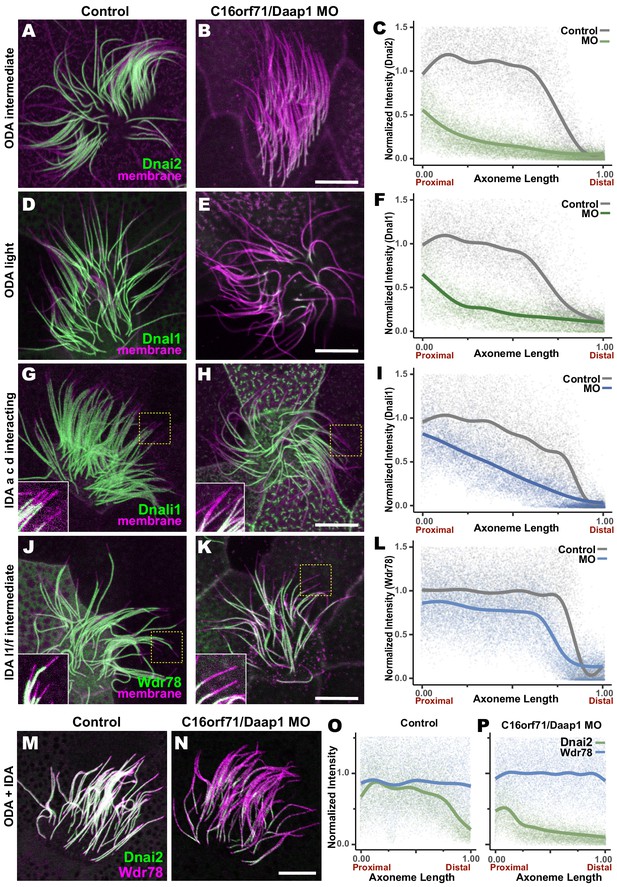
Loss of C16orf71 disrupts deployment of dynein subunits to the axoneme.
(A–B) Xenopus MCC axonemes labeled by membrane-RFP (magenta) together with the GFP-Dnai2, an ODA intermediated chain in control embryo (A) and in C16orf71/Daap1 morphant (B). (C) Graph showing intensity of GFP-Dnai2 along the normalized axoneme length (n = 29 for control, 51 for morphant). (D–E) MCCs labeled by membrane-RFP (magenta) together with the GFP-Dnal1, an ODA light chain in control embryo (D) and in C16orf71/Daap1 morphant (E). (F) Graph showing intensity of GFP-Dnal1 along the normalized axoneme length (n = 27 for control, 26 for morphant). (G–H) MCCs labeled by membrane RFP (magenta) together with the GFP-Dnali1, an IDA a, c, d interacting protein in control embryo (G) and in C16orf71/Daap1 morphant (H). (I) Graph showing intensity of GFP-Dnali1 along the normalized axoneme length (n = 30 for control, 33 for morphant). (J–K) MCCs labeled by membrane-RFP (magenta) together with the GFP-Wdr78, an IDA l1/f intermediated chain in control embryo (J) and in C16orf71/Daap1 morphant (K). (L) Graph showing intensity of GFP-Dnal1 along the normalized axoneme length (n = 32 for control, 43 for morphant). Both Dnai2, an ODA Intermediated chain and Dnal1, an ODA light chain are severely reduced in the axoneme in C16orf71 morphants, while IDA subunits display only mild loss from the distal most axoneme (inset). Yellow boxes indicate regions shown in accompanying insets for each panel. (M–N) MCCs co-expressing a marker for both ODAs (Dnai2, green) and IDAs (Wdr78, magenta). Loss of C16orf71 results in specific loss of ODAs in the axoneme (N), while both ODA and IDA properly localize in the axoneme in control (M). (O–P) Graphs showing intensities of GFP-Dnai2 and mCherry-Wdr78 along the normalized axoneme length in controls (O) and morphants (P). Scale bars = 10 µm.
Given the specific localization of Daap1 to ODA sub-DynAPs, we expected that Daap1 loss should specifically impact axonemal deployment of ODAs, and for the most part, this was the case. While both ODA proteins were depleted along the entire length of the axoneme, the IDA-a, c, d protein Dnali1 and the IDA-f protein Wdr78 displayed more subtle defects (Figure 9G,H,J,K). Dnali1 displayed a mild reduction along the entire axoneme (Figure 9I), while Wdr78 displayed an even mild disruption that was largely restricted to the distal axoneme (Figure 9L). Interestingly, this result may relate to the partial co-localization of Dnali1 with Dnai2 (Figure 2—figure supplement 1C) and reflects the known intimate link between assembly of ODAs and IDA-c (e.g. Yamaguchi et al., 2018; Yamamoto et al., 2020).
Overall, comparisons of subunit intensities demonstrated that loss of Daap1 had a significantly greater impact on ODA compared to IDAs. Because the effect on IDA-f was quite subtle (Figure 9L), we also made a more direct comparison by generating intensity plots to directly compare Wdr78 and Dnai1 co-expressed in the same axonemes (Figure 9M–P). This analysis clearly demonstrated a profoundly more substantial loss of ODAs than IDAs after Daap1 depletion.
Thus, Daap1 is a DynAP-specific protein that interacts with ODA subunits and is essential for the deployment of ODAs to axonemes and further suggests a complex interplay of ODAs and IDAs is required to establish the final patterning of motors that drive ciliary beating.
Discussion
Here, we have used live imaging to demonstrate that DynAPs contain sub-compartments that specifically partition inner and outer dynein arm subunits (Figures 1 and 2). Using in vivo proteomics, we identify several novel axonemal dynein interactors, including C16orf71/Daap1 (Figures 3 and 5). In both Xenopus and humans, Daap1 is present exclusively in the cytoplasm and not in ciliary axonemes. Moreover, the protein is highly enriched in DynAPs, where it is restricted to the ODA sub-DynAP (Figures 6–8). Remarkably, loss-of-function experiments revealed a requirement for Daap1 predominantly in the deployment of ODAs throughout the length of MCC cilia, while the impact on IDAs was only very mild, and restricted largely to the distal axoneme (Figure 9). Together, these data are significant for (1) adding weight to the argument that DynAPs serve a specific function in the assembly of axonemal dyneins, (2) demonstrating that sub-DynAPs represent molecularly and functionally distinct spaces within DynAPs, and (3) suggesting that additional DynAP-specific regulatory factors remain to be discovered.
Novel axonemal dynein interactors
In addition to the mechanistic insights provided by this study (discussed in detail below), our method for APMS and the datasets of in vivo IDA and ODA interactors provided here will be a useful resource for future studies. For example, wdr18 knockdown results in left/right asymmetry defects in zebrafish and mutations in human WDR18 are associated with congenital heart defects (Gao et al., 2011; Silversides et al., 2012); both phenotypes are indicative of ciliary beating defects, but the mechanism of Wdr18 action is entirely unknown. The data here are significant, then, for connecting Wdr18 to IDAs (Figure 3C) and for reporting the localization of Wdr18 in motile ciliated cells for the first time. Because we found that Wdr18 was not present in the axonemes of motile cilia but was present in DynAPs and near basal bodies (Figure 4D,E), our data suggest that Wdr18 function may not relate directly to IDA function, but rather to the assembly or transport of IDAs.
The more curious result here is the interaction of Wdr78 with Sf3a3 (Figure 3C), a well-defined component of the RNA splicing machinery (Krämer et al., 2005). Though unexpected, this result, and our finding that Sf3a3 is enriched in DynAPs (Figure 4F) is consistent with our recent report that DynAPs contain both RNA and RNA-associated proteins (Drew et al., 2020), similar to other liquid-like organelles such as stress granules and P bodies (Banani et al., 2017; Lin et al., 2015; Mittag and Parker, 2018). Indeed, we found that Cfap43 and Cfap44, which tether IDA-f to microtubules (Fu et al., 2018; Kubo et al., 2018), are both RNA-associated and localized to sub-DynAPs in Xenopus MCCs (Drew et al., 2020).
These findings are especially interesting in light of two additional recent papers. First, a recent paper reports that splicing factor Srsf1 plays a splicing-independent role in ciliary beating, likely via control of translation of protein essential for ciliary beating (Haward et al., 2020). A second recent paper reports the presence of ‘KL granules’ in Drosophila sperm that -like DynAPs- contain the Dynein assembly chaperones Ruvbl1/2, and these granules are also highly enriched in the mRNAs encoding axonemal dyneins (Fingerhut and Yamashita, 2020). Taken together, these findings suggest that the role of RNA-associated proteins in DynAP assembly or function should be a rich area for future investigation.
Daap1 and the Nme family of proteins and ciliary beating
Our data here also shed light on the evolution of motile cilia function and regulation in vertebrates, particularly with respect to Daap1 and the Nme family of NDK proteins (Desvignes et al., 2009). Three Nme proteins have been implicated in motile cilia function, including Nme8, which is orthologous to the LC3 ODA subunit in Chlamydomonas and has been implicated in human motile ciliopathy (Duriez et al., 2007; Pazour et al., 2006). Nme5 is also required for ciliary beating in Xenopus and mammals, though its mechanism of action remains unknown (Anderegg et al., 2019; Chung et al., 2014; Vogel et al., 2012). Nme9 (aka Txndc6 or Txl-2) is localized to motile cilia in the mouse (Sadek et al., 2003), but its function is also unknown. It is significant, then, that we found Nme9 to be localized to Xenopus MCC cilia (Figure 4A–C). The observed interactions with Dnai2 and Daap1 (Figure 3C, Figure 5A) suggest that it too may be an ODA subunit.
Curiously, examination of genome data using Xenbase (Karimi et al., 2018; Session et al., 2016) suggests that Xenopus lacks the Nme8 gene, and further exploration in NCBI revealed that many vertebrate genomes contain only Nme8 or Nme9, but not both (not shown). The linkage of an NDK domain to the DUF4701 domain in non-mammalian Daap1 orthologs suggest the possibility that these two protein domains may act in concert, and it is tempting to speculate that the NDK domain of Daap1 in non-mammalian vertebrates may explain the absence of either Nme8 or Nme9 in those genomes. Interestingly, a very recent report describes the discovery of novel NDK domain protein in Tetrahymena with a specific role in stabilizing ODA dyneins in an inactive conformation for targeting to axonemes (Mali et al., 2020). It is possible then that Daap1 plays a similar role in Xenopus. Continued studies in diverse organisms will thus be of great interest.
The composition and function of DynAPs
DynAPs have now been observed in human, mouse, Xenopus and zebrafish, so it is clear that these organelles represent a conserved element of MCCs (Diggle et al., 2014; Horani et al., 2018; Huizar et al., 2018; Li et al., 2017; Mali et al., 2018). However, the precise function of DynAPs remains unknown, a situation shared with many liquid-like organelles. Genetic evidence suggests that DynAPs are factories for axonemal dynein assembly, as genetic loss of DynAP-localized DNAAFs consistently results in a failure of dynein arm assembly, failure of dynein deployment to axonemes, and defective ciliary beating (Fabczak and Osinka, 2019). Our identification of Daap1 as yet another protein that is enriched in DynAPs and is specifically required for deployment of axonemal dyneins to cilia adds weight to the argument that DynAPs perform a critical assembly or deployment function.
Strictly speaking, however, all DNAAF proteins and chaperones yet studied are present throughout the cytoplasm and enriched in DynAPs, so we cannot rule out the possibility that dyneins are normally assembled in the cytoplasm, while DynAPs serve some other function. For example, DynAPs may act in storage or assembly of dyneins for rapid deployment to cilia (e.g. for ciliary regeneration) or for quality control and/or degradation of mis-folded dyneins. Answering such questions is a key challenge, and is one faced generally for liquid-like organelles.
Regardless, our data clearly demonstrate that DynAPs are structurally partitioned, as are many other liquid-like organelles (e.g. Feric et al., 2016; Jain et al., 2016; Schmidt and Rohatgi, 2016). Moreover, our observation that Daap1 is restricted to ODA sub-DynAPs (Figures 6 and 7) and that its loss results predominantly in ODA defects (Figure 9) argues that sub-DynAPs reflect a functional sub-compartmentalization. Conversely, though it has been suggested that DNAAFs and chaperones act in a relay system to catalyze iterative steps of ODA and IDA assembly (Mali et al., 2018), we found no evidence of physical compartmentalization of any of the tested DNAAFs and chaperones (Figure 1), suggesting that partitioned localization of those factors is not strictly required. Consistent with this idea, though Ktu has been shown to specifically impact assembly of ODAs and IDA-c (Yamaguchi et al., 2018), we found no restriction of Ktu localization within DynAPs (Figure 1 and see Huizar et al., 2018). Together, our findings here provide a deeper cell biological framework in which to understand the complex genetics of dynein assembly in motile ciliated cells.
Materials and methods
Xenopus embryo manipulations
Request a detailed protocolXenopus embryo manipulations were carried out using standard protocols. Briefly, female adult Xenopus were induced to ovulate by injection of hCG (human chorionic gonadotropin). In vitro fertilization was carried out by homogenizing a small fraction of a testis in 1X Marc’s Modified Ringer’s (MMR). Embryos were dejellied in 1/3x MMR with 2.5%(w/v) cysteine (pH7.8). Embryos were microinjected with mRNA, circular DNA or morpholinos in 2% Ficoll (w/v) in 1/3x MMR and injected embryos were washed with 1/3x MMR after 2 hr.
Plasmids and MOs for microinjections
Request a detailed protocolXenopus gene sequences were obtained from Xenbase (http://www.xenbase.org) and open reading frames (ORF) of genes were amplified from the Xenopus cDNA library by polymerase chain reaction (PCR), and then are inserted into a pCS10R vector containing a fluorescence tag. In addition to the vectors described previously (Huizar et al., 2018), the following constructs were cloned into pCS vector: ruvbl2-mCherry, mCherry-zmynd10, GFP-wdr78, GFP-dnai1, GFP-dnal4, GFP-dnal1, GFP-nme9, GFP-wdr18, GFP-sf3a3, GFP-c16orf71, GFP-c16orf71 N-term (1-527aa), GPF-tctex1d2 and mCherry-wdr78. These constructs were linearized and the capped mRNAs were synthesized using mMESSAGE mMACHINE SP6 transcription kit (ThermoFisher Scientific). Each 80 pg of each mRNA was injected into two ventral blastomeres. For APMS, GFP-dnai2, GFP-wdr78 and GFP-c16orf71 N-term were inserted into pCS2 vectors under multiciliated-cell-specific alpha-tubulin promoter and each 40 pg of each DNA was injected into blastomeres. C16orf71/Daap1 morpholinos were designed to target 1st or 3rd exon-intron splicing junction, The MO sequences and the working concentrations include:
MO #1: 5’-AGTAAGGTCTGTACACTTACCAGGG-3’, 30 ng per injection
MO #2: 5’-ACAAATGCAAGTTTTTCTTACCTCA-3’, 10 ng per injection
Immunoprecipitation of Xenopus animal caps for mass-spectrometry
Request a detailed protocolTo identify Dnai2 or Wdr78 interactors, circular plasmids of GFP only, GFP-dnai2 and GFP-wdr78 driven by MCC-specific α-tubulin promoter were injected into 4-blastomeres of 4 cell stage Xenopus embryos. Approximately 550 animal caps per sample were isolated at stage eight using forceps and were cultured in 1X Steinberg’s solution (0.58 mM NaCl, 0.64 mM KCl, 0.33 mM Ca(NO2)2, 0.8 mM MgSO4, 5 mM Tris, 50 µg/ml gentamicin, pH 7.4–7.6) until sibling embryos reached stage 23. The cultured explants were collected and immunoprecipitation (IP) was performed using GFP-Trap Agarose Kit (ChromoTek, cat# gtak-20). Immunoprecipitated proteins were eluted in 2X sample buffer. For MS of C16orf71, GFP only and GFP-c16orf71 plasmid driven by alpha-tubulin promoter were injected into Xenopus embryos and ~500 animal caps were collected from each sample. The following steps were performed with the same procedures as described for Dnai2 and Wdr78 AP. For statistical tests of enrichment, GFP-wdr78 and GFP-dnai2 share a GFP- only control (GFP_1a_08292018, GFP_1b_08292018) and GFP-c16orf71 uses a separate GFP-only control (GFP_1a_04252019, GFP_1b_ 04252019).
Affinity-purification-mass-spectrometry
Request a detailed protocolImmunoprecipitated proteins were resuspended in SDS-PAGE sample buffer and heated 5 min at 95°C before loading onto a 7.5% acrylamide mini-Protean TGX gel (BioRad). After 7 min of electrophoresis at 100 V the gel was stained with Imperial Protein stain (Thermo) according to manufacturer’s instructions. The protein band was excised, diced to 1 mm cubes and processed by standard trypsin in-gel digest methods for mass-spectrometry (for experiments with baits Dnai2 and Wdr78) or by the more rapid method of Goodman et al., 2018 for C16orf71. Digested peptides were desalted with HyperSep Spin Tip C-18 columns (Thermo Scientific), dried, and resuspended in 30–60 µl of 5% acetonitrile, 0.1% acetic acid for mass-spectrometry.
Samples from experiments with baits Dnai2 and Wdr78 were analyzed on a Thermo Orbitrap Fusion mass spectrometer and those with bait C16orf71 were analyzed using a Thermo Orbitrap Fusion Lumos Tribrid mass spectrometer. In all cases peptides were separated using reverse phase chromatography on a Dionex Ultimate 3000 RSLCnano UHPLC system (Thermo Scientific) with a C18 trap to Acclaim C18 PepMap RSLC column (Dionex; Thermo Scientific) configuration and eluted using a 3% to 45% gradient over 60 min. with direct injection into the mass spectrometer using nano-electrospray. Data were collected using a data-dependent top speed HCD acquisition method with full precursor ion scans (MS1) collected at 120,000 m/z resolution. Monoisotopic precursor selection and charge-state screening were enabled using Advanced Peak Determination (APD), with ions of charge ≥ + two selected for high energy-induced dissociation (HCD) with stepped collision energy of 30% +/- 3% (Lumos) or 31% +/- 4% (Orbitrap Fusion). Dynamic exclusion was active for ions selected once with an exclusion period of 20 s (Lumos) or 30 s (Orbitrap Fusion). All MS2 scans were centroid and collected in rapid mode.
Raw MS/MS spectra were processed using Proteome Discoverer (v2.3). We used the Percolator node in Proteome Discoverer to assign unique peptide spectral matches (PSMs) at FDR < 5% to the composite form of the X. laevis reference proteome described in Drew et al., 2020, which comprises both genome-derived Xenbase JGI v9.1 + GenBank X. laevis protein sets, but with homeologs and highly related entries combined into EggNOG vertebrate-level orthology groups (Huerta-Cepas et al., 2016), based on the method developed in McWhite et al., 2020. In order to identify proteins statistically significantly associated with each bait, we calculated both a log2 fold-change and a Z-score for each protein based on the observed PSMs in the bait (‘expt’) versus control (‘ctrl’) pulldown. The fold-change was computed for each protein as:
where n is the total number of proteins considered in the experiment.
For visualization purposes and initial rankings, we calculated significance for protein enrichment in the experiment relative to control using a one-sided Z-test as in Lu et al., 2007 with a 95% confidence threshold (z ≥ 1.645), as:
e for each protein based on the where and .
We determined significance by calculating p-values for each Z-score using the pnorm distribution function available in the R Stats Package (v3.6.1). We corrected for multiple comparisons by computing the Benjamini-Hochberg false discovery rate using the p.adjust function, also from the R Stats Package (v3.6.1). Probability values and false discovery rates are provided in Supplementary files 1–3.
Imaging, FRAP and image analysis
Request a detailed protocolXenopus embryos were mounted between cover glass and submerged in 1/3x MMR at stage 22 or 23, and then were imaged immediately. Live images were captured with a Zeiss LSM700 laser scanning confocal microscope using a plan-apochromat 63 × 1.4 NA oil objective lens (Zeiss) or with Nikon eclipse Ti confocal microscope with a 63×/1.4 oil immersion objective. For FRAP experiments, a region of interest (ROI) was defined as a 1.75 µm X 1.75 µm box. ROIs were bleached using 50% laser power of a 488 nm laser and a 0.64 µsec pixel dwell time. Fluorescence recovery was recorded at ~ 0.20 s intervals for up to 300 frames. Bleach correction and FRAP curve-fitting was carried out using a custom python script (modified from http://imagej.net/Analyze_FRAP_movies_with_a_Jython_script). For colocalization analysis, z- stack images were captured from at least 15 cells from at least five different embryos. Z stacks were split to single sections and ROI for DynAPs was defined in red channel (mCherry) of each section using ImageJ selection tool. The analysis was carried out using Fiji coloc2 plugin.
Intensity of dynein subunits and length of axonemes were measured using Fiji. Plots were generated using PRISM eight and the ggplot2 package in R. One-way ANOVA and Tukey’s Honest Significant Difference (HSD) test and Welch Two Sample t-test were performed in R.
Protein domain prediction
Request a detailed protocolThe human DAAP1 sequence was analyzed using the PSIPRED Protein Analysis Workbench for disorder and secondary structure prediction (Buchan and Jones, 2019).
Airway epithelial cell culture and immunostaining
Request a detailed protocolHuman trachea were isolated from surgical excess of tracheobronchial segments of lungs donated for transplantation. These unidentified tissues are exempt from regulation by HHS regulation 45 CFR Part 46. Paraffin embedded tracheal sections were fixed and immunostained as previously described (Pan et al., 2007; You et al., 2002). Nuclei were stained using 4’, 6-diamidino-2-phenylindole (DAPI, Vector Laboratories, Burlingame, CA, USA). The ODA protein, DNAI1, was detected using primary antibodies obtained from NeuroMab (UC Davis, Ca, clone UNC 65.56.18.11). Antibodies to DAAP1 (C16orf71) were obtained from Sigma-Millipore (St. Louis, MO, HPA049468). Images were acquired using a Zeiss LSM700 laser scanning confocal microscope.
In situ hybridization
Request a detailed protocolIn situ hybridization was performed as described previously (Sive et al., 2000) using DIG-labeled single-stranded RNA probes against a partial sequence (1–1572) of Xenopus c16orf71 ORF. Bright field images were captured with a Zeiss Axio Zoom. V16 stereo microscope with Carl Zeiss Axiocam HRc color microscope camera.
Rt-pcr
Request a detailed protocolTo verify the efficiency of C16orf71 MOs, MOs were injected into all cells at the 4 cell stage and total RNA was isolated using the TRIZOL reagent (Invitrogen) at stage 23. cDNA was synthesized using M-MLV Reverse Transcriptase (Invitrogen) and random hexamers. C16orf71 cDNAs were amplified by Taq polymerase (NEB) with these primers: c16orf71 5F 5’-cttcagagcaggacggattt-3’, c16orf71 582R 5’-ggcagaggtgcttagatgtt-3’, c16orf71 600F 5’- gggcttgtcattgcagtttc-3’, c16orf71 1254R 5’-tctctaccgtccttctcttctc-3’. odc1 primers were odc1 426F 5’-ggcaaggaatcacccgaatg-3’ and odc1 843R 5’-ggcaacatagtatctcccaggctc-3’.
Data deposition
Request a detailed protocolProteomics data has been deposited into Massive which in turn was passed to ProteomeXchange.
The Massive accession # is: MSV000085075 The direct link is https://massive.ucsd.edu/ProteoSAFe/dataset.jsp?task=f6a5e10c6e114b36b8c895664860db7e.
The ProteomeXchange # is PXD017980 as noted in the paper. The direct link is http://proteomecentral.proteomexchange.org/cgi/GetDataset?ID=PXD017980.
The direct link to the data ftp site is ftp://massive.ucsd.edu/MSV000085075/.
Data availability
Proteomics data has been deposited into Massive which in turn was passed to ProteomeXchange. The Massive accession # is: MSV000085075 The ProteomeXchange # is PXD017980 as noted in the paper. The direct link is http://proteomecentral.proteomexchange.org/cgi/GetDataset?ID=PXD017980 The direct link to the data ftp site is ftp://massive.ucsd.edu/MSV000085075/. These data are also provided in Supp. Tables 1-3.
-
MassIVEID 10.25345/C5T69F. Functional partitioning of a liquid-like organelle during assembly of axonemal dyneins.
-
ProteomeXchangeID PXD017980. Functional partitioning of a liquid-like organelle during assembly of axonemal dyneins.
References
-
Isolation and Differentiation of Xenopus Animal Cap CellsCurrent Protocols in Stem Cell Biology 9:sc01d05s9.https://doi.org/10.1002/9780470151808.sc01d05s9
-
Biomolecular condensates: organizers of cellular biochemistryNature Reviews Molecular Cell Biology 18:285–298.https://doi.org/10.1038/nrm.2017.7
-
The PSIPRED protein analysis workbench: 20 years onNucleic Acids Research 47:W402–W407.https://doi.org/10.1093/nar/gkz297
-
A two-step mechanism generates the spacing pattern of the ciliated cells in the skin of Xenopus embryosDevelopment 126:4715–4728.
-
BookCytoplasmic preassembly and trafficking of axonemal dyneinsIn: King S. M, editors. Dyneins: Structure, Biology, and Disease. Academic Press. pp. 140–161.https://doi.org/10.1016/B978-0-12-809471-6.00004-8
-
Nme protein family evolutionary history, a vertebrate perspectiveBMC Evolutionary Biology 9:256.https://doi.org/10.1186/1471-2148-9-256
-
DNAH11 localization in the proximal region of respiratory cilia defines distinct outer dynein arm complexesAmerican Journal of Respiratory Cell and Molecular Biology 55:213–224.https://doi.org/10.1165/rcmb.2015-0353OC
-
Role of the novel Hsp90 Co-Chaperones in dynein arms’ PreassemblyInternational Journal of Molecular Sciences 20:6174.https://doi.org/10.3390/ijms20246174
-
Mislocalization of DNAH5 and DNAH9 in respiratory cells from patients with primary ciliary dyskinesiaAmerican Journal of Respiratory and Critical Care Medicine 171:1343–1349.https://doi.org/10.1164/rccm.200411-1583OC
-
The role of preassembled cytoplasmic complexes in assembly of flagellar dynein subunitsMolecular Biology of the Cell 9:2337–2347.https://doi.org/10.1091/mbc.9.9.2337
-
The I1 dynein-associated tether and tether head complex is a conserved regulator of ciliary motilityMolecular Biology of the Cell 29:1048–1059.https://doi.org/10.1091/mbc.E18-02-0142
-
Whole-exome capture and sequencing identifies HEATR2 mutation as a cause of primary ciliary dyskinesiaThe American Journal of Human Genetics 91:685–693.https://doi.org/10.1016/j.ajhg.2012.08.022
-
Genetics and biology of primary ciliary dyskinesiaPaediatric Respiratory Reviews 18:18–24.https://doi.org/10.1016/j.prrv.2015.09.001
-
Xenbase: a genomic, epigenomic and transcriptomic model organism databaseNucleic Acids Research 46:D861–D868.https://doi.org/10.1093/nar/gkx936
-
BookComposition and assembly of axonemal dyneinsIn: King S. M, editors. Dyneins: Structure, Biology, and Disease. Elsevier. pp. 162–201.
-
Loss-of-function mutations in LRRC6, a gene essential for proper axonemal assembly of inner and outer dynein arms, cause primary ciliary dyskinesiaThe American Journal of Human Genetics 91:958–964.https://doi.org/10.1016/j.ajhg.2012.10.003
-
Structure-function analysis of the U2 snRNP-associated splicing factor SF3aBiochemical Society Transactions 33:439–442.https://doi.org/10.1042/BST0330439
-
A microtubule-dynein tethering complex regulates the axonemal inner dynein f (I1)Molecular Biology of the Cell 29:1060–1074.https://doi.org/10.1091/mbc.E17-11-0689
-
Multicilin drives centriole biogenesis via E2f proteinsGenes & Development 28:1461–1471.https://doi.org/10.1101/gad.243832.114
-
Motile and non-motile cilia in human pathology: from function to phenotypesThe Journal of Pathology 241:294–309.https://doi.org/10.1002/path.4843
-
Multiple modes of Protein-Protein interactions promote RNP granule assemblyJournal of Molecular Biology 430:4636–4649.https://doi.org/10.1016/j.jmb.2018.08.005
-
Mutations in ZMYND10, a gene essential for proper axonemal assembly of inner and outer dynein arms in humans and flies, cause primary ciliary dyskinesiaThe American Journal of Human Genetics 93:346–356.https://doi.org/10.1016/j.ajhg.2013.07.009
-
Mutations in PIH1D3 cause X-Linked primary ciliary dyskinesia with outer and inner dynein arm defectsThe American Journal of Human Genetics 100:160–168.https://doi.org/10.1016/j.ajhg.2016.11.019
-
RhoA-mediated apical actin enrichment is required for ciliogenesis and promoted by Foxj1Journal of Cell Science 120:1868–1876.https://doi.org/10.1242/jcs.005306
-
Identification of predicted human outer dynein arm genes: candidates for primary ciliary dyskinesia genesJournal of Medical Genetics 43:62–73.https://doi.org/10.1136/jmg.2005.033001
-
BookEarly Development of Xenopus laevis: A Laboratory ManualCold Spring Harbor Press.
-
Protein localization screening in vivo reveals novel regulators of multiciliated cell development and functionJournal of Cell Science 131:jcs206565.https://doi.org/10.1242/jcs.206565
-
DYX1C1 is required for axonemal dynein assembly and ciliary motilityNature Genetics 45:995–1003.https://doi.org/10.1038/ng.2707
-
Congenital hydrocephalus in genetically engineered miceVeterinary Pathology 49:166–181.https://doi.org/10.1177/0300985811415708
-
Growth and differentiation of mouse tracheal epithelial cells: selection of a proliferative populationAmerican Journal of Physiology-Lung Cellular and Molecular Physiology 283:L1315–L1321.https://doi.org/10.1152/ajplung.00169.2002
-
ZMYND10 is mutated in primary ciliary dyskinesia and interacts with LRRC6The American Journal of Human Genetics 93:336–345.https://doi.org/10.1016/j.ajhg.2013.06.007
-
Ciliary dynein motor preassembly is regulated by Wdr92 in association with HSP90 co-chaperone, R2TPJournal of Cell Biology 217:2583–2598.https://doi.org/10.1083/jcb.201709026
Article and author information
Author details
Funding
NIH (HD085901)
- John B Wallingford
NIH (HL117164)
- John B Wallingford
NIH (R01 DK110520)
- Edward M Marcotte
NIH (R35 GM122480)
- Edward M Marcotte
NIH (R01 HL128370)
- Steven L Brody
NIH (R01 HL146601)
- Steven L Brody
NIH (K08HL150223)
- Amjad Horani
NIH (K99 HD092613)
- Kevin Drew
NIH (LRP)
- Kevin Drew
Welch Foundation (F-1515)
- Edward M Marcotte
CPRIT (RP110782)
- Edward M Marcotte
Army Research Office (W911NF-12-1-0390)
- Edward M Marcotte
The funders had no role in study design, data collection and interpretation, or the decision to submit the work for publication.
Acknowledgements
We thank Claire McWhite and Anna Battenhouse for consulting and advice on X. laevis orthogroup calculations. This work was supported by grants from the NIH R01 HL117164 and R01 HD085901 to JBW; R01 DK110520, R35 GM122480 to EMM; R01 HL128370 and R01 HL146601 to SLB; NIH K08HL150223 to AH and K99 HD092613 and LRP to KD, as well as the Welch Foundation (F‐1515) to EMM. Mass spectrometry data collection was supported by CPRIT grant RP110782 to Maria Person and by Army Research Office grant W911NF-12-1-0390.
Ethics
Animal experimentation: All experiments were performed in strict accordance with the UT IACU protocol # AUP-2018-00225.
Copyright
© 2020, Lee et al.
This article is distributed under the terms of the Creative Commons Attribution License, which permits unrestricted use and redistribution provided that the original author and source are credited.
Metrics
-
- 2,108
- views
-
- 305
- downloads
-
- 40
- citations
Views, downloads and citations are aggregated across all versions of this paper published by eLife.
Citations by DOI
-
- 40
- citations for umbrella DOI https://doi.org/10.7554/eLife.58662