Neurovascular coupling and bilateral connectivity during NREM and REM sleep
Figures
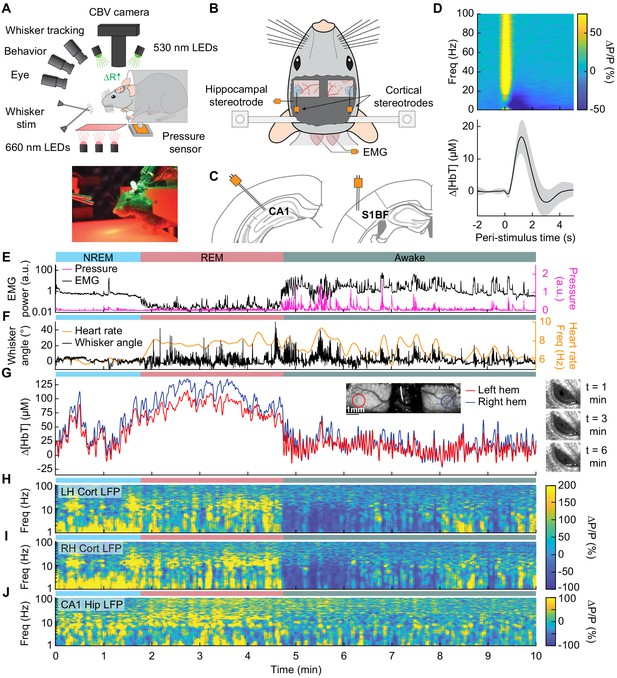
Sleep drives large changes in cerebral blood volume.
(A) Schematic of IOS experimental setup. The brain is illuminated with 530 nm LEDs, and changes in reflected light captured by a CCD camera mounted above the head. Other cameras track the whiskers (illuminated by 660 nm LEDs beneath the animal), the eye (illuminated by 780 nm LEDs), and changes in animal behavior. A piezo sensor to record changes in body motion is located beneath the animal, which rests head-fixed in a cylindrical tube. Tubes direct air to the distal part of the whiskers (but not the face), and do not interfere with volitional whisking. (B) Schematic showing the locations of the bilateral thinned-skull windows and recording electrodes. Each electrode consists of two Teflon-coated tungsten wires (~100 µm tip spacing), while the EMG electrode consists of two stainless-steel wires with several mm of insulation stripped off each end, inserted into adjacent nuchal muscles. (C) Left: Diagram showing hippocampal CA1 recording site. Right: Diagram of somatosensory cortex recording site. Adapted from Figure (52) (left) and Figure (42) (right) of The Mouse Brain in Stereotactic Coordinates, 3rd Edition (Franklin and Paxinos, 2007). (D) Average neural and hemodynamic responses to contralateral whisker stimulation (n = 14 mice, 28 hemispheres, 110 ± 70 stimulations per animal). Top: average normalized change in LFP power (∆P/P) in the somatosensory cortex in response to contralateral whisker stimulation. Bottom: mean change in total hemoglobin (∆[HbT]) within the ROI. Shaded regions indicate ± 1 standard deviation. (E-J) Example showing the hemodynamic and neural changes accompanying transitions among the NREM, REM and awake states. (E) Plot of nuchal muscle EMG power and body motion via a pressure sensor located beneath the mouse. (F) Plot of the whisker position and heart rate (G) Changes in total hemoglobin ∆[HbT] within the ROIs. Inset shows images of the two windows and respective ROIs. (H,I) Normalized left and right vibrissae cortex LFP power (∆P/P). (J) Normalized CA1 LFP power.
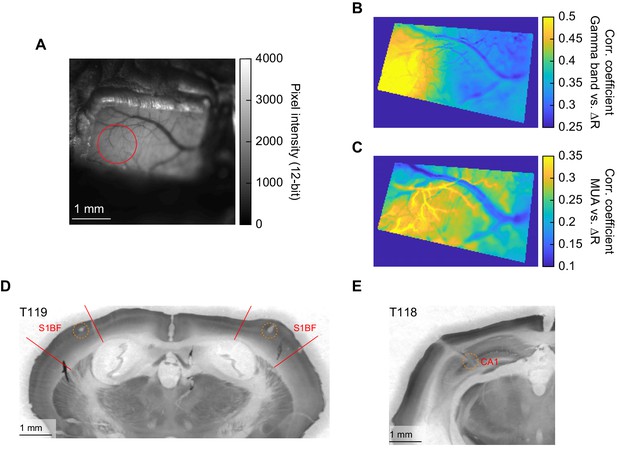
Localization of electrodes and hemodynamic regions of interest.
(A) Image of a cortical window showing cortical vasculature. (B) Peak pixel-wise cross-correlation (1–2 s lag) between gamma band power [30–100 Hz] and pixel reflectance during the first 60 min of data. (C) Same as (B) For multi-unit activity (300–3000 Hz). The peak cross-correlation was used to localize a 1 mm diameter region of interest (ROI). (D) Histological example of a coronal section stained with cytochrome oxidase (CO). Small holes in the slice indicate the location of cortical stereotrodes in the vibrissa barrel cortex. Adapted from Figure (39) of The Mouse Brain in Stereotactic Coordinates, 3rd Edition (Franklin and Paxinos, 2007). (E) Histological example of a coronal section stained with CO. Electrode path is visible and terminates in the CA1 region of hippocampus. Adapted from Figure (52) of The Mouse Brain in Stereotactic Coordinates, 3rd Edition (Franklin and Paxinos, 2007).
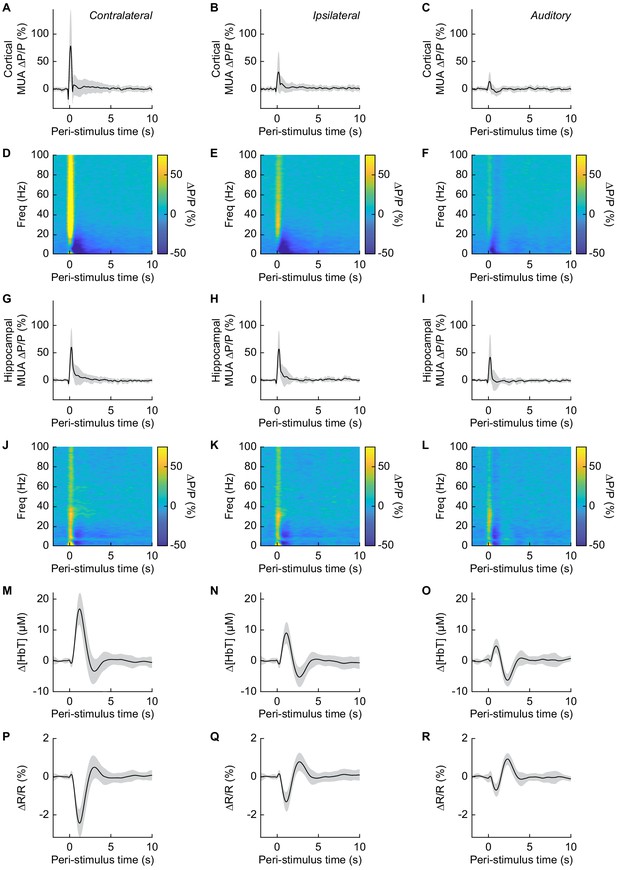
Whisker stimulation causes increases in neural activity and blood volume.
Comparisons between contralateral, ipsilateral, and auditory whisker stimulation and the corresponding changes in vibrissa cortical MUA/LFP, hippocampal MUA/LFP, and hemodynamic changes (reflectance/[HbT]) (n = 14 mice, 28 hemispheres). (A–C) Contralateral whisker stimulation caused large increases in vibrissa cortical MUA power (78.1 ± 66.7%) in comparison to ipsilateral (30.8 ± 37.5%) and auditory (13.7 ± 16.8%) stimulation. (D–F) LFP gamma band power increased (110.4 ± 96.7%) in comparison to ipsilateral (36.8 ± 32.8%) and auditory stimulation (17.4 ± 15.7%). (G–I) All three forms of stimulation caused relatively similar changes in hippocampal MUA power (contralateral: 60.1 ± 34.3%, ipsilateral: 57 ± 33.4%, auditory: 42 ± 41.8%) and in (J–R) LFP gamma band power (contralateral: 32.5 ± 17.2%, ipsilateral: 28 ± 14.3%, auditory: 18.6 ± 8.1%). Contralateral whisker stimulation caused increases in total hemoglobin ∆[HbT] (16.8 ± 5.1 µM corresponding to a −2.4 ± 0.7% reflectance) larger than those from ipsilateral (9 ± 3.5 µM, −1.3 ± 0.5%) and auditory (4.8 ± 2.3 µM, −0.7 ± 0.3%) stimulation.
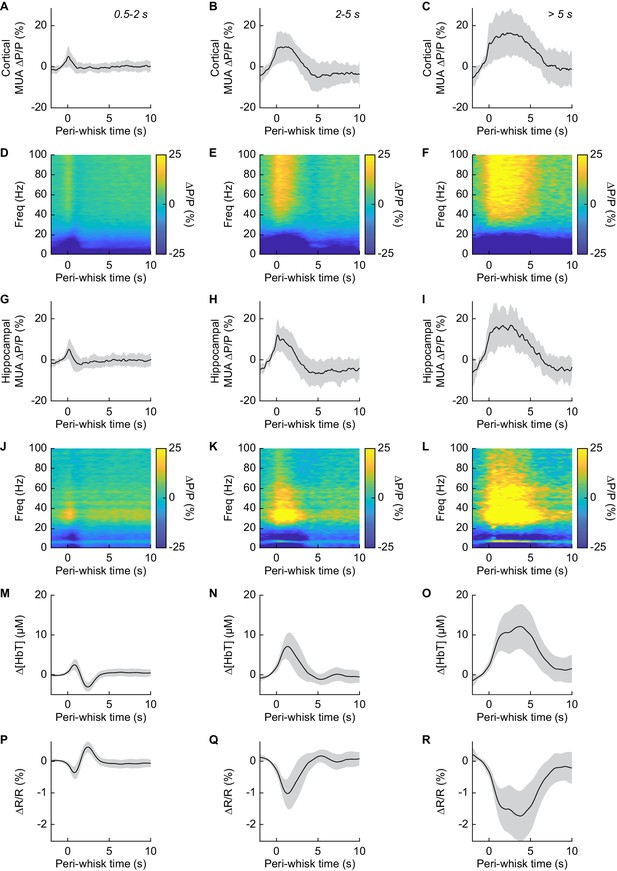
Volitional whisking causes increases in neural activity and hemodynamics.
Changes in vibrissa cortical MUA/LFP, hippocampal MUA/LFP, and blood volume during whisking events of various durations (0.5–2 s, 2–5 s, > 5 s) (n = 14 mice, 28 hemispheres). (A–C) Extended whisking caused larger increases in vibrissa cortical MUA power (16.5 ± 10.8%) in comparison to moderate (9.7 ± 7%) and brief (5 ± 4.8%) durations. (D–F) LFP gamma band power was higher during extended whisking (19.2 ± 18.9%) in comparison to moderate (15.1 ± 16.3%) and brief (6.7 ± 9.2%) durations. (G–I) Whisking drives increases in hippocampal MUA power (brief: 5.1 ± 5%, moderate: 12 ± 7.7%, extended: 16.9 ± 10.1%) and in (J–R) LFP gamma band power (brief: 5.7 ± 11.7%, moderate: 13.9 ± 14.3%, extended: 18.4 ± 14.9%). Extended whisking caused increases in total hemoglobin ∆[HbT] (12.1 ± 5.6 µM corresponding to a −1.7 ± 0.8% reflectance) that were larger than those seen in moderate (7.1 ± 3.3 µM, −1 ± 0.5%) and brief (2.5 ± 1.4 µM, −0.4 ± 0.2%) duration whisking events.
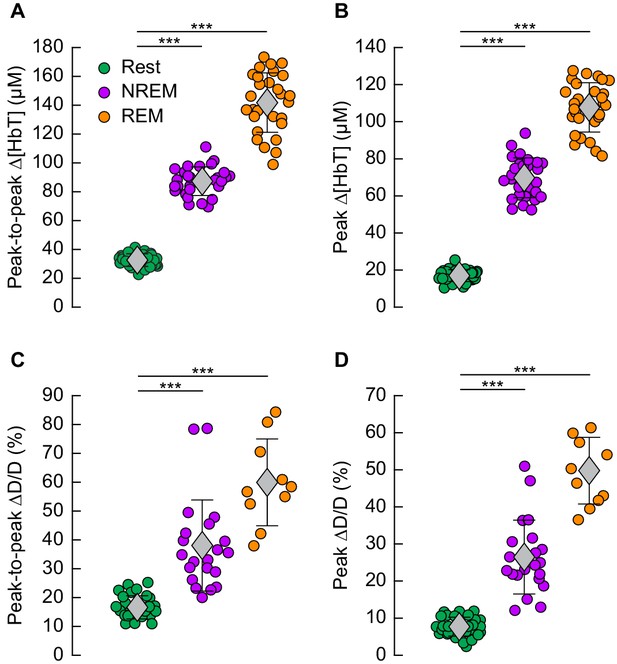
Amplitude of hemodynamic oscillations is largest during NREM and REM sleep.
(A) The peak-to-peak amplitude of ∆[HbT] oscillations during awake rest (32.3 ± 4.4 µM) were significantly smaller than those during contiguous NREM (87.3 ± 9.9 µM, GLME, p<9 × 10−32) and contiguous REM (142.1 ± 20.7 µM, GLME, p<1.5 × 10−53) sleep. (B) Mean peak ∆[HbT] of individual awake resting events (17 ± 3.2 µM) were significantly smaller than the peaks during contiguous NREM (69.8 ± 10.7 µM, GLME, p<1.1 × 10−37) and contiguous REM (107.7 ± 13.3 µM, GLME, p<3.5 × 10−55) sleep (n = 14 mice, 28 hemispheres). (C) Peak-to-peak amplitude of ∆D/D oscillations during awake rest (16.6 ± 4 µM) were significantly smaller by those during contiguous NREM (38 ± 15.8%, GLME, p<3.6 × 10−10) and contiguous REM (59.9 ± 15 µM, GLME, p<3.3 × 10−16) sleep. (D) Mean peak ∆D/D of individual awake resting events (7.4 ± 2.6 µM) were significantly smaller than the peaks during contiguous NREM (26.4 ± 10.1 µM, GLME, p<1.9 × 10−14) and contiguous REM (49.9 ± 9.1 µM, GLME, p<2 × 10−24) sleep (awake rest: n = 6 mice, 29 arterioles, contiguous NREM: n = 6 mice, 21 arterioles, contiguous REM: n = 5 mice, 10 arterioles). *p<0.05, **p<0.01, ***p<0.001 GLME.
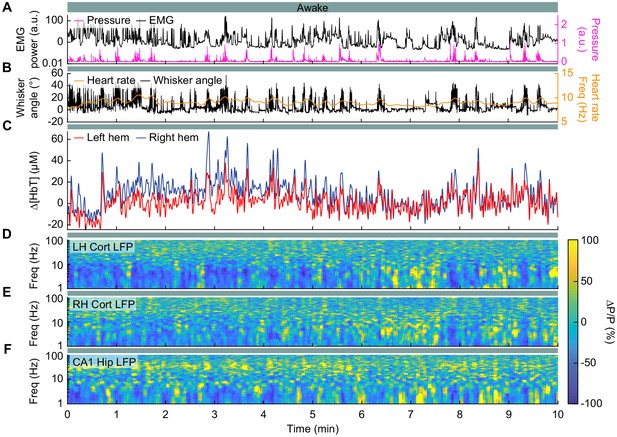
Sleep drives hemodynamic fluctuations larger than awake behaviors.
Examples showing the hemodynamic and neural changes accompanying transitions among the NREM, REM and awake states. (A) Plot of nuchal muscle EMG power and body motion via a pressure sensor located beneath the mouse. (B) Plot of the whisker position and heart rate. (C) Changes in total hemoglobin ∆[HbT] within the ROIs over the putative vibrissa cortex. (D) Normalized left vibrissae cortex LFP power (∆P/P). (E), Normalized right vibrissae cortex LFP power. (F) Normalized CA1 LFP power.
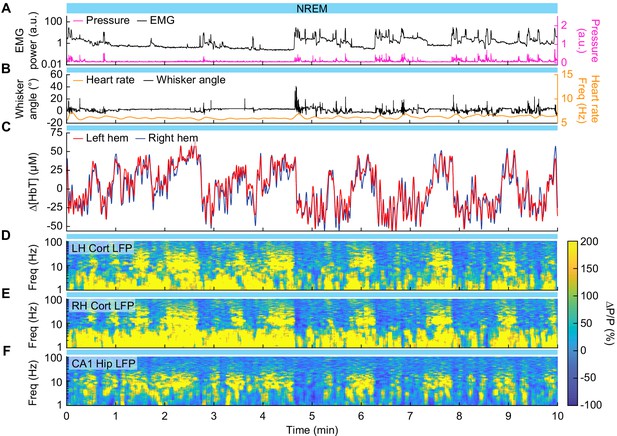
Sleep drives hemodynamic fluctuations larger than awake behaviors.
Examples showing the hemodynamic and neural changes accompanying transitions among the NREM, REM and awake states. (A) Plot of nuchal muscle EMG power and body motion via a pressure sensor located beneath the mouse. (B) Plot of the whisker position and heart rate. (C) Changes in total hemoglobin ∆[HbT] within the ROIs over the putative vibrissa cortex. (D) Normalized left vibrissae cortex LFP power (∆P/P). (E) Normalized right vibrissae cortex LFP power. (F) Normalized CA1 LFP power.
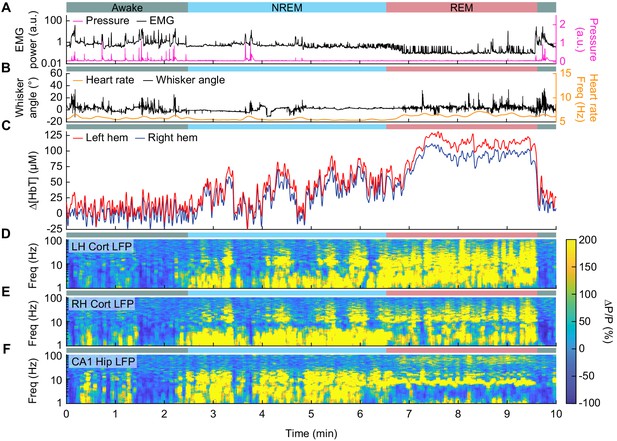
Sleep drives hemodynamic fluctuations larger than awake behaviors.
Examples showing the hemodynamic and neural changes accompanying transitions among the NREM, REM and awake states. (A) Plot of nuchal muscle EMG power and body motion via a pressure sensor located beneath the mouse. (B) Plot of the whisker position and heart rate. (C) Changes in total hemoglobin ∆[HbT] within the ROIs over the putative vibrissa cortex. (D), Normalized left vibrissae cortex LFP power (∆P/P). (E), Normalized right vibrissae cortex LFP power. (F), Normalized CA1 LFP power.
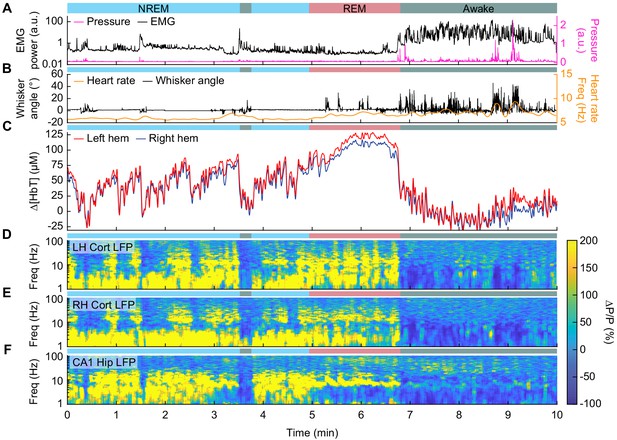
Sleep drives hemodynamic fluctuations larger than awake behaviors.
Examples showing the hemodynamic and neural changes accompanying transitions among the NREM, REM and awake states. (A) Plot of nuchal muscle EMG power and body motion via a pressure sensor located beneath the mouse. (B) Plot of the whisker position and heart rate. (C), Changes in total hemoglobin ∆[HbT] within the ROIs over the putative vibrissa cortex. (D) Normalized left vibrissae cortex LFP power (∆P/P). (E) Normalized right vibrissae cortex LFP power. (F) Normalized CA1 LFP power.
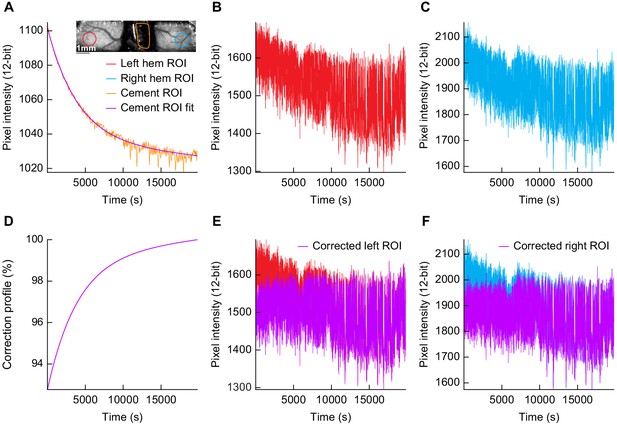
Correction of slow drifts in reflectance during IOS imaging.
(A) Image of bilateral hemispheres during IOS imaging with localized left, right ROIs as well as a region over the central cement that is used to correct a slow exponential drift in the camera’s sensitivity. The drift in reflectance of the cement (orange) is fit with an exponential (purple) and is used to correct the drifts in the lateral ROIs. (B) Raw pixel reflectance from the left hemisphere ROI. The exponential drift is clearly visible prior to correction. (C) Raw pixel reflectance from the right hemisphere ROI. (D) The exponential drift from the cement ROI is inverted and normalized to correct pixel reflectance in each hemisphere. (E) Original (red) vs. corrected (purple) pixel reflectance for the left hemisphere ROI. (F) Original (blue) vs. corrected (purple) pixel reflectance for the right hemisphere ROI.
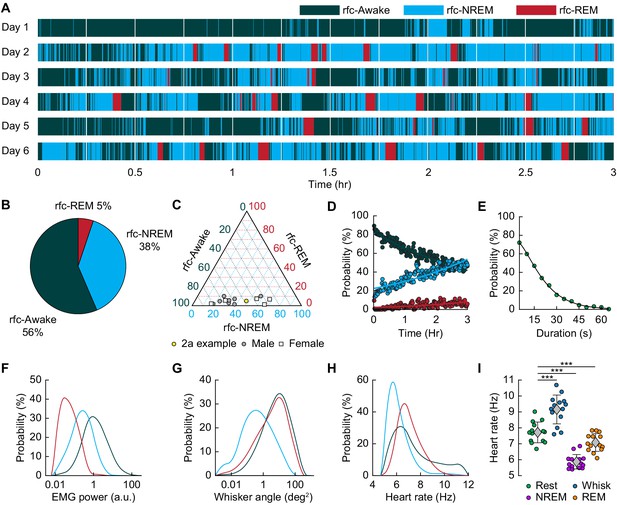
Mice rapidly and repeatedly transition between wake and sleep during head-fixation.
(A) Hypnogram showing the arousal states for a single mouse over six days. The hypnogram has a resolution of 5 s. White denotes breaks in data acquisition for saving of data. Note the rapid and frequent transitions between rfc-Awake, rfc-NREM and rfc-REM. (B-I) n = 14 mice. (B) Average percentage of the time spent in each arousal state. (C) Ternary plot showing each individual animal’s percentage in each arousal state. (D) Average probability of an animal being classified in a given arousal state as a function of time since the start of the session. Mice are progressively more likely to sleep and to be in REM sleep the longer they have been head-fixed E, Average probability of the animal being awake as a function of the duration of the period without movement. Mice are more likely to be asleep the longer they go without moving their whiskers or body. (F) Probability distribution of the mean EMG power during individual arousal states (5 s resolution) taken from all animals. (G) Probability distribution of variance in the whisker angle during individual arousal states (5 s resolution) taken from all animals. (H) Probability distribution of the mean heart rate for each arousal state. (I) Mean heart rate during different arousal states. Circles represent individual mice and diamonds represent population averages ± 1 standard deviation. *p<0.05, **p<0.01, ***p<0.001 GLME.
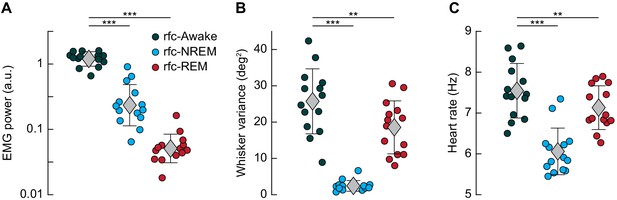
Behavioral measurements demarcate transitions between arousal states.
(A) Power in rfc-Awake electromyograph (EMG) recordings (1.3 ± 1.3 from the nuchal muscles were substantially smaller during rfc-NREM sleep (0.25 ± 2), GLME, p<3.2 × 10−12) and during rfc-REM sleep (0.05 ± 1.6, GLME, p<1.7 × 10−21) in comparison to the rfc-Awake state. (B) Variance in the whisker angle during rfc-NREM (2.4 ± 1.5 deg2, GLME, p<1.3 × 10−12) was significantly less than that of the awake state (25.7 ± 9 deg2). Whisker angle variance during rfc-REM (18.6 ± 7.3 deg2, GLME, p<0.003), though statistically different, was much more similar to the awake state due to mice sporadically moving their whiskers during rfc-REM sleep, analogous to rapid-eye movement seen in humans. (C) Heart rate during the rfc-Awake state was 7.5 ± 0.7 Hz. During rfc-NREM sleep, the heart rate dropped to 6.1 ± 0.6 Hz (GLME, p<1.8 × 10−13), and was elevated slightly during rfc-REM to 7.1 ± 0.5 Hz (GLME, p<0.004) (n = 14 mice). *p<0.05, **p<0.01, ***p<0.001 GLME.
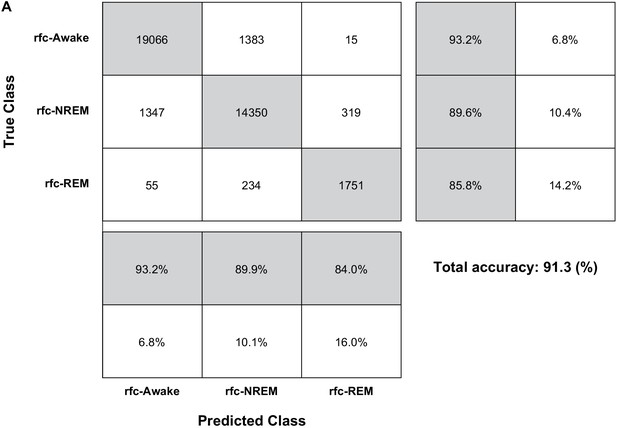
Random forest model validation.
All data from the first and last day of imaging from each animal was manually scored as rfc-Awake, rfc-NREM, or rfc-REM. Alternating 15 min periods of data from these two days were divided into two discrete sets: one for model training, the other is held back for model validation beyond the out-of-bag error obtained from the training data set. A confusion matrix containing each IOS animal’s (n = 14) random forest model predictions of the held back, second data set compared to its manual scores are presented in (A). The total model accuracy across all 14 animals was 91.3%, with the most accurate predictions coming from the most prevalent classification class (rfc-Awake), followed by rfc-NREM and then rfc-REM.
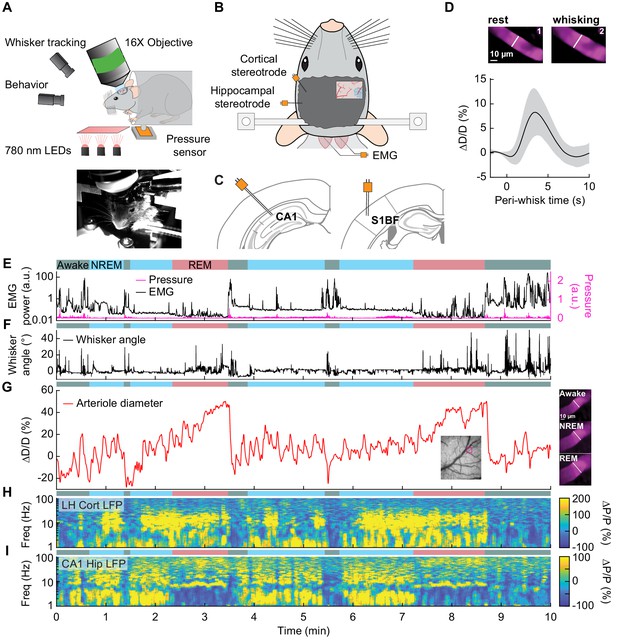
Sleep drives arteriole dilatations larger than those seen in the awake brain.
(A) Schematic of two-photon experimental setup. (B) Schematic of thinned-skull window and electrode recording sites. (C) Left: Diagram showing hippocampal CA1 recording site. Right: Diagram of vibrissae cortex recording site. Adapted from Figure (52) (left) and Figure (42) (right) of The Mouse Brain in Stereotactic Coordinates, 3rd Edition (Franklin and Paxinos, 2007). (D) Average response to awake volitional whisking (n = 6 mice, 29 arterioles). Top: Example showing a single arteriole’s diameter during rest and during a brief whisking event. Bottom: average change in arteriole diameter ∆D/D (%) during brief (2–5 s long) whisking events. Shaded regions indicate ±1 standard deviation. (E-I) Example showing the vascular and neural changes accompanying transitions among the NREM, REM and awake states. (E) Nuchal muscle activity through normalized EMG and body motion via a pressure sensor located beneath the mouse. (F) Whisker position. (G) Changes in arteriole diameter ∆D/D (%). (H) Normalized vibrissae cortical LFP. (I) Normalized CA1 LFP power.
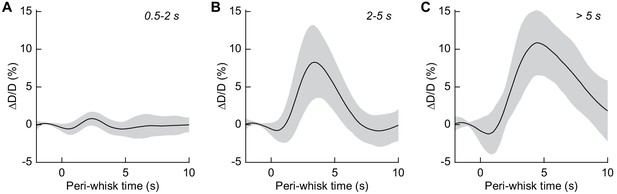
| Volitional whisking causes arteriole dilation.
(A) Brief awake whisking events (0.5–2 s) spurred a small dilation 0.8 ± 0.9%. (B) Moderate length awake whisking events (2–5 s) lead to a more robust dilation (8.3 ± 4.9%). (C) Extended awake whisking events (>5 s) produced the largest dilations (10.9 ± 4.3%, n = 29 arterioles).
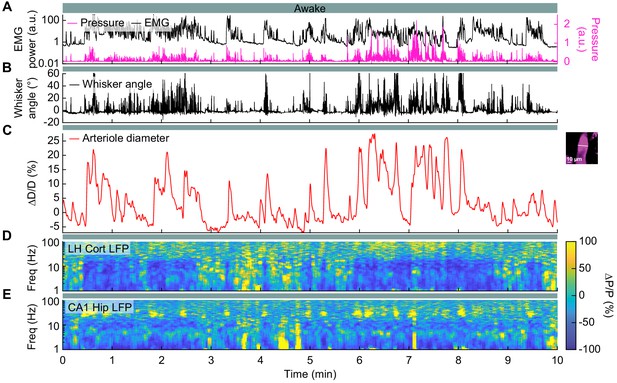
Arteriole dilatations during sleep are larger than those during the awake state.
Example showing the vascular and neural changes accompanying transitions among the NREM, REM, and awake states. Arterial diameters were imaged using two-photon microscopy. (A) Nuchal muscle activity through normalized EMG and body motion via a pressure sensor located beneath the mouse. (B) Whisker position. (C) Changes in arteriole diameter ∆D/D (%) in the putative vibrissa cortex. (D) Normalized LFP power from the left hemisphere stereotrode located in the left vibrissa cortex. (E) Normalized LFP power from the stereotrode in left CA1.
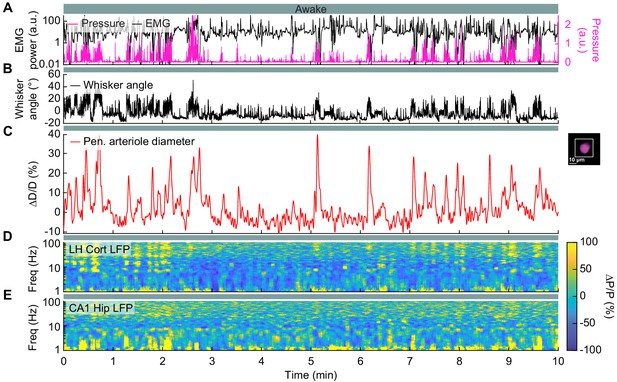
Arteriole dilatations during sleep are larger than those during the awake state.
Example showing the vascular and neural changes accompanying transitions among the NREM, REM, and awake states. Arterial diameters were imaged using two-photon microscopy. (A) Nuchal muscle activity through normalized EMG and body motion via a pressure sensor located beneath the mouse. (B) Whisker position. (C) Changes in arteriole diameter ∆D/D (%) in the putative vibrissa cortex. (D) Normalized LFP power from the left hemisphere stereotrode located in the left vibrissa cortex. (E) Normalized LFP power from the stereotrode in left CA1.
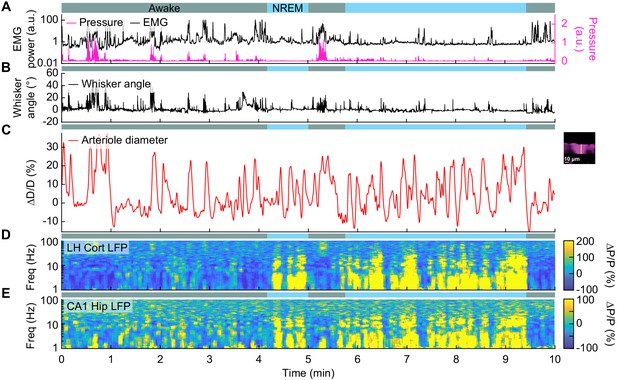
Arteriole dilatations during sleep are larger than those during the awake state.
Example showing the vascular and neural changes accompanying transitions among the NREM, REM, and awake states. Arterial diameters were imaged using two-photon microscopy. (A) Nuchal muscle activity through normalized EMG and body motion via a pressure sensor located beneath the mouse. (B) Whisker position. (C) Changes in arteriole diameter ∆D/D (%) in the putative vibrissa cortex. (D) Normalized LFP power from the left hemisphere stereotrode located in the left vibrissa cortex. (E) Normalized LFP power from the stereotrode in left CA1.
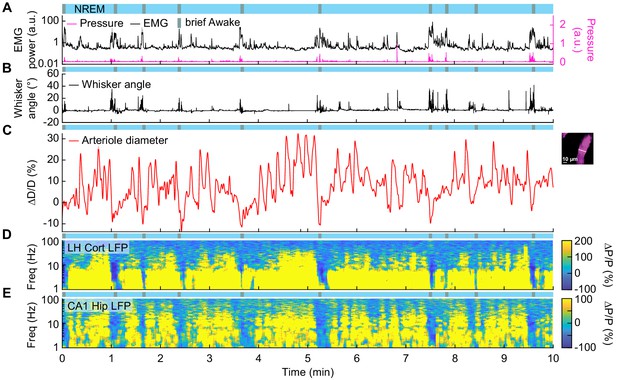
Arteriole dilatations during sleep are larger than those during the awake state.
Example showing the vascular and neural changes accompanying transitions among the NREM, REM and awake states. Arterial diameters were imaged using two-photon microscopy. (A) Nuchal muscle activity through normalized EMG and body motion via a pressure sensor located beneath the mouse. (B) Whisker position. (C) Changes in arteriole diameter ∆D/D (%) in the putative vibrissa cortex. (D) Normalized LFP power from the left hemisphere stereotrode located in the left vibrissa cortex. (E) Normalized LFP power from the stereotrode in left CA1.
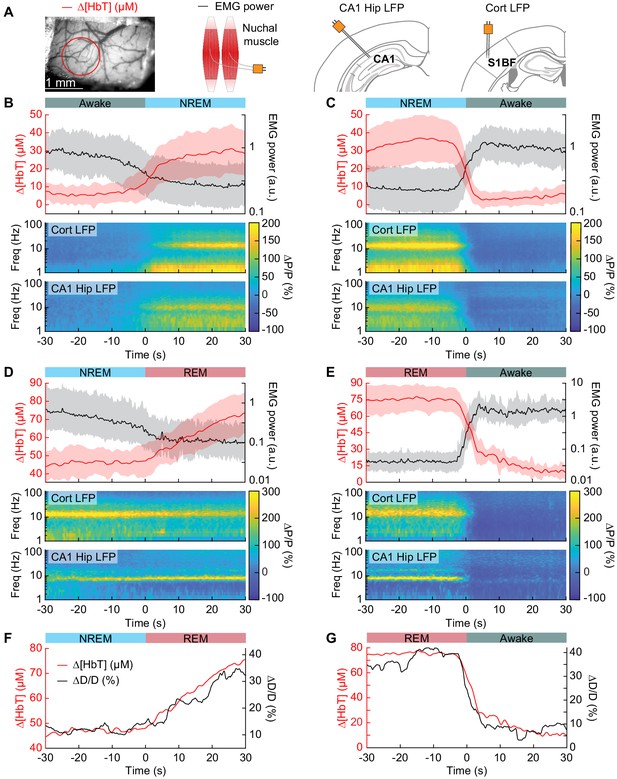
Vasodilation tracks transitions between arousal states.
(A) Schematic of each data type. (B) Transition from periods classified as rfc-Awake into periods classified as rfc-NREM. Top: Average change in total hemoglobin ∆[HbT] within the ROI and normalized change in EMG power. Shaded regions indicate ± 1 standard deviation (n = 14 mice, 28 hemispheres). (C) Transition from periods classified as rfc-NREM into periods classified as rfc-Awake. (D) Transition from periods classified as rfc-NREM into periods classified as rfc-REM. (E) Transitions from periods classified as rfc-REM into periods classified as rfc-Awake. (F) Mean arteriole diameter during the transition of NREM into REM (n = 5 mice, 8 arterioles). (G) Mean arteriole diameter during the transition from REM into Awake (n = 5 mice, 8 arterioles). Note that the EMG scales are different across conditions.
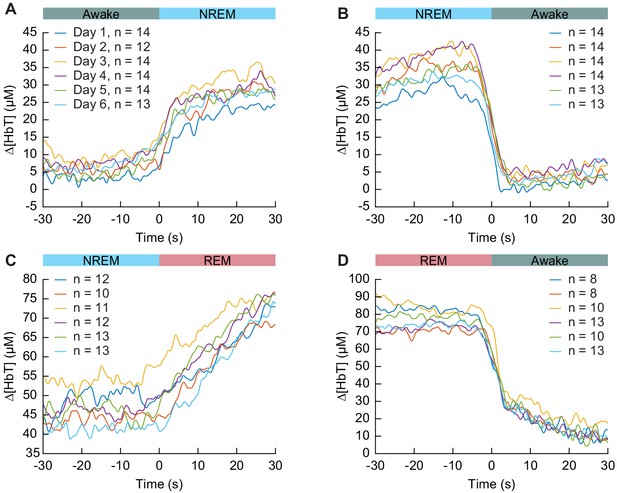
Transitional changes in hemodynamics are consistent across each day.
Average change in total hemoglobin ∆[HbT] for different days for various behavioral state transitions. Each colored line indicates a unique day of imaging (n = 14 mice). (A) Transition from rfc-Awake to rfc-NREM. (B) Transition from rfc-NREM to rfc-Awake. (C) Transition from rfc-NREM to rfc-REM. (D) Transitions from rfc-REM to rfc-Awake.
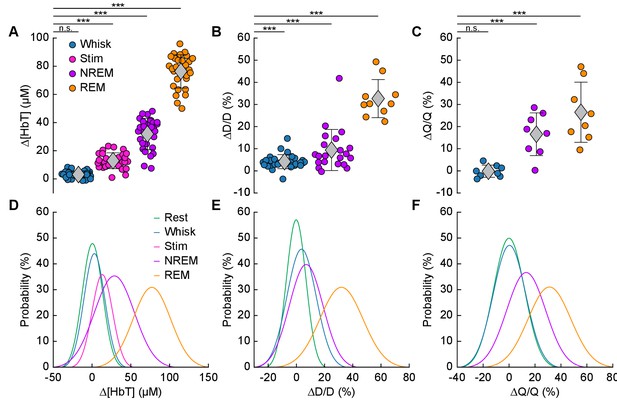
Increases in blood volume and arterial diameter during NREM and REM sleep.
(A) Average change in total hemoglobin ∆[HbT] within the ROI. Circles represent individual hemispheres of each mouse, diamonds represent population averages, with error bar showing ±1 standard deviation (n = 14 mice, 28 hemispheres). (B) Average change in peak arteriole diameter ∆D/D (%) (n = 6 mice, 29 arterioles for whisking; n = 6 mice, 21 arterioles for contiguous NREM; n = 5 mice, 10 arterioles for contiguous REM). (C) Average change in volumetric flux (∆Q/Q, %) measured with laser Doppler flowmetry during different arousal states (n = 8 mice). (D) Probability distribution of ∆[HbT] during each arousal state. (E) Probability distribution of ∆D/D (%) during each arousal state. (F) Probability distribution of ∆Q/Q (%) during each arousal state. *p<0.05, **p<0.01, ***p<0.001 GLME.
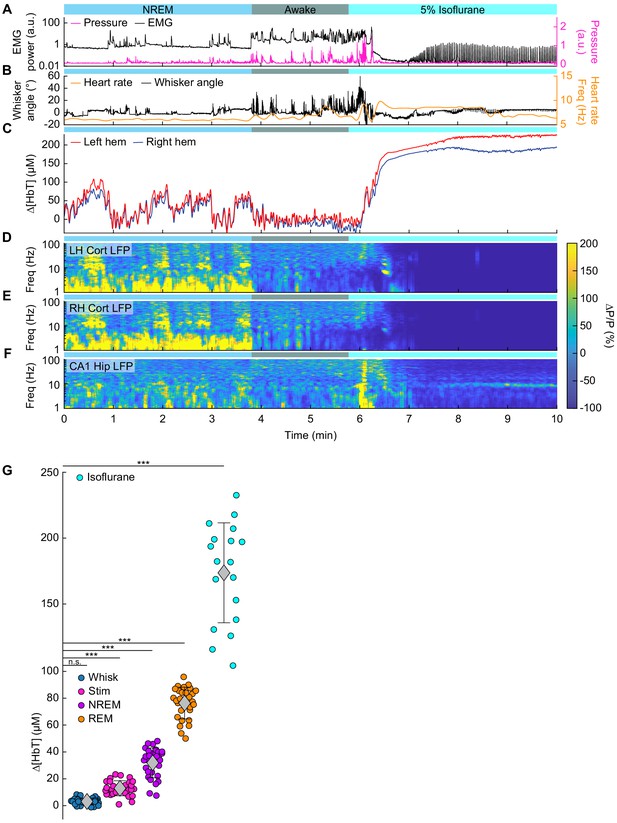
Isoflurane drives larger vasodilations than sleep.
Example showing the blood volume and neural changes accompanying transitions between NREM and awake states followed by administration of isoflurane. Isoflurane is a potent vasodilator and causes the cortical vasculature to reach a maximum or near-maximum saturation in blood volume. (A) Plot of nuchal muscle EMG power and body motion via a pressure sensor located beneath the mouse. Large oscillations in EMG are due to heavy breathing during anesthesia. (B) Plot of the whisker position and heart rate. (C) Changes in total hemoglobin ∆[HbT] within the ROIs over the putative vibrissa cortex. Inset shows images of the two windows and respective ROIs. (D) Normalized left vibrissae cortex LFP power (∆P/P). (E) Normalized right vibrissae cortex LFP power. (F) Normalized CA1 LFP power. (G) Average change in total hemoglobin ∆[HbT] within the ROI during different arousal states. Circles represent individual hemispheres of each mouse and diamonds represent population averages, with error bar showing ±1 standard deviation (n = 14 mice, 28 hemispheres; Isoflurane: n = 9, 18 hemispheres). *p<0.05, **p<0.01, ***p<0.001 GLME.
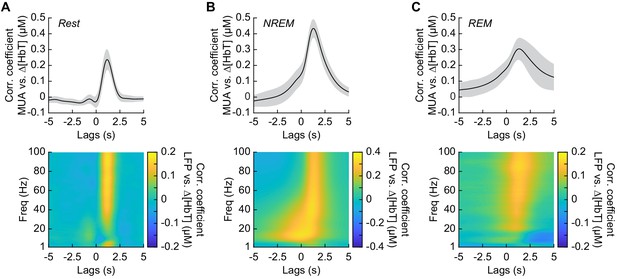
Neurovascular coupling is strongest during NREM sleep.
Cross-correlation between neural activity and changes in total hemoglobin ∆[HbT] during different arousal states, averaged across hemispheres. MUA power [300–3000 Hz] (top) and the LFP [1–100 Hz] (bottom) were consistently correlated with hemodynamics to varying degrees. (A) Awake rest. (B) Contiguous NREM sleep. (C) Contiguous REM sleep. Shaded regions indicate ±1 standard deviation (n = 14 mice, 28 hemispheres).
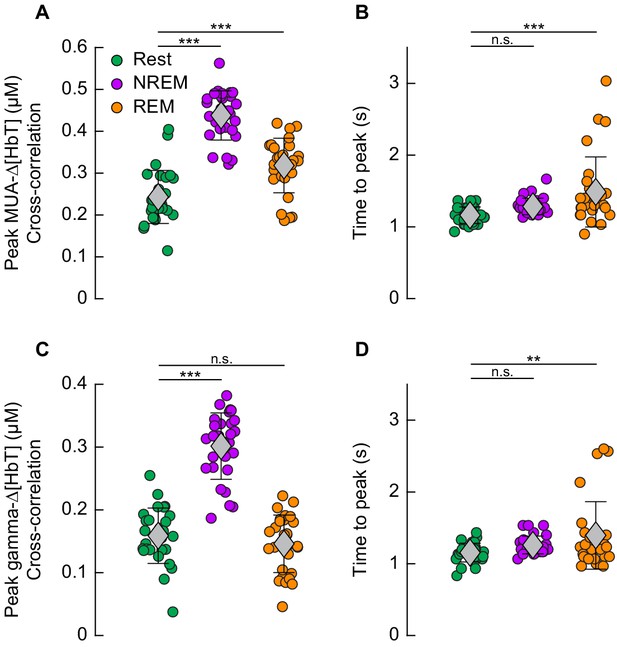
Neurovascular coupling dynamics change with arousal state.
Cross-correlation between neural activity and changes in total hemoglobin ∆[HbT] during different arousal states, averaged across hemispheres. MUA power [300–3000 Hz] and the LFP gamma band [30–100 Hz] were consistently correlated with hemodynamics to varying degrees, dependent on arousal state. (A) Peak cross-correlation between MUA and ∆[HbT]. (B) Time-to-peak of the cross-correlation between MUA and ∆[HbT]. (C) Peak cross-correlation between gamma band and ∆[HbT]. (D) Time-to-peak of the cross-correlation between gamma band and ∆[HbT]. Error bars indicate ±1 standard deviation (n = 14 mice, 28 hemispheres). *p<0.05, **p<0.01, ***p<0.001 GLME.
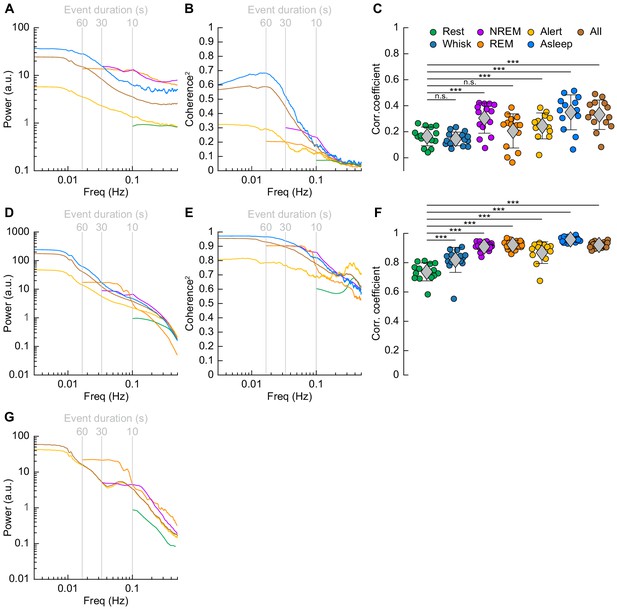
Correlations in neural activity and blood volume between hemispheres increase during sleep.
(A) Mean gamma band power spectral density during different arousal states. (B) Mean coherence (between hemispheres) in the changes in the envelope (≤1 Hz) of gamma band power [30–100 Hz] between left and right vibrissa cortex during different arousal states. (C) Average gamma band power Pearson’s correlation coefficient between left and right vibrissa cortex during different arousal states. Circles represent individual mice and diamonds represent population averages ± 1 standard deviation. (D-F) Same as in A-C except for the changes in total hemoglobin ∆[HbT]. MoC2 between the left and right somatosensory cortex for gamma band power and ∆[HbT] during each arousal state exceeded the 95% confidence level for all frequencies below 1 Hz. (A-F) n = 14 mice (n*two hemispheres in A,D) for all arousal states except Alert: n = 12 mice, Asleep: n = 13 mice. (G) Mean arteriole ∆D/D power spectral density during different arousal states (Rest: n = 6 mice, 29 arterioles, NREM: n = 6 mice, 21 arterioles, REM: n = 5 mice, 10 arterioles, Awake: n = 6 mice, 27 arterioles, All data: n = 6 mice, 29 arterioles). *p<0.05, **p<0.01, ***p<0.001 GLME.
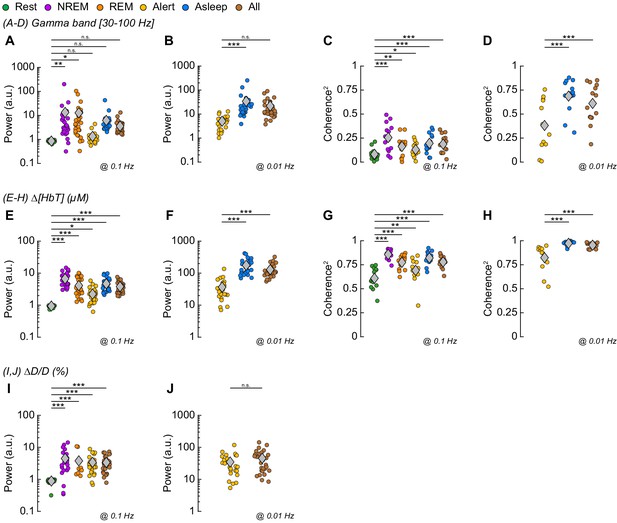
Arousal state dependence of low-frequency neural power and coherence2.
Spectral power and MoC2 at 0.1 or 0.01 Hz during different arousal states. (A–H) n = 14 mice (n*two hemispheres in A,B,E,F) for all arousal states except Alert: n = 12 mice, Asleep: n = 13 mice. (A–D) Gamma band power. (E–H) ∆[HbT]. (I,J) Spectral power at 0.1 or 0.01 Hz during different arousal states for arteriole ∆D/D (Rest: n = 6 mice, 29 arterioles, NREM: n = 6 mice, 21 arterioles, REM: n = 5 mice, 10 arterioles, Awake: n = 6 mice, 27 arterioles, All data: n = 6 mice, 29 arterioles). Circles represent individual hemispheres of each mouse (A–H) or individual arterioles (I,J) and diamonds represent population averages, with error bar showing ±1 standard deviation. Data is presented in Tables 1, 2, 3, 4, 5. *p<0.05, **p<0.01, ***p<0.001 GLME.
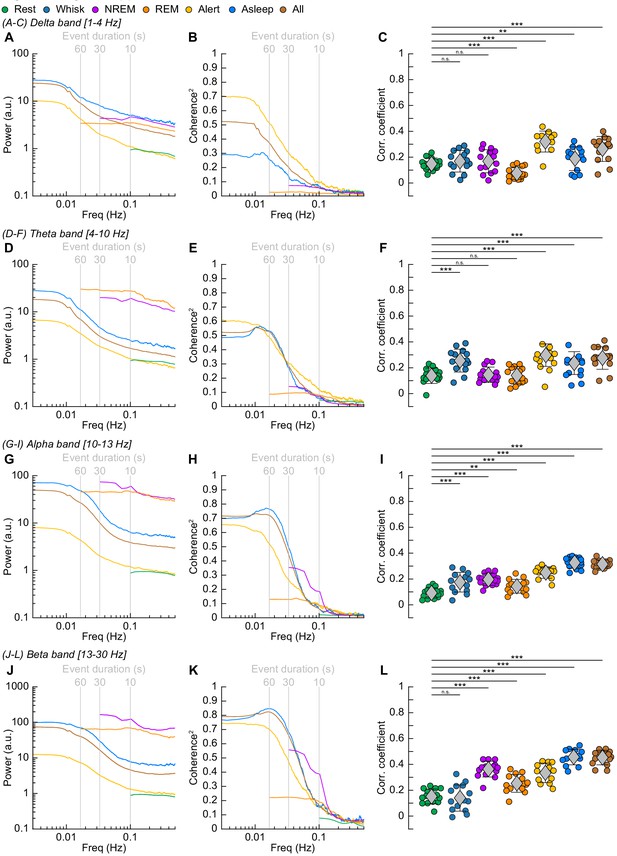
Correlations in neural activity between hemispheres increase during sleep.
(A) Mean delta band power spectral density during different arousal states. (B) Mean coherence (between hemispheres) in the changes in the envelope (≤1 Hz) of delta band power [1–4 Hz] between left and right vibrissa cortex during different arousal states. (C) Average delta band power Pearson’s correlation coefficient between left and right vibrissa cortex during different arousal states. Circles represent individual mice and diamonds represent population averages ± 1 standard deviation. (D–F) Same as in (A–C) except for the theta band power [4–10 Hz]. (G–I) Same as in (A–C) except for the alpha band power [10–13 Hz]. (J–L) Same as in (A–C) except for the beta band power [13–30 Hz]. MoC2 between the left and right somatosensory cortex for each LFP band during each arousal state exceeded the 95% confidence level for all envelope frequencies below 1 Hz. (A–L) n = 14 mice (n*two hemispheres in A,D,G,J) for all arousal states except Alert: n = 12 mice, Asleep: n = 13 mice. Data is presented in Supplementary file 1. *p<0.05, **p<0.01, ***p<0.001 GLME.
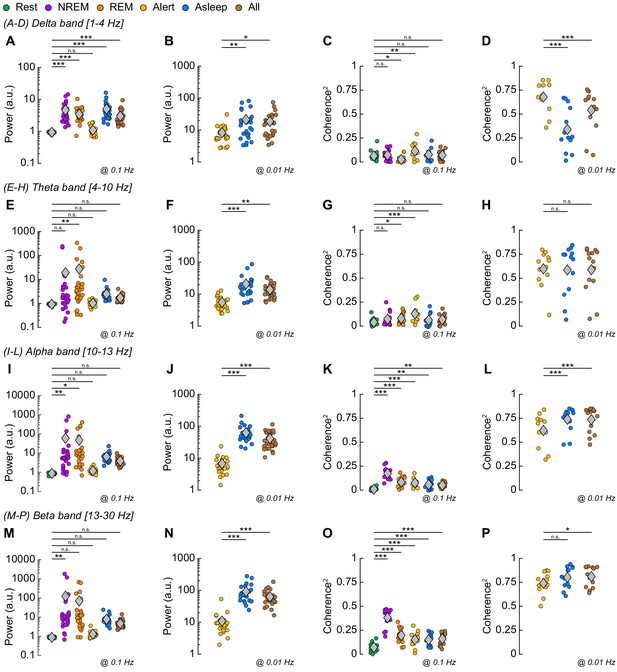
Arousal state dependence of low-frequency neural power and coherence.
Spectral power and MoC2 at 0.1 or 0.01 Hz for different arousal states. (A–P) n = 14 mice (n*two hemispheres in A,B,E,F,I,J,M,N) for all arousal states except Alert: n = 12 mice, Asleep: n = 13 mice. (A–D) Delta band power. (E–H) Theta band power. (I–L) Alpha band power. (M–P) Beta band power. Circles represent individual hemispheres of each mouse and diamonds represent population averages, with error bar showing ±1 standard deviation. Data is presented in Supplementary file 1. *p<0.05, **p<0.01, ***p<0.001 GLME.
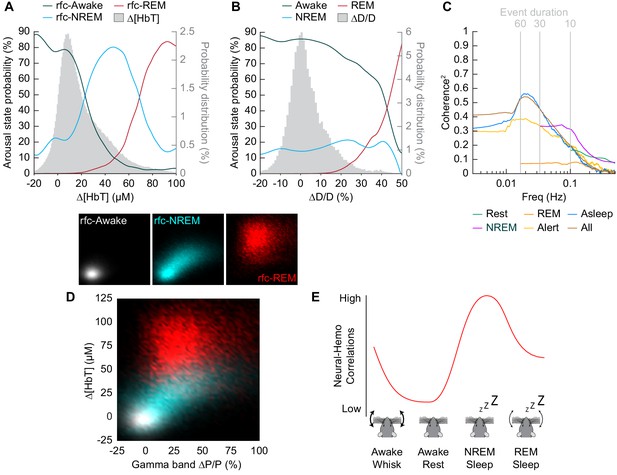
Influence of arousal state on vascular correlations to ongoing neural activity.
(A) Probability of being in a given arousal state as a function of mean ∆[HbT]. As ∆[HbT] increases, so does the probability that the animal is asleep. Temporal resolution is 5 s, ∆[HbT] bins have a 1 µM resolution. (B) Probability of being in a given arousal state as a function of mean ∆D/D. As ∆D/D increases, the probability that the animal is asleep is not well defined until reaching very large vasodilation. Temporal resolution is 5 s, ∆D/D bins are 1% resolution. (C) MoC2 between the envelope (≤1 Hz) of gamma band power and ∆[HbT] during each arousal state. Three additional states of alert, asleep, and all data are included to extend into the ultra-low frequencies. n = 14 mice. Alert arousal state n = 12; Asleep arousal state n = 13; All data arousal state n = 14. (D) Relationship between the gamma band power and ∆[HbT] during each arousal state. 5 s resolution. (E) Schematic demonstrating the observed relationship between ∆[HbT] and gamma band power during each arousal state.
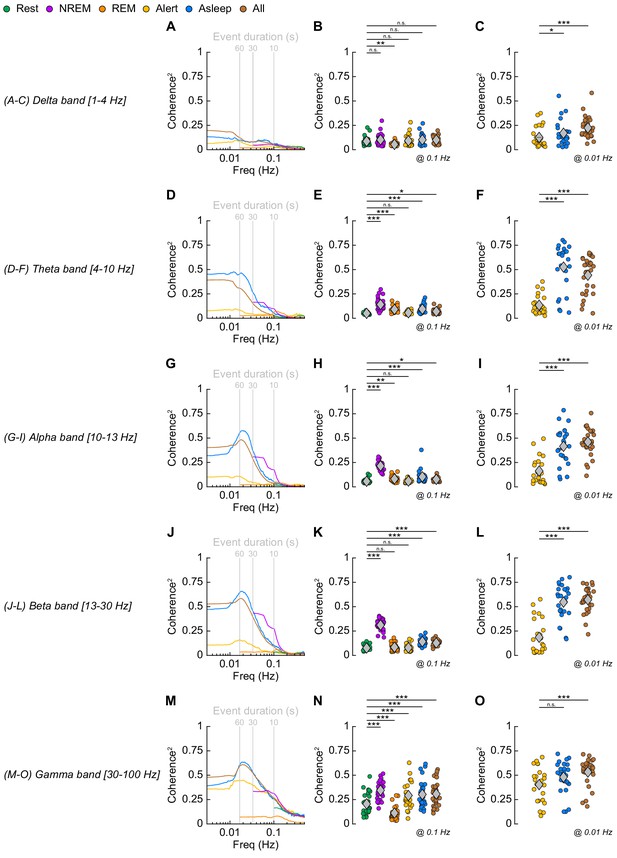
Arousal state dependence of low-frequency neural-hemo coherence.
Coherence between LFP and changes total hemoglobin ∆[HbT] evaluated at 0.1 and 0.01 Hz. (A) Mean MoC2 between the envelope (≤1 Hz) of delta band power [1–4 Hz] and ∆[HbT] in a single cortical hemisphere during different arousal states at 0.1 (B) and 0.01 (C) Hz. (D–F) Same as in (A–C) except for theta band power [4–10 Hz]. (G–I) Same as in (A–C) except for alpha band power [10–13 Hz]. (J–L) Same as in (A–C) except for beta band power [13–30 Hz]. (M–O) Same as in (A–C) except for gamma band power [30–100 Hz]. (A–O) n = 14 mice (n*two hemispheres) for all arousal states except Alert: n = 12 mice, Asleep: n = 13 mice. Data is presented in Supplementary file 1. *p<0.05, **p<0.01, ***p<0.001 GLME.
Videos
Arousal and associated changes in neural activity and hemodynamics in the awake state.
Whisker motion, IOS reflectance, and eye camera activity are shown alongside measurements of neural activity and hemodynamics. The awake state shows a large amount of whisker motion and elevations in heart rate. The eye is open. High-frequency neural activity increases and low-frequency activity decreases during whisking events, with corresponding decreases in reflectance. An increase in blood volume/[HbT] corresponds to a decrease in pixel reflectance, as more light is absorbed by the increase in hemoglobin.
Arousal and associated changes in neural activity and hemodynamics during NREM sleep.
Whisker motion, IOS reflectance, and eye camera activity are shown alongside measurements of neural activity and hemodynamics. The NREM state shows little whisker motion and a lower heart rate. The eye is still open. Low-frequency (delta band) cortical neural activity is elevated, and there are large changes in reflectance.
Arousal and associated changes in neural activity and hemodynamics during REM sleep.
Whisker motion, IOS reflectance, and eye camera activity are shown alongside measurements of neural activity and hemodynamics. The transition into the REM state shows an increase in whisker motion and an increase heart rate, similar to the awake state. The eye remains open. The neural activity in the hippocampal theta band and upper frequencies of cortical neural activity increase while [HbT] increases substantially.
Tables
Spectral power in gamma band, ∆[HbT], and arteriole diameter (∆D/D) at 0.1 Hz.
Spectral Power at 0.1 Hz | Awake Rest | Cont. NREM | Cont. REM | Alert | Asleep | All Data |
---|---|---|---|---|---|---|
Gamma band Power (a.u.) | 0.9 ± 0.1 | 13.3 ± 37.4 (p<0.01) | 12.8 ± 23.4 (p<0.02) | 1.3 ± 0.8 (p<0.93) | 6.2 ± 8 (p<0.29) | 3.6 ± 2.5 (p<0.58) |
∆[HbT] Power (a.u.) | 0.9 ± 0.1 | 6.7 ± 3.4 (p<7.6 × 10−19) | 4.1 ± 2.6 (p<1.3 × 10−7) | 2.2 ± 1.3 (p<0.03) | 4.7 ± 2.3 (p<1.3 × 10−9) | 3.8 ± 1.6 (p<1.3 × 10−6) |
∆D/D Power (a.u.) | 0.9 ± 0.1 | 4.5 ± 3.9 (p<2.7 × 10−10) | 3.8 ± 3.7 (p<2.1 × 10−5) | 3.4 ± 2.1 (p<9.3 × 10−7) | 3.4 ± 1.9 (p<5.8 × 10−7) |
-
Mean ± 1 standard deviation. p-values as a comparison to ‘Rest’. Shaded region indicates insufficient data for that arousal state.
Spectral power in gamma band, ∆[HbT], and arteriole diameter (∆D/D) at 0.01 Hz.
Spectral Power at 0.01 Hz | Alert | Asleep | All Data d |
---|---|---|---|
Gamma band Power (a.u.) | 5 ± 3.2 | 34 ± 51 (p<0.001) | 21.7 ± 20.6 (p<0.06) |
∆[HbT] Power (a.u.) | 36.5 ± 28.6 | 176.2 ± 98.9 (p<5.3 × 10−10) | 128.9 ± 63.8 (p<7.9 × 10−6) |
∆D/D Power (a.u.) | 34.9 ± 25 | 45.6 ± 33.6 (p<0.06) |
-
Mean ± 1 standard deviation. p-values as a comparison to ‘Alert’. Shaded region indicates insufficient data for that arousal state.
Magnitude of Coherence2 of bilateral gamma band and bilateral ∆[HbT] at 0.1 Hz.
Coherence2 at 0.1 Hz | Awake Rest | Cont. NREM | Cont. REM | Alert | Asleep | All Data |
---|---|---|---|---|---|---|
Gamma band (Coherence2) | 0.08 ± 0.06 | 0.26 ± 0.14 (p<4.8 × 10−8) | 0.16 ± 0.1 (p<0.006) | 0.13 ± 0.08 (p<0.04) | 0.2 ± 0.11 (p<0.0002) | 0.19 ± 0.09 (p<0.0004) |
∆[HbT] (Coherence2) | 0.61 ± 0.11 | 0.86 ± 0.05 (p<1.2 × 10−16) | 0.77 ± 0.08 (p<5.7 × 10−10) | 0.69 ± 0.14 (p<0.002) | 0.82 ± 0.07 (p<2.9 × 10−13) | 0.78 ± 0.06 (p<2.6 × 10−10) |
-
Mean ± 1 standard deviation. p-values as a comparison to ‘Rest’.
Magnitude of Coherence2 of bilateral gamma band and bilateral ∆[HbT] at 0.01 Hz.
Coherence2 at 0.01 Hz | Alert | Asleep | All Data |
---|---|---|---|
Gamma band (Coherence2) | 0.38 ± 0.27 | 0.69 ± 0.18 (p<7.2 × 10−6) | 0.61 ± 0.21 (p<0.0002) |
∆[HbT] Power (Coherence2) | 0.82 ± 0.15 | 0.97 ± 0.02 (p<1.5 × 10−5) | 0.95 ± 0.03 (p<7.5 × 10−5) |
-
Mean ± 1 standard deviation. p-values as a comparison to ‘Alert’.
Pearson’s correlation coefficients of bilateral gamma band and bilateral ∆[HbT].
Pearson’s Correlation Coef. | Awake Rest | Whisking | Cont. NREM | Cont. REM | Alert | Asleep | All Data |
---|---|---|---|---|---|---|---|
Gamma band (R) | 0.16 ± 0.07 | 0.15 ± 0.05 (p<0.47) | 0.3 ± 0.12 (p<6.1 × 10−7) | 0.21 ± 0.13 (p<0.11) | 0.26 ± 0.1 (p<0.0004) | 0.35 ± 0.14 (p<4.7 × 10−10) | 0.33 ± 0.11 (p<8.5 × 10−9) |
∆[HbT] (R) | 0.74 ± 0.06 | 0.82 ± 0.09 (p<3 × 10−8) | 0.9 ± 0.03 (p<3.6 × 10−20) | 0.91 ± 0.03 (p<4.9 × 10−22) | 0.87 ± 0.07 (p<7.9 × 10−15) | 0.96 ± 0.02 (p<8.1 × 10−28) | 0.93 ± 0.02 (p<1.1 × 10−24) |
-
Mean ± 1 standard deviation. p-values as a comparison to ‘Rest’.
Reagent type (species) or resource | Designation | Source or reference | Identifiers | Additional information |
---|---|---|---|---|
Strain, strain background (M. musculus) | Strain: C57BL6/J | Jackson Laboratory | Stock No: 000664 | |
Chemical compound, drug | Fluorescein isothiocyanate–dextran 150 kDa | Sigma-Aldrich | Stock No: FD150S | 100 µL, 5% (weight/volume) |
Software, algorithm | Data analysis software | MathWorks | MATLAB 2019a | https://github.com/KL-Turner/Turner_Gheres_Proctor_Drew_eLife2020; Turner et al., 2020 |
Software, algorithm | IOS and 2PLSM data acquisition software | National Instruments | LabVIEW 2018 | https://github.com/DrewLab/LabVIEW-DAQ; Drew, 2020 |
Software, algorithm | 2PLSM data acquisition software | Sutter Instrument | MSCAN 2015 | |
Other | PFA-coated tungsten microwires | A-M Systems | Stock No: #795500 | |
Other | PFA-coated 7-strand stainless-steel microwires | A-M Systems | Stock No: #793200 |
Additional files
-
Supplementary file 1
Supplemental tables.
- https://cdn.elifesciences.org/articles/62071/elife-62071-supp1-v3.docx
-
Transparent reporting form
- https://cdn.elifesciences.org/articles/62071/elife-62071-transrepform-v3.docx