Modulation of flight and feeding behaviours requires presynaptic IP3Rs in dopaminergic neurons
Abstract
Innate behaviours, although robust and hard wired, rely on modulation of neuronal circuits, for eliciting an appropriate response according to internal states and external cues. Drosophila flight is one such innate behaviour that is modulated by intracellular calcium release through inositol 1,4,5-trisphosphate receptors (IP3Rs). Cellular mechanism(s) by which IP3Rs modulate neuronal function for specific behaviours remain speculative, in vertebrates and invertebrates. To address this, we generated an inducible dominant negative form of the IP3R (IP3RDN). Flies with neuronal expression of IP3RDN exhibit flight deficits. Expression of IP3RDN helped identify key flight-modulating dopaminergic neurons with axonal projections in the mushroom body. Flies with attenuated IP3Rs in these presynaptic dopaminergic neurons exhibit shortened flight bouts and a disinterest in seeking food, accompanied by reduced excitability and dopamine release upon cholinergic stimulation. Our findings suggest that the same neural circuit modulates the drive for food search and for undertaking longer flight bouts.
Introduction
The Inositol-1, 4, 5-trisphosphate receptor (IP3R) is an Endoplasmic Reticulum (ER) resident ligand-gated calcium (Ca2+) channel found in metazoans. In neurons, the IP3R is activated through multiple classes of signaling molecules that include neuromodulators such as neuropeptides, neurotransmitters, and neurohormones. Studies from ex vivo vertebrate neurons have identified a role for the IP3R in multiple cellular processes including regulation of neurite growth (Takei et al., 1998; Xiang et al., 2002), synaptic plasticity (Fujii et al., 2000; Nishiyama et al., 2000) and more recently pre-synaptic neurotransmitter release (Gomez et al., 2020). However, the relevance of IP3-mediated signaling mechanisms to cellular processes and subsequent behavioural and neurophysiological outputs need better understanding. In non-excitable cells Ca2+ release through the IP3R regulates a range of cellular events including growth, secretion (Inaba et al., 2014), gene expression (Ouyang et al., 2014), and mitochondrial function (Cárdenas et al., 2010; Bartok et al., 2019). In excitable cells such as neurons, identifying cellular functions of the IP3R is more complex because in addition to IP3-mediated Ca2+ release, there exist several plasma-membrane localised ion channels that bring in extracellular Ca2+ in response to neurotransmitters and changes in membrane excitability.
Three IP3R isotypes, IP3R1, 2 and 3 are encoded by mammalian genomes (Furuichi, 1994; Taylor et al., 1999). Of these, IP3R1 is the most prevalent isoform in neurons and is relevant in the context of several neurodegenerative disorders (Terry et al., 2018). Human mutations in IP3R1 cause Spinocerebellar ataxia 15 (SCA15), SCA29 and Gillespie syndrome (Hasan and Sharma, 2020). Disease causing IP3R1 mutations span different domains but several are clustered in the amino terminal IP3 binding region, from where they impact ER-Ca2+ release when tested in mammalian cell lines (Ando et al., 2018). A common feature of these neurological disorders is loss of motor-coordination or ataxia. The ataxic symptoms arise primarily from malfunction and/or degeneration of cerebellar Purkinje neurons where the IP3R1 is expressed abundantly in the soma, dendrites, and axons. Dendritic expression of the IP3R in Purkinje neurons determines Long Term Depression (LTD), a form of post-synaptic plasticity (Miyata et al., 2000). Interestingly, a recent study in Drosophila also identified the IP3R as an essential component of post-synaptic plasticity, required for decoding the temporal order of a sensory cue and a reward stimulus, in neurons of a higher brain centre, the Mushroom Body (Handler et al., 2019). Somatic expression of the IP3R very likely contributes to maintenance of cellular Ca2+ homeostasis (Berridge, 2016). A pre-synaptic role for the IP3R has been demonstrated in the Drosophila neuromuscular junction (Shakiryanova et al., 2011), motor neurons (Klose et al., 2010) and most recently in axonal projections of Purkinje neurons (Gomez et al., 2020). Physiological and behavioural significance of pre-synaptic IP3/Ca2+ signals in either case,however, remain speculative.
To understand how IP3R alters neuronal function and related neurophysiology and behaviour, we have in the past studied several mutants for the single IP3R gene (itpr) in Drosophila melanogaster (Joshi et al., 2004). The Drosophila IP3R shares 60% sequence identity, similar domain organisation, biophysical and functional properties with mammalian IP3Rs (Chakraborty and Hasan, 2012; Srikanth and Hasan, 2004). Several hypomorphic and heteroallelic combinations of Drosophila IP3R mutants are viable and exhibit flight deficits ranging from mild to strong, the focus of which lies in aminergic neurons (Banerjee et al., 2004). However, such mutants do not easily allow cell specific attenuation of IP3R function. In this context, a dominant-negative mutant form of mammalian IP3R1, generated recently, abrogated IP3R function in mammalian cell lines (Alzayady et al., 2016). The mammalian dominant negative IP3R1 gene was based on the finding that all monomers in the IP3R tetramer need to bind IP3 for channel opening (Alzayady et al., 2016). Failure of a single IP3R monomer to bind IP3 renders the resultant IP3R tetramer non-functional. Drosophila IP3Rs are also tetrameric suggesting that a similar strategy for generating a dominant-negative IP3R could be used for cell-specific studies of neuronal function and behaviour. Consequently, we generated and characterised a Drosophila IP3R dominant negative (IP3RDN) transgene. Cell-specific expression of Drosophila IP3RDN allowed the identification of two pairs of flight modulating dopaminergic neurons. Neuromodulatory signals received by these neurons stimulate IP3/Ca2+ signals to regulate critical aspects of pre-synaptic cellular physiology with significant impact on flight and feeding behaviour.
Results
Ex vivo characterisation of a dominant negative IP3R
To understand how Ca2+ release through IP3R affects cellular properties of neurons, we designed a mutant itpr cDNA to function as a dominant negative upon overexpression in wild-type Drosophila neurons. The dominant negative construct (ItprDN) was designed based on previous studies in mammalian IP3Rs (Figure 1A; Alzayady et al., 2016). Three conserved basic residues in the ligand binding domain (R272, K531, and Q533) of the Drosophila IP3R cDNA were mutated to Glutamine (Q) and the resultant mutant cDNA was used to generate GAL4/UAS inducible transgenic strains (UASItprDN) as described in Materials and methods. Expression from the ItprDN construct was validated by western blots of adult fly head lysates. IP3R levels were significantly enhanced in fly heads with ItprDN as compared to genetic controls and were equivalent to overexpression of a wild-type IP3R transgene (Itpr+; Figure 1B, Figure 1—source data 1). IP3-mediated calcium release from the IP3R in the presence of ItprDN, was tested on previously characterised glutamatergic interneurons in the larval ventral ganglion known to respond to the muscarinic acetylcholine receptor (mAChR) ligand Carbachol. These neurons can be marked with a GAL4 strain (vGlutVGN6341; Jayakumar et al., 2016; Jayakumar et al., 2018). Changes in cytosolic calcium were measured by visualising Ca2+ dependent fluorescence changes of a genetically encoded Ca2+ sensor GCaMP6m (Chen et al., 2013) in ex vivo preparations. vGlutVGN6341 marked neurons expressing IP3RDN exhibit reduced as well as delayed Ca2+ responses to Carbachol stimulation as compared to controls (Figure 1C). Ca2+- released from the IP3R is also taken up by mitochondria (Bartok et al., 2019). Hence we measured mitochondrial Ca2+ uptake post Carbachol stimulation using mitoGCaMP, a mitochondrial targeted fluorescence sensor for Ca2+ (Lutas et al., 2012). Similar to cytosolic GCaMP, attenuated Ca2+ responses, that took longer to reach peak values, were observed upon carbachol stimulation in presence of IP3RDN (Figure 1D). We attribute the residual Ca2+ release observed in the cytosol and mitochondria to the persistence of some IP3R tetramers with all four wild-type subunits, encoded by the native itpr gene. In summary, both cytosolic and mitochondrial Ca2+ measurements confirmed the efficacy of the Drosophila IP3RDN for attenuating IP3-mediated Ca2+ release from ER stores upon GPCR stimulation in Drosophila neurons.
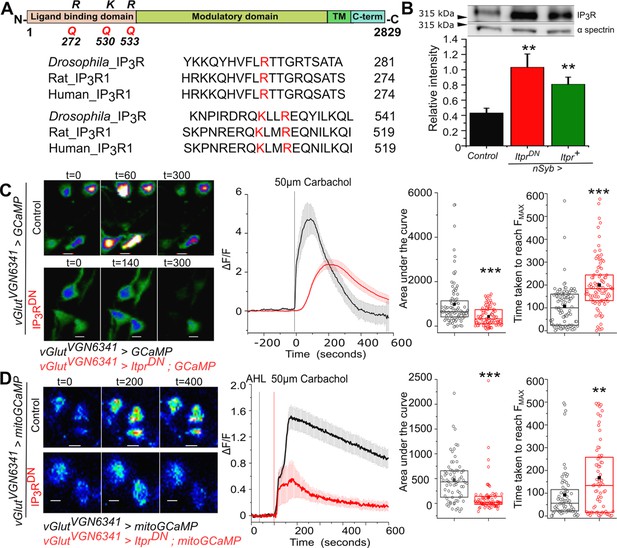
Generation and characterisation of a dominant negative IP3R (IP3RDN).
(A) Domain organisation of the Drosophila IP3R. The Arginine (R) and Lysine (K) residues mutated to Glutamine (Q) to generate a dominant-negative IP3R (IP3RDN) are shown (top). Alignment of the Drosophila IP3R with Rat IP3R1 and Human IP3R1 in the region of the mutated amino acids. All three residues (red) are conserved (below). (B) Significantly higher immuno-reactivity against the IP3R is observed upon pan-neuronal (nsybGAL4) expression of IP3RDN (UASitprDN) and IP3R (UASitpr+) in adult heads. Statistical comparison was made with respect to Canton S as control, n=5, **p< 0.05, t-test. (C) Representative images show changes in cytosolic Ca2+ in larval neurons of the indicated genotypes as judged by GCaMP6m fluorescence at the indicated time intervals after stimulation with Carbachol (Scale bars indicate 5 μm). Warmer colors denote increase in [Ca2+]cyt. Mean traces of normalized GCaMP6m fluorescence in response to Carbachol (4s/frame) in vGlutVGN6341GAL4 marked neurons, with (red) and without (black) IP3RDN (ΔF/F ± SEM) (middle panel). Area under the curve (right) was quantified from 0 - 420s from the traces in the middle. Time taken to reach peak fluorescence (FMAX) for individual cells (far right). vGlutVGN6341GAL4 > UAS GCaMP N = 6 brains, 92 cells; vGlutVGN6341GAL4 > UAS ItprDN; UAS GCaMP N = 6 brains, 95 cells. ***p< 0.005 (Two tailed Mann-Whitney U test). (D) Representative images show changes in mitochondrial Ca2+ in larval neurons of the indicated genotypes as judged by mitoGCaMP6m fluorescence at the indicated time intervals after stimulation with Carbachol (Scale bars indicate 5 μm). Warmer colors denote increase in [Ca2+]cyt. Mean traces of normalized mitoGCaMP6m fluorescence in response to Carbachol (2s/frame) in vGlutVGN6341GAL4 marked neurons, with (red) and without (black) IP3RDN (ΔF/F ± SEM) (middle panel). Area under the curve (right) was quantified from 100 to 400s from the traces in the middle. Time taken to reach peak fluorescence (FMAX) for individual cells (far right). vGlutVGN6341GAL4 > UAS mitoGCaMP N = 5 brains, 69 cells; vGlutVGN6341GAL4 > UAS ItprDN; UAS mitoGCaMP N = 5 brains, 68 cells. ***p< 0.005, **p< 0.05 (Two tailed Mann-Whitney U test).
-
Figure 1—source data 1
Western Blots for IP3R upon pan-neuronal expression of IP3RDN and IP3R WT in adult heads.
- https://cdn.elifesciences.org/articles/62297/elife-62297-fig1-data1-v1.pptx
The IP3R is required in a subset of central dopaminergic neurons for maintenance of Drosophila flight
Next we tested the functional efficacy of ItprDN for attenuating neuronal function in Drosophila. From previous reports, we know that itpr mutants are flightless and their flight deficit can be rescued partially by overexpression of a wild-type cDNA construct (Itpr+; Venkatesh et al., 2001) in monoaminergic neurons (Banerjee et al., 2004). Subsequent studies identified mild flight deficits upon RNAi mediated knock down of the IP3R in dopaminergic neurons (DANs) (Pathak et al., 2015) when tethered flight was tested for 30 s. Knock-down of the IP3R in serotonergic neurons, that form another major subset of the tested monoaminergic neurons, did not give a flight deficit (Sadaf et al., 2012). Here we tested if pan-neuronal expression of the IP3RDN affects longer flight bout durations in a modified tethered flight assay lasting for 15 min (see Materials and methods and Manjila and Hasan, 2018). Flies with pan-neuronal nsybGAL4-driven expression of ItprDN exhibit significantly reduced flight bouts (281.4 ± 38.9 s) as compared to the appropriate genetic controls ItprDN/+ (773.3 ± 30.4 s) and nsyb/+ (670.6 ± 41.3 s) (Figure 2—figure supplement 1A, Figure 2—source data 4). Stronger deficits in flight bout durations (185.2 ± 33.7 s) were obtained by expression of ItprDN in Tyrosine Hydroxylase expressing cells, that include a majority of dopaminergic neurons (THGAL4; Friggi-Grelin et al., 2003), as well as in a dopaminergic neuron subset (260 ± 30.5 s) marked by THD’GAL4 (Liu et al., 2012; Figure 2A and B, Figure 2—source data 1). As additional controls the same GAL4 drivers were tested with overexpression of Itpr+. Interestingly, flight deficits were also observed upon overexpression of Itpr+ across all neurons (nsyb >Itpr+; 410 ± 31.6 s; Figure 2—figure supplement 1A), all TH-expressing cells (TH >Itpr+; 482.2 ± 41.4 s) and a dopaminergic neuron subset, (THD’>Itpr+; 433.5 ± 35.6 s; Figure 2A), although these were milder than with expression of ItprDN.
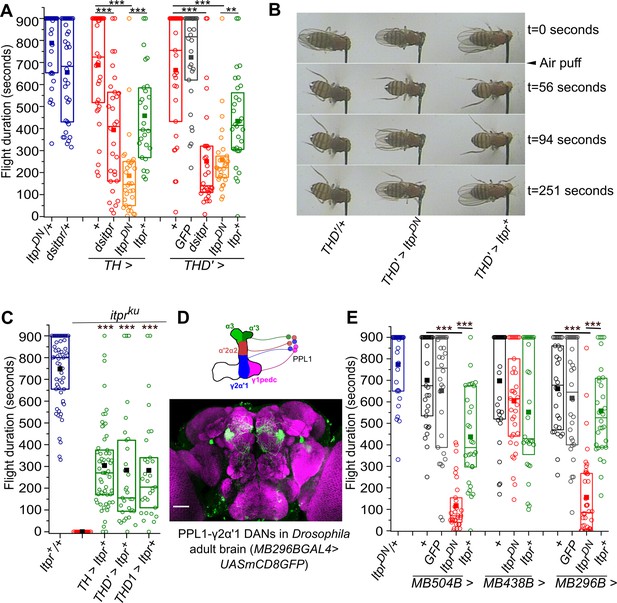
The IP3R is required in central dopaminergic neurons for maintaining long flight bouts.
(A) Flight deficits observed in flies expressing IP3R RNAi (dsitpr), IP3RDN (ItprDN) and IP3RWT (Itpr+) across all dopaminergic and TH-expressing cells (TH) as well as a subset of dopaminergic neurons (THD’). Flight times of flies of the indicated genotypes are represented as box plots where the box represents 25-75% of the distribution, each circle is the flight time of an individual fly, the small filled square represents mean flight time and the horizontal line is the median. Flight was tested in flies with GFP in THD’ marked neurons as an over-expression control. (B) Snapshots from flight videos of air puff stimulated flight bouts in the indicated genotypes at the specified time points. (C) Box plots (as in A above) represent flight bout durations of control and itpr mutant flies (itprku). Expression of a wild-type cDNA for the IP3R (UAS Itpr+) with indicated dopaminergic GAL4s rescued flight to a significant extent. (D) A schematic of PPL1 DANs projecting to different lobes of one half of the mushroom body (top); An adult brain with GFP expression (green) driven by MB296BGAL4. GFP expression is restricted to PPL1-γ2α′1 neurons and the γ2α′1 MB lobe. The brain neuropil is immunostained with anti Brp (purple). Scale bar = 50 μm. (E) Box plots (as in A) of flight bout durations in flies expressing IP3RDN (ItprDN) and IP3RWT (Itpr+) in the indicated PPL1 DAN splitGAL4 strains, not significant at p < 0.05 by Mann-Whitney U test (for C) or Kruskal-Wallis test (for A and E). Comparisons for significance were with the control values except where marked by a horizontal line. Comparisons for significance were with itpr mutants in C.
-
Figure 2—source data 1
Flight duration for flies upon perturbing IP3R signaling in dopaminergic neurons.
- https://cdn.elifesciences.org/articles/62297/elife-62297-fig2-data1-v1.xlsx
-
Figure 2—source data 2
Flight duration of itpr mutant flies after overexpressing a wild type itpr transgene in various dopaminergic subs.
- https://cdn.elifesciences.org/articles/62297/elife-62297-fig2-data2-v1.xlsx
-
Figure 2—source data 3
Flight duration of flies upon perturbing IP3R signaling in PPL1 neurons.
- https://cdn.elifesciences.org/articles/62297/elife-62297-fig2-data3-v1.xlsx
-
Figure 2—source data 4
Flight duration of flies upon perturbing IP3R signaling pan neuronally.
- https://cdn.elifesciences.org/articles/62297/elife-62297-fig2-data4-v1.xlsx
-
Figure 2—source data 5
Flight duration of itpr mutant flies after overexpressing a wild type itpr transgene in various neuronal subsets in a short flight assay.
- https://cdn.elifesciences.org/articles/62297/elife-62297-fig2-data5-v1.xlsx
-
Figure 2—source data 6
Flight duration of flies upon perturbing IP3R signaling in subsets of PPL1 .neurons.
- https://cdn.elifesciences.org/articles/62297/elife-62297-fig2-data6-v1.xlsx
To confirm that shorter flight bouts in flies expressing ItprDN are a consequence of reduced IP3R function in dopaminergic neurons and not an overexpression artefact, we expressed a previously validated Itpr RNAi (Agrawal et al., 2010) and recorded flight durations in an identical flight assay. Significantly reduced flight bouts, comparable to the flight deficits obtained upon expression of ItprDN, were observed by knockdown of the IP3R with nsybGAL4, THGAL4, and THD’GAL4 (Figure 2A, Figure 2—figure supplement 1A, Figure 2—video 1). These data confirm that IP3R function is necessary in the THD’ marked subset of dopaminergic neurons for maintenance of flight bouts. Further, it suggests that either decrease (ItprDN and Itpr RNAi) or increase (Itpr+) of IP3-mediated Ca2+ release in THD’ neurons affects flight bout durations.
As an independent test of IP3R requirement, the wild-type IP3R was overexpressed in dopaminergic neurons of an adult viable IP3R mutant, itprka1091/ug3 or itprku (Joshi et al., 2004). Overexpression of the IP3R in monoaminergic neurons of itpr mutants can rescue free flight measured for short durations of 5–10 secs (Banerjee et al., 2004). Short (30 s, Figure 2—figure supplement 1B, Figure 2—source data 5) and long (900 s; Figure 2C, Figure 2—source data 2) flight bouts were measured in a heteroallelic viable itpr mutant combination itprku, with Itpr+ overexpression in all neurons or in dopaminergic neurons and dopaminergic neuronal subsets. Pan-neuronal overexpression of the IP3R (nsyb >Itpr+) rescued short flight bouts partially in 10 out of 30 flies tested (Figure 2—figure supplement 1B). Interestingly, complete rescue of short flight was observed in flies rescued by IP3R overexpression in dopaminergic neurons and their TH-D subsets but not the TH-C’ subset (Figure 2—figure supplement 1B). Better rescue by IP3R overexpression in dopaminergic neurons suggests weak expression of nSybGAL4 in dopaminergic neurons, although this idea needs further verification. When tested for longer flight bouts, a partial rescue from dopaminergic neurons and TH-D subsets was observed (304.2 ± 25.1 s; TH >Itpr+) as compared to control flies (747.8 ± 19.4 s; Figure 2C), suggesting that the IP3R regulates flight bout durations from both dopaminergic and certain non-dopaminergic neurons (Agrawal et al., 2010). The TH-D and TH-C GAL4s express in anatomically distinct central dopaminergic neurons of which the THD1GAL4 and THD’GAL4 uniquely mark the PPL1 and PPM3 DAN clusters. Taken together, shorter flight bouts in THD >ItprDN flies and significant rescue of flight by THD >Itpr+ overexpression in flightless itprku identifies an essential requirement for IP3R function in the PPL1 and/or PPM3 DANs for flight bouts lasting upto ~300 s.
The two PPL1 clusters on each side of the brain consist of 12 pairs of neurons (Mao, 2009) and have been implicated in the maintenance of long flight bouts previously (Pathak et al., 2015). To further restrict flight modulating neuron/s in the PPL1 group we identified splitGAL4 strains that mark fewer PPL1 neuron/s and project to individual lobes of the Mushroom Body (MB; Figure 2—figure supplement 1C and Figure 2D; Aso et al., 2014a; Aso and Rubin, 2016). Amongst the identified splitGAL4 strains, flight deficits were observed by expression of IP3RDN in PPL1 DANs projecting to the MB lobes α’2α2, α3, γ1peduncle and γ2α′1 (MB504BGAL4), but not with PPL1 DANs projecting to the MB lobes α’2α2, α3, and Y1peduncle (MB438BGAL4; Figure 2E, Figure 2—source data 3), suggesting PPL1-γ2α′1DANs as the primary focus of IP3R function. Indeed, expression of ItprDN in PPL1-γ2α′1 DANs, marked by MB296BGAL4, resulted in significantly shorter flight bouts, when compared to Itpr+ expression in the same cells (Figure 2E). Expression of ItprDN in other PPL1 neurons failed to exhibit significant flight deficits (Figure 2—figure supplement 1D, Figure 2—source data 6). Thus PPL1-γ2α′1 DANs require IP3R function to sustain longer flight bouts. These data do not exclude a role for the IP3R in PPM3 DANs that are also marked by THD’GAL4 in the context of flight.
The IP3R is required in a late developmental window for adult flight
Shorter flight bouts in adults might arise either due to loss or change in properties of identified neurons during development. Alternately, the IP3R might acutely affect the function of these neurons during flight in adults. To distinguish between these possibilities, we employed the TARGET system (McGuire et al., 2003) for temporal control of IP3RDN expression in PPL1 and PPM3 DANs. TARGET uses a ubiquitously expressed temperature-sensitive repressor of GAL4 (Tub-GAL80ts) that allows GAL4 expression at 29o, but not at lower temperatures. Hence expression of a GAL4 driven UAS transgene can be controlled by changing incubation temperatures from 18o to 29o. THD’GAL4 driven ItprDN expression was restricted to the larval stages (data not shown), 0–48 hr after puparium formation (APF), 48–96 hr APF and for 0–3 days in adults. Flight deficits were observed upon expression of IP3RDN during pupal development and not when expressed in adults. In pupae the deficit was most prominent by expression of the ItprDN during the shorter window of 48–96 hr APF (Figure 3A, Figure 3—source data 1).
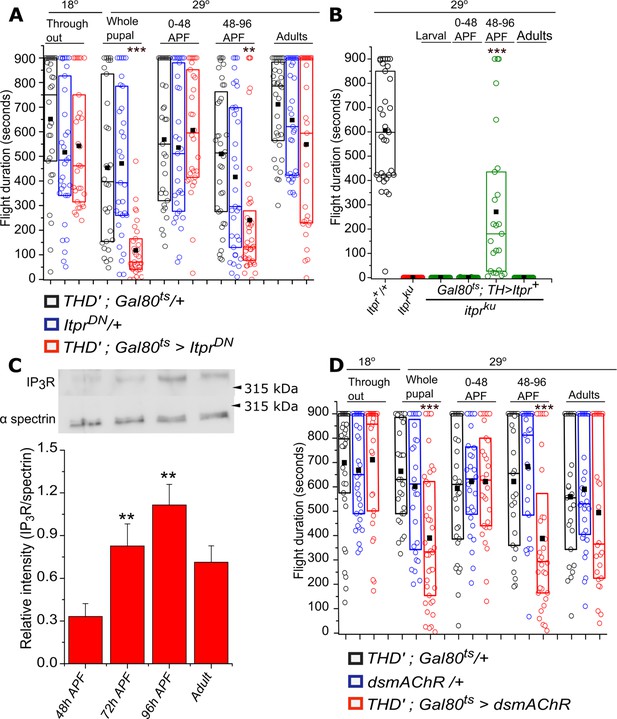
Adult flight phenotypes arise from late pupal expression of the IP3R and mAChR.
(A) Box plots of flight bout durations in adults after temporal expression of IP3RDN in THD’ neurons (THD’; TubGAL80ts > ItprDN) by transferring them to 29°C at the indicated stages of development. For adult expression, flies were transferred to 29°C immediately after eclosion and tested for flight after 3 days. (B) Box plots of flight bout durations after expressing the IP3R (Itpr+) in dopaminergic cells of itpr mutants at the indicated stages of development. For stage specific expression a TH; TubGAL80ts strain was used and the progeny transferred to 29°C at appropriate time developmental points. (C) Levels of the IP3R increase in late pupae between 72-96 hrs APF and plateau between 96hrs APF and adults (3 days). A representative western blot from lysates of dissected central nervous systems of Canton S probed with anti-IP3R and anti-spectrin is shown (top). Three independent lysates and blots were quantified (below). **p< 0.05, t-test. (D) Box plots with flight bout durations of adult flies after knockdown of the Muscarinic acetylcholine receptor (mAChR) in THD’ neurons (THD’;TubGAL80ts > dsmAChR) at the indicated stages of pupal development and in adults.Box plot symbols are as described in methods, n≥30, ***p< 0.005 *p< 0.05, n.s., not significant by Kruskal-Wallis test (for A and D) and n≥23, ***p< 0.005, at p < 0.05 by Mann-Whitney U test (for B). All comparisons for significance were with the control values for A and C, with itpr mutants for B.
-
Figure 3—source data 1
Flight duration of flies upon overexpression of IP3RDN at different stages of development.
- https://cdn.elifesciences.org/articles/62297/elife-62297-fig3-data1-v1.xlsx
-
Figure 3—source data 2
Flight duration of itpr mutant flies after overexpressing a wild type itpr transgene at different stages of development.
- https://cdn.elifesciences.org/articles/62297/elife-62297-fig3-data2-v1.xlsx
-
Figure 3—source data 3
Western blots of IP3R.
- https://cdn.elifesciences.org/articles/62297/elife-62297-fig3-data3-v1.pptx
-
Figure 3—source data 4
Flight duration of flies upon knockdown of mAChR in dopaminergic neurons at different stages of development.
- https://cdn.elifesciences.org/articles/62297/elife-62297-fig3-data4-v1.xlsx
-
Figure 3—source data 5
Flight duration of flies upon knockdown of mAChR in PPL1-γ2α′1 neurons.
- https://cdn.elifesciences.org/articles/62297/elife-62297-fig3-data5-v1.xlsx
Requirement for the IP3R during the 48–96 hr APF window was independently confirmed by measuring flight after temporal expression of Itpr+ in dopaminergic neurons of itprku (Figure 3B, Figure 3—source data 2). Flight in itprku mutant animals was rescued to a significant extent by Itpr+ expression from 48 to 96 hr APF (277 ± 60.3 s) but not when expressed before and after. Interestingly, post-48 hr APF is also the time period during which levels of the IP3R are upregulated in the pupal brain (Figure 3C, Figure 3—source data 3). Thus, expression from the ItprDN encoding transgene during the 48–96 hr interval presumably results in the formation of a majority of inactive IP3R tetramers due to the presence of at least one IP3RDN monomer. The cellular and physiological consequences of inactive IP3Rs in pupal, and subsequently adult brains, was investigated next.
It is known that specification of central dopaminergic neurons is complete in late larval brains (Hartenstein et al., 2017) The number of PPL1 DANs was no different between controls and in flies expressing ItprDN in either TH-D or MB296B marked neurons (Figure 3—figure supplement 1A). This finding agrees with our observation that larval expression of ItprDN had no effect on adult flight. Moreover, projections from MB296B to the γ2α′1 MB lobes also appeared unchanged upon expression of ItprDN (Figure 3—figure supplement 1B).
To understand the nature of signaling through the IP3R, required during pupal development, we tested flight after knockdown of an IP3/Ca2+ linked GPCR, the muscarinic acetylcholine receptor (mAChR). From a previous study, it is known that neuronal expression of the mAChR during pupal development is required for adult flight (Agrawal et al., 2013). Adult flight bouts were significantly shorter upon stage specific knock-down (48–96 hr APF) of the mAChR in TH-D’ neurons with an RNAi under temporal control of the TARGET system (Figure 3D, Figure 3—source data 4). Further knockdown of mAChR in PPL1- γ2α′1 DANs also manifested mild flight defects (Figure 3—figure supplement 1C, Figure 3—source data 5). These data suggest that the mAChR and IP3-mediated Ca2+ release are required during pupal maturation of a central brain circuit that functions for the maintenance of long flight bouts. Alternately, the pupal requirement may arise from the fact that both the IP3R (Figure 3A,B) and the mAChR (Figure 3D) are synthesized in late pupal neurons, carried over to adult neurons where they have a slow turnover, and function during acute flight. Taken together our data support the idea that acetylcholine, a neurotransmitter, activates the mAChR on PPL1 DANs of late pupae and/or adults to stimulate Ca2+ release through the IP3R. Cellular changes arising from loss of IP3 mediated Ca2+ release were investigated next.
Synaptic vesicle release and IP3R are both required for the function of PPL1-γ2α′1 DANs
The 48–96 hr time window of pupal development is when adult neural circuits begin to mature with the formation of synapses, some of which are eliminated whilst others are strengthened (Akin et al., 2019; Consoulas et al., 2002; Zhang et al., 2016). Synapse strengthening occurs when pre-synaptic neurotransmitter release leads to post-synaptic excitation/inhibition (Andreae and Burrone, 2018; Baines, 2003; Baines et al., 2001; Pang et al., 2010). We hypothesized that IP3-mediated Ca2+ release might modulate synaptic activity and hence lead to strengthening of synapses between THD’ DANs and their post-synaptic partners during pupal development. To test this idea, the requirement for synaptic vesicle recycling in THD’ and PPL1-γ2α′1 DANs was investigated through pupal development. A transgene encoding a temperature-sensitive mutant of Dynamin, Shibirets (Kitamoto, 2001), that prevents synaptic vesicle recycling at 29°C and hence blocks neurotransmitter release, was expressed during pupal development, by transfer to 29°C at the appropriate time interval. Loss of synaptic vesicle recycling in pupae resulted in significantly shorter flight bout durations of 419.9 ± 50.2 s (THD’) and 411.5 ± 57 s (MB296B) as compared to controls (Figure 4A, Figure 4—source data 1 and Figure 4—figure supplement 1A, Figure 4—source data 4). The requirement for synaptic vesicle release was further restricted to 48–96 hr APF consistent with the requirement of IP3R at the same time interval (Figure 3A,B). These data suggest that THD’ marked dopaminergic neurons require both synaptic vesicle recycling and IP3-mediated Ca2+ release during late pupal development for their adult function.
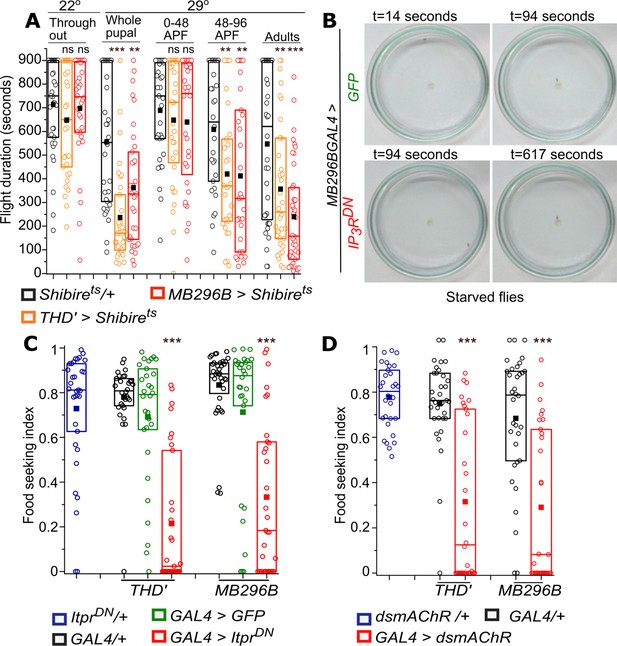
Synaptic vesicle recycling and IP3R function are required in PPL1 dopaminergic neurons for adult flight and feeding.
(A) Quantification of flight deficits observed upon blocking synaptic vesicle recycling by expression of a temperature-sensitive Dynamin mutant, Shibirets in THD’ and MB296B cells. n≥30, ***p< 0.005, **p< 0.05 by Mann Whitney U test. (B) Food seeking by hungry flies is significantly diminished upon expression of IP3RDN in PPL1- γ2α′1 DANs (MB296B). Snapshots at the indicated time points from videos of starved male flies of the indicated genotype seeking a drop of yeast placed in the centre of a petriplate. (C) Quantification of food seeking behaviour in starved males of the indicated genotypes. Expression of IP3RDN in THD’ and MB296B cells reduced food seeking behaviour to a significant extent (red). Expression of GFP did not affect the behaviour (green). n≥30, ***p< 0.005 by Kruskal-Wallis test. (D) Quantification of food-seeking behaviour in starved males of the indicated genotypes. Knockdown of mAChR in THD’ and MB296B cells reduced food-seeking behaviour to a significant extent (red), n≥30, ***p< 0.005 at by Kruskal-Wallis test.
-
Figure 4—source data 1
Flight duration of flies upon expression of Shibirets in dopaminergic neurons at different developmental stages.
- https://cdn.elifesciences.org/articles/62297/elife-62297-fig4-data1-v1.xlsx
-
Figure 4—source data 2
Food seeking index of starved male flies after overexpression of IP3RDN in dopaminergic neurons.
- https://cdn.elifesciences.org/articles/62297/elife-62297-fig4-data2-v1.xlsx
-
Figure 4—source data 3
Food seeking index of starved male flies upon knockdown of mAChR in dopaminergic neurons.
- https://cdn.elifesciences.org/articles/62297/elife-62297-fig4-data3-v1.xlsx
-
Figure 4—source data 4
Flight duration of flies upon expression of Shibirets in dopaminergic neurons, grown at 25°C.
- https://cdn.elifesciences.org/articles/62297/elife-62297-fig4-data4-v1.xlsx
-
Figure 4—source data 5
Food seeking index of fed male flies after overexpression of IP3RDN in dopaminergic neurons.
- https://cdn.elifesciences.org/articles/62297/elife-62297-fig4-data5-v1.xlsx
-
Figure 4—source data 6
Food seeking index of fed male flies upon knockdown of mAChR in dopaminergic neurons.
- https://cdn.elifesciences.org/articles/62297/elife-62297-fig4-data6-v1.xlsx
The acute requirement for synaptic vesicle recycling in adult PPL1 (THD’) and PPL1-γ2α′1 subset (MB296B) of DANs for maintenance of flight bouts was tested next. A previous report shows that synaptic vesicle recycling is required in PPL1 DANs marked by THD1GAL4 during active flight (Ravi et al., 2018). We tested if synaptic vesicle release is also required in THD’ and MB296B marked PPL1 DANs in adults. Importantly, flight bout durations were reduced significantly after acute inactivation (5 min) of synaptic vesicle recycling in PPL1 DANs (THD’) and the pair of PPL1-γ2α′1 subset (MB296B) DANs in adults, supporting a requirement for neurotransmitter release from these neurons during flight (Figure 4A).
Impaired synaptic vesicle release from adult PPL1-γ2α′1 DANs, by expression of Shibirets, also reduces the ability of starved flies to identify a food source rapidly (Tsao et al., 2018). To understand if the IP3R affects the function of PPL1-γ2α′1 DANs in more than one behavioural context, we tested food-seeking behaviour of starved flies expressing ItprDN in PPL1 (THD’) and PPL1-γ2α′1 subset (MB296B) DANs. The food seeking index of hungry flies with IP3RDN was reduced significantly as compared to controls (Figure 4B and C, Figure 4—source data 2, Figure 4—video 1 and Figure 4—video 2). Food-seeking behaviour of fed flies of all genotypes tested appeared similar (Figure 4—figure supplement 1B, Figure 4—source data 5).
Next, we tested if knockdown of mAChR, the identified flight regulating GPCR (Figure 3 and Figure 3—figure supplement 1C) that couples to IP3/Ca2+ signaling, also affected food seeking in starved flies. Knockdown of mAChR in either PPL1 (THD’) or PPL1-γ2α′1 decreased the ability of starved males to find a yeast drop (Figure 4D, Figure 4—source data 3), while fed flies were similar to genetic controls (Figure 4—figure supplement 1C, Figure 4—source data 6). Thus cholinergic stimulation of IP3/Ca2+ in PPL1-γ2α′1 DANs reduces the motivation for longer flight bouts as well as the motivation to search for food.
Taken together these data demonstrate a requirement for both synaptic vesicle recycling and IP3-mediated Ca2+ release in the modulation of behaviour by PPL1-γ2α′1 DANs. Furthermore, they suggest that the IP3R might regulate synaptic function in PPL1-γ2α′1 DANs.
The IP3R affects neurotransmitter release from adult dopaminergic neurons
Decreased synaptic activity upon IP3RDN expression might be a consequence of a reduction in synapse number during circuit maturation in pupae. Therefore, a pre-synaptic marker, synaptotagmin tagged to GFP (syt.eGFP; Zhang et al., 2002), that localises to synaptic vesicles was expressed in THD’ marked neurons in the absence and presence of IP3RDN. Fluorescence of Syt.eGFP in the MB lobes was no different between control brains and in presence of the IP3RDN (Figure 5A), suggesting that synapse numbers were unchanged by expression of IP3RDN. However, Syt.eGFP does not necessarily measure functional MB synapses. Acetylcholine and mAchR driven IP3R – Ca2+ signals in dopaminergic neurons might thus effect formation of functional MB synapses during circuit maturation in pupae. Loss of flight deficits observed upon inactivating synaptic vesicle recycling in late pupae supports this idea (Figure 4A).
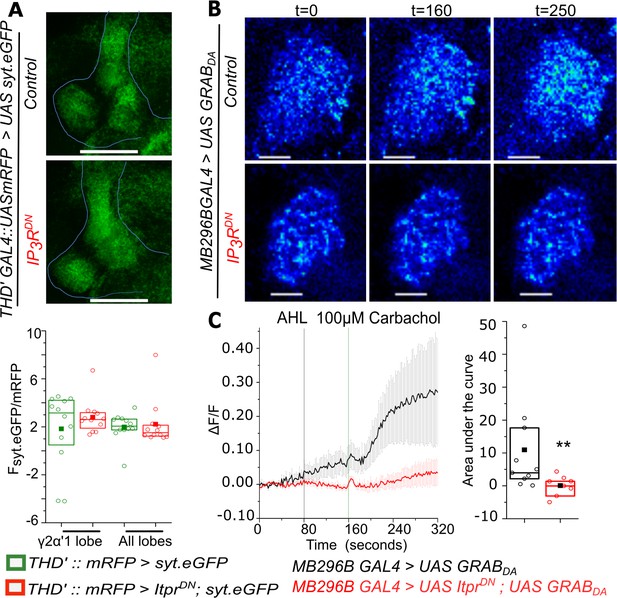
Carbachol-stimulated Dopamine release at the axonal terminals of PPL1-γ2α′1 neurons is attenuated by expression of IP3RDN.
(A) Representative images of a presynaptic marker synaptotagmin GFP (syt.eGFP) in lobes of the Mushroom body (top). Quantification of syt.eGFP fluorescence normalized to mRFP is shown below in the indicated MB regions marked by THD’GAL4. N = 6 brains, n.s. not significant at p < 0.05 by two tailed Mann-Whitney U test. Scale bars indicate 50 μm (B) Carbachol stimulated dopamine release at PPL1- γ2α′1 termini visualized by changes in GRABDA fluorescence in a representative mushroom body lobe from brains of the indicated genotypes. Images were acquired at 2s per frame. Brighter fluorescence denotes increase in Dopamine. Scale bars = 10 μm (C) Average traces (± SEM) of normalized GRABDA fluorescence responses (ΔF/F) from the right lobe of each brain upon addition of Carbachol (Green line) (panel on the left). Images were acquired at 2s per frame; Quantification of area under the curve was from the point of stimulation at 160s and up to 250 s. Box plots and symbols are as described in Figure 2A. MB296B GAL4 > UAS GRABDA N = 10 cells from 10 brains; MB296B GAL4 > UAS ItprDN; UAS GRABDA N = 8 cells from 8 brains. **p< 0.05, Mann-Whitney U test.
-
Figure 5—source data 1
GRABDA fluorescence (ΔF/F) traces from axonal termini of MB296BGAL4 marked DANs in individual brains (Black -MB296B GAL4>UAS GRABDA; Red-MB296B GAL4>UAS ItprDN; UAS GRABDA).
- https://cdn.elifesciences.org/articles/62297/elife-62297-fig5-data1-v1.pptx
Flight deficits also occur upon acute inactivation of synaptic vesicle recycling in adults (Figure 4A). Therefore, neurotransmitter release from PPL1-γ2α′1 (MB296B) DANs in response to stimulation of the IP3R was investigated next in adults. Presence of the mAChR was confirmed on PPL1-γ2α′1 DANs in adults by measuring Ca2+ release upon stimulation with the mAChR agonist Carbachol. Changes in GCaMP6m fluorescence in MB296B marked neurons post-Carbachol stimulation are shown in Figure 5—figure supplement 1A. The ability of Carbachol to stimulate dopamine release from MB296B marked neurons in the γ2α′1 MB-lobe was measured next. For this purpose, a recently designed fluorescent sensor for dopamine, GRABDA (G-protein-coupled receptor-activation based DA sensor; Sun et al., 2018) was expressed in MB296B neurons followed by stimulation with Carbachol. GRABDA consists of a dopamine receptor linked to cpEGFP such that its fluorescence increases upon binding of dopamine. Thus, a qualitative measure of dopamine release at the synaptic cleft is the change in GRABDA fluorescence when recorded in the γ2α′1 MB lobe, the site of PPL1-γ2α′1 synapses (Figure 5B and C and Figure 5—source data 1). Control brains, with detectable changes in GRABDA fluorescence (ΔF/F) above an arbitrary value of 0.05 within 140 s of Carbachol stimulation, were classified as responders. Responding brains decreased from 77% (10/13 brains) in controls to 47% (8/17 brains) upon expression of IP3RDN. Moreover, GRABDA fluorescence arising from dopamine release was significantly muted amongst responders expressing the IP3RDN as compared to responders from control brains (Figure 5C and Figure 5—figure supplement 1B). Thus, expression of IP3RDN in MB296B DANs reduced the synaptic release of dopamine at the γ2α′1 lobe. Interestingly, increase in GRABDA fluorescence at the synaptic terminals in the MB was faster (Figure 5C) than the carbachol-stimulated change in GCaMP fluorescence measured in the cell body as measured by time taken to reach the peak response (Figure 5—figure supplement 1A). Perhaps, there exist a greater number of mAChRs near the synapse than on the soma of MB296B DANs. For example, mAChRs are also present on the processes of Kenyon cells in the mushroom body (Bielopolski et al., 2019; Kondo et al., 2020) and form a tripartite synapse with dendrites from MB output neurons (MB-ONs) and axonal processes of MB296B DANs. Although requirement for the IP3R and the mAChR was restricted to 48–96 hr APF, these data suggest that the neurotransmitter acetylcholine stimulates dopamine release in adult DANs through mAChR-IP3R signaling. The lack of flight deficits by adult-specific expression of IP3RDN (Figure 3A) and knockdown of mAChR (Figure 3D) is probably due to perdurance of the respective proteins from late pupae through to adults. It remains possible that reduced strength of MB synapses, by pupal expression of IP3RDN and concomitant inhibition of synaptic vesicle release during circuit maturation, also contribute to adult flight deficits.
The IP3R helps maintain membrane excitability of PPL1-γ2α′1neurons
In addition to the neuromodulatory input of acetylcholine, the PPL1-γ2α′1 DANs are likely to receive direct excitatory inputs. Therefore, next we investigated if loss of IP3/Ca2+ signals alter the essential properties of neuronal excitability of MB296B neurons. Stimulation by activation of an optogenetic tool, CsChrimson (Klapoetke et al., 2014) was followed by measuring changes in fluorescence of the Ca2+ sensor GCaMP in ex-vivo brain preparations. In neurons expressing IP3RDN and GCaMP optogenetic stimulation of CsChrimsom did not evince a significant Ca2+ response either during or after the red light stimulus, as compared with the control genotype expressing RFP and GCaMP (Figure 6A,B and Figure 6—source data 1). In agreement with recent findings, some MB296B neurons exhibit high basal activity even in the absence of optogenetic stimulation (Figure 6—figure supplement 1; Siju et al., 2020). Similar results were obtained by KCl - evoked depolarisation in the presence of 2 μM Tetrodotoxin (TTX) (Figure 6—figure supplement 1A,B, Figure 6—source data 3). TTX was added so as to prevent excitation by synaptic inputs from other neurons upon KCl addition. These data suggest that neurons with IP3RDN fail to respond to a depolarising stimulus. This idea was tested directly by measuring the change in membrane potential in response to depolarisation, with a genetically encoded fluorescent voltage indicator, Arclight (Cao et al., 2013). There was an instant decline in Arclight fluorescence in control cells, while cells with IP3RDN showed almost no change in fluorescence after KCl-mediated depolarisation (Figure 6C,D, Figure 6—source data 2). Taken together, these observations confirm that signaling through the IP3R is also required for maintaining excitability of central neuromodulatory dopaminergic neurons.
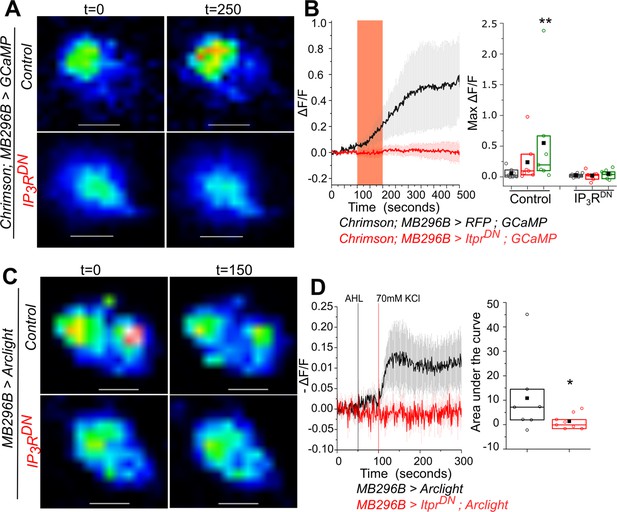
Optimal excitability of PPL1- γ2α′1 dopaminergic neurons requires the IP3R.
(A) Optogenetic activation of PPL1- γ2α′1 DANs, with the red light activated Channelrhodopsin variant Chrimson, is attenuated by expression of IP3RDN. Representative images of MB296BGAL4 marked DANs of the indicated genotypes are shown with changes in GCaMP6m fluorescence before (t=0) and after (t=250s) a 100s pulse of red light (red bar). Images were acquired at 2s per frame. Warmer colors denote increase in [Ca2+]. Scale bar = 5 μm. (B) Average traces (± SEM) of normalized changes in GCaMP6m fluorescence (ΔF/F) in MB296BGAL4 marked DANs after activation by Chrimson (left); Quantification of areas under the curve are shown for before stimulation (0-100 sec, gray), during stimulation (100-200s, red) and after stimulation (200-500s, green); (right). Box plots and symbols are as described in Figure 2A. MB296B GAL4 > UAS RFP; UAS GCaMP, N = 7 cells from 7 brains; MB296B GAL4 > UAS ItprDN; UAS GCaMP, N = 8 cells from 8 brains **p< 0.05, Mann-Whitney U test. (C) Changes in membrane potential upon addition of KCl, visualized by expression of the voltage sensor Arclight. Representative images of Arclight responses in MB296BGAL4 marked DANs are shown from the indicated genotypes and time points. Images were acquired at 1s per frame; Scale bar = 5 μm. Average traces (± SEM) of normalized changes in Arclight fluorescence (-ΔF/F) in MB296BGAL4 marked DANs after addition of KCl (left). Images were acquired at 1s per frame; Quantification of area under the curve is from the point of stimulation at 100s up to 200s (right). Box plots and symbols are as described in Figure 2A. MB296B GAL4 > UAS Arclight N = 7 cells from 7 brains; MB296B GAL4 > UAS ItprDN; UAS Arclight N = 8 cells from 8 brains *p< 0.1, Mann-Whitney U test.
-
Figure 6—source data 1
GCaMP6m fluorescence (ΔF/F) traces inMB296BGAL4 marked DANs after activation by Chrimson in individual brains (Black -MB296B GAL4>UAS RFP; UAS GCaMP; Red-MB296B GAL4>UAS ItprDN; UAS GCaMP).
- https://cdn.elifesciences.org/articles/62297/elife-62297-fig6-data1-v1.pptx
-
Figure 6—source data 2
Arclight fluorescence (ΔF/F) traces in MB296BGAL4 marked DANs after KCl induced depolarisation in individual brains (Black -MB296B GAL4>UAS Arclight; Red-MB296B GAL4>UAS ItprDN; UAS Arclight).
- https://cdn.elifesciences.org/articles/62297/elife-62297-fig6-data2-v1.pptx
-
Figure 6—source data 3
GCaMP fluorescence (ΔF/F) traces inMB296BGAL4marked DANs after KCl induced depolarisation in individual brains (Black -MB296B GAL4>UAS GCaMP; Red-MB296B GAL4>UASItprDN; UAS GCaMP).
- https://cdn.elifesciences.org/articles/62297/elife-62297-fig6-data3-v1.pptx
Discussion
An inducible IP3RDN construct developed and used in this study allowed us to perform stage and cell-specific attenuation of IP3R mediated calcium signaling in vivo. Consistent with well characterised phenotypes of IP3R mutants (Banerjee et al., 2004), neuronal expression of IP3RDN affected flight. Spatiotemporal studies identified a requirement for the IP3R in a small subset of central dopaminergic neurons for maintenance of adult flight bouts as well as in the food-seeking behaviour of hungry flies. Inhibition of synaptic release in the identified dopaminergic subset also reduced the duration of flight bouts. Dopamine release at synapses in the MB γ2α′1 lobe was significantly attenuated in adults expressing the IP3RDN (carried over from late pupae). These animals in addition exhibit reduced membrane excitability. Intracellular Ca2+ signaling through the IP3R is thus required to ensure optimal neuronal excitability and synaptic function in specific central dopaminergic neurons that appear to drive the motivation for both longer flight bouts and the search for food in a hungry fly (Figure 7).
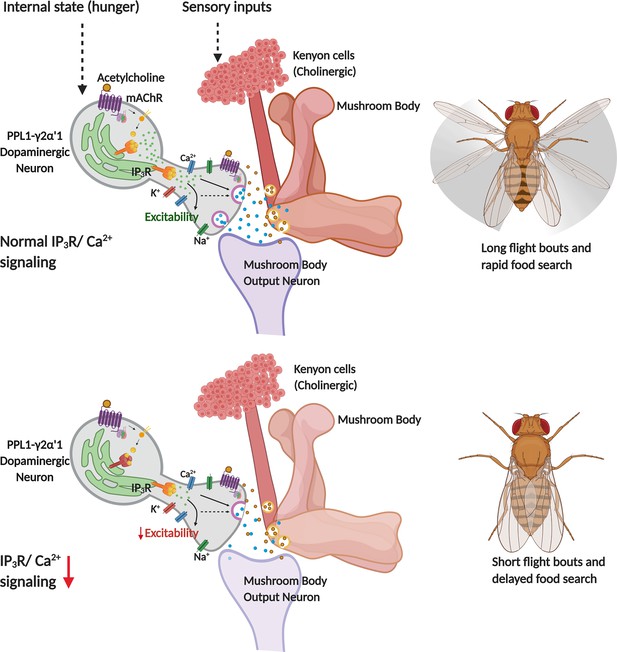
Schematic showing neuronal properties regulated by IP3/Ca2+ signals in central dopaminergic neurons for flight and food search behaviour in Drosophila melanogaster.
The IP3R and synaptic release in pupae and adults
Temporal expression of IP3RDN demonstrated a requirement during circuit maturation in late pupae but not in adults. Taken together with flight deficits that arise as a consequence of pupal inactivation of vesicle recycling (Figure 4A), these data suggest that Ca2+ release through the IP3R stimulates neurotransmitter release required for maturation of PPL1- γ2α′1 synapses with MBONs (Aso et al., 2014b; Berry et al., 2018) and possibly KCs (Cervantes-Sandoval et al., 2017) in late pupae. However, these data do not rule out an acute function for the IP3R in adult dopaminergic neurons because functional wild-type tetramers of the IP3R assembled during pupal development very likely mask the effect of adult specific expression of IP3RDN. The presence of perdurant IP3R proteins in adults may be addressed in future by pupal expression of tagged proteins. Because short flight bouts are also observed upon acute inhibition of vesicle recycling in adults our data support a model where mAChR stimulated ER-Ca2+ signals through the IP3R regulate synaptic release of dopamine from PPL1- γ2α′1 DANs during circuit maturation and during adult flight. Both direct and indirect effects of ER-Ca2+ on synaptic vesicle release have been observed in vertebrates (Gomez et al., 2020; Rossi et al., 2008; Sharma and Vijayaraghavan, 2003) and Drosophila (James et al., 2019; Klose et al., 2010; Richhariya et al., 2018; Shakiryanova et al., 2011). Further studies are required to identify the molecular mechanism by which ER-Ca2+ signals regulate dopamine release in PPL1- γ2α′1 DANs.
Intracellular Ca2+ signaling and neuronal excitability
Genetic manipulations that target intracellular calcium signaling are known to affect the intrinsic excitability of neurons. For example, Purkinje neurons in mice with cell-specific knockout of the ER-Ca2+ sensor Stim1, that functions downstream of mGluR1, exhibit a decreased frequency of firing (Ryu et al., 2017). In Drosophila FMRFa Receptor mediated calcium signaling modulates the excitability of PPL1 dopaminergic neurons through CAMKII (Ravi et al., 2018). Reduced excitability of dopaminergic neurons expressing the IP3RDN transgene (Figure 6 and Figure 6—figure supplement 1) further supports a role for intracellular calcium signals in setting the threshold of membrane excitability in response to neuromodulatory signals, such as acetylcholine (this study), the FMRFa neuropeptide (Ravi et al., 2018), and glutamate (Ryu et al., 2017).
The cellular mechanism(s) by which IP3/Ca2+ signals regulate neuronal excitability probably vary among different classes of neurons. In addition to the direct activation of Ca2+-dependant enzymes such as CamKII, previous reports have shown that knock-down of the IP3R in Drosophila larval neurons alters their expression profile and specifically affects the expression of several membrane localised ion-channels (Jayakumar et al., 2018). Reduced translation of proteins due to IP3R knockdown has also been demonstrated in peptidergic neurons (Megha and Hasan, 2017). Changes in membrane excitability could thus derive from direct regulation of ion channels by Ca2+ and Ca2+ dependant enzymes as well as an altered density of specific ion channels. Moreover, Ca2+ release through the IP3R regulates mitochondrial Ca2+ entry (Figure 1E). Expression of IP3RDN might thus impact neuronal firing and synaptic release by changes in cellular bioenergetics (Cárdenas et al., 2010; Chouhan et al., 2012) .
PPL1- γ2α′1 DANs, flight and the search for food
Although insect flight is an innate behaviour the persistence of flight requires motivation presumably driven by neuromodulatory mechanisms and circuits in the central brain, that need to intersect with hard-wired flight circuits in the ventral ganglion. A role for the PPL1 cluster of dopaminergic neurons in modulating flight and longer flight bouts has been reported earlier (Pathak et al., 2015; Ravi et al., 2018). However, amongst the PPL1 cluster this is the first report identifying the two PPL1- γ2α′1 DANs as required for maintenance of flight. The PPL1- γ2α′1 DANs and their downstream Mushroom body output neuron (MBON- γ2α′1) also encode the state of hunger (Tsao et al., 2018), formation and consolidation of appetitive memory (Berry et al., 2018; Felsenberg et al., 2017; Yamazaki et al., 2018), fat storage (Al-Anzi and Zinn, 2018), and sleep (Aso et al., 2014b; Sitaraman et al., 2015). These studies identified the importance of modulated dopamine release as a motivational cue wherein dopamine release from PPL1- γ2α′1 DANs increased in starved flies (Tsao et al., 2018) and inhibited the activity of a cholinergic output neuron from the Mushroom Body (MBON- γ2α′1; Tsao et al., 2018). The MBON- γ2α′1 projects to another set of central DANs, the PAM neurons (Felsenberg et al., 2017), identified as flight promoting in a previous study (Manjila et al., 2019), as well as the fan shaped body (FSB) and the lateral accessory lobe (LAL; Scaplen et al., 2020). This leads to the hypothesis that inputs regarding the internal state reach the PPL1- γ2α′1 DANs through mAChR, amongst other GPCR (see below), driven IP3/Ca2+ signals to modulate dopamine release at the PPL1- γ2α′1 > MBON- γ2α′1 synapse, and the extent of dopamine release changes the output strength of MBON - γ2α′1. In addition, continuous sensory inputs are essential for flight and these may reach PPL1- γ2α′1/MBON - γ2α′1 synapses through cholinergic Kenyon cells (Cervantes-Sandoval et al., 2017). In this context, carbachol-stimulated Dopamine release at the MB-γ2α′1 lobe may in part be post-synaptic to acetylcholine release from the Kenyon cells. Altered MBON- γ2α′1 outputs might then regulate flight through their functional connections with the PAM-DANs, the LAL neurons and the FSB (Scaplen et al., 2020). Amongst these the LAL neurons signal to descending neurons (Namiki et al., 2018; Namiki and Kanzaki, 2016) that presumably connect with the flight circuit in the ventral ganglion. This idea is broadly supported by the observation that LAL neurons in locusts exhibit flight correlated activity changes (Homberg, 1994). The FSB processes visual inputs for flight navigation (Weir and Dickinson, 2015) and functions during turning behaviour (Shiozaki et al., 2020). In cockroaches, activation of the FSB induces turns (Guo and Ritzmann, 2013).
Prolonged flight bouts could serve to identify new sources of food for a hungry fly but at the same time they are energy intensive and very likely require multisensory integration with the inner state for continuous motivation. A recent review (Lee and Wu, 2020) describes a framework of ‘homeostatic motivation ‘as an integral part of such motivated behaviour, consisting of neural circuits with a ‘sensor, integrator and effector’. The PPL1- γ2α′1 DANs receive inputs for assessing the internal state through multiple GPCRs including certain neuropeptide receptors such as sNPFR, Insulin receptor and AstA receptor and a serotonin receptor 5HT1B (Albin et al., 2015; Hergarden et al., 2012; Root et al., 2011; Tsao et al., 2018). Each of these signals appear to integrate hunger with food seeking behaviour (Tsao et al., 2018). We propose that modulated activity in the PPL1- γ2α′1 DANs serves as a ‘sensor’ followed by inhibition of MBON - γ2α′1, that forms an important multisensory ‘integrating component’ for control of downstream ‘effector’ circuits. Appropriate MBON- γ2α′1 outputs are required to maintain long flight bouts, similar to the maintenance of wakefulness (Aso et al., 2014b; Sitaraman et al., 2015) and increase in the search for food when hungry (Tsao et al., 2018). The integration of external cues that stimulate flight with the internal states of hunger and wakefulness very likely serve important functions of survival in the wild.
Materials and methods
Reagent type (species) or resource | Designation | Source or reference | Identifiers | Additional information |
---|---|---|---|---|
Genetic reagent (D. melanogaster) | nSybGAL4 | Bloomington Drosophila Stock Center | RRID:BDSC_51635 | |
Genetic reagent (D. melanogaster) | UAS GCaMP6m | Bloomington Drosophila Stock Center | RRID:BDSC_42750 | |
Genetic reagent (D. melanogaster) | UAS GCaMP6m | Bloomington Drosophila Stock Center | RRID:BDSC_42748 | |
Genetic reagent (D. melanogaster) | UAS Arclight | Bloomington Drosophila Stock Center | RRID:BDSC_51056 | |
Genetic reagent (D. melanogaster) | UAS mCD8GFP | Bloomington Drosophila Stock Center | RRID:BDSC_5130 | |
Genetic reagent (D. melanogaster) | UAS Dicer | Bloomington Drosophila Stock Center | RRID:BDSC_24648 | |
Genetic reagent (D. melanogaster) | UAS Chrimson | Bloomington Drosophila Stock Center | RRID:BDSC_55137 | |
Genetic reagent (D. melanogaster) | UAS syt.eGFP | Bloomington Drosophila Stock Center | RRID:BDSC_6926 | |
Genetic reagent(D. melanogaster) | UAS RFP | Bloomington Drosophila Stock Center | RRID:BDSC_32218 | |
Genetic reagent (D. melanogaster) | MB058BGAL4 | Bloomington Drosophila Stock Center | RRID:BDSC_68278 | |
Genetic reagent (D. melanogaster) | MB296BGAL4 | Bloomington Drosophila Stock Center | RRID:BDSC_63308 | |
Genetic reagent (D. melanogaster) | MB304BGAL4 | Bloomington Drosophila Stock Center | RRID:BDSC_68367 | |
Genetic reagent (D. melanogaster) | MB320CGAL4 | Bloomington Drosophila Stock Center | RRID:BDSC_68253 | |
Genetic reagent (D. melanogaster) | MB630BGAL4 | Bloomington Drosophila Stock Center | RRID:BDSC_68334 | |
Genetic reagent (D. melanogaster) | MB438BGAL4 | Bloomington Drosophila Stock Center | RRID:BDSC_68326 | |
Genetic reagent (D. melanogaster) | MB504BGAL4 | Bloomington Drosophila Stock Center | RRID:BDSC_68329 | |
Genetic reagent (D. melanogaster) | UAS mAChR RNAi (dsmAChR) | Vienna Drosophila Resource Center RRID:SCR_013805 | VDRC_101407 | |
Genetic reagent (D. melanogaster) | UAS itprRNAi (dsitpr) | National Institute of Genetics | NIG_1063 R-2 | |
Genetic reagent (D. melanogaster) | UAS Itpr+ | Venkatesh et al., 2001 | RRID:BBSC_30742 | |
Genetic reagent (D. melanogaster) | THGAL4 | Friggi-Grelin et al., 2003 (DOI:10.1002/neu.10185) | Gift from Serge Birman (CNRS, ESPCI Paris Tech, France) | |
Genetic reagent (D. melanogaster) | THD’ GAL4 | Liu et al., 2012 (DOI:10.1016/j.cub.2012.09.008) | Gift from Mark N Wu (Johns Hopkins University, Baltimore) | |
Genetic reagent (D. melanogaster) | THD1 GAL4 | Liu et al., 2012 (DOI:10.1016/j.cub.2012.09.008) | Gift from Mark N Wu (Johns Hopkins University, Baltimore) | |
Genetic reagent (D. melanogaster) | THC’ GAL4 | Liu et al., 2012 (DOI:10.1016/j.cub.2012.09.008) | Gift from Mark N Wu (Johns Hopkins University, Baltimore) | |
Genetic reagent (D. melanogaster) | UAS GRABDA | Sun et al., 2018 (DOI:10.1016/j.cell.2018.06.042) | Gift from Yulong Li (Peking University School of Life Sciences, Beijing, China) | |
Genetic reagent (D. melanogaster) | UAS Shibirets | Kitamoto, 2001 (DOI:10.1002/neu.1018) | Gift from Toshihiro Kitamoto (University of Iowa, Carver College of Medicine, Iowa) | |
Genetic reagent (D. melanogaster) | vGlutVGN6341GAL4 | Syed et al., 2016 (DOI:10.7554/eLife.11572) | Gift from K. Vijayraghavan (NCBS, India) | |
Genetic reagent (D. melanogaster) | UAS mitoGCaMP | Lutas et al., 2012 (DOI:10.1534/g3.111.001586) | Gift from Fumiko Kawasaki (Pennsylvania State University, Pennsylvania) | |
Genetic reagent (D. melanogaster) | UAS TubGAL80ts | Pathak et al., 2015 (DOI:10.1523/JNEUROSCI.1680–15.2015) | Generated by Albert Chiang, NCBS, Bangalore, India | |
Genetic reagent (D. melanogaster) | UAS ItprDN | This paper | Transgenic Drosophila with a Dominant negative Drosophila IP3R cDNA | |
Genetic reagent (D. melanogaster) | itpr gene mutant (itprug3) | Joshi et al., 2004 (DOI:10.1534/genetics.166.1.225) | RRID:BDSC_30738 | |
Genetic reagent (D. melanogaster) | itpr gene mutant (itprka1091) | Joshi et al., 2004 (DOI:10.1534/genetics.166.1.225) | RRID:BDSC_30739 | |
Antibody | Rabbit anti GFP (polyclonal) | Life Technologies, Thermo Fisher | RRID:AB_221570,Cat # A-6455 | IHC: 1:10,000 |
Antibody | Mouse anti-bruchpilot (monoclonal) | Wagh et al., 2006 (DOI:10.1016/j.neuron.2006.02.008) | Gift from Eric Buchner, University of Wuerzburg, Germany (dilution 1:150) | |
Antibody | Anti-mouse Alexa Fluor 568 (polyclonal) | Life Technologies, ThermoFisher Scientific | Cat# A-11004, RRID:AB_2534072 | IHC: 1:400 |
Antibody | Anti-rabbit Alexa Fluor 488 (polyclonal) | Life Technologies, ThermoFisher Scientific | Cat# A-11008, RRID:AB_143165 | IHC: 1:400 |
Antibody | Rabbit anti-IP3R (polyclonal) | Agrawal et al., 2009 (DOI:10.1371/journal.pone.0006652) | IB-9075 | WB: 1:300; Gift from Ilya Bezprozvanny (UT South Western, USA). |
Antibody | Mouse anti-spectrin (monoclonal) | Developmental Studies Hybridoma Bank | Cat# 3A9 (323 or M10-2), RRID:AB_528473 | WB: 1:50 |
Antibody | Anti-mouse HRP (polyclonal) | Cell Signaling Technology | Cat# 7076, RRID:AB_330924 | WB: 1:3000 |
Antibody | Anti-rabbit HRP (polyclonal) | ThermoFisher Scientific | Cat#32260, RRID:AB_1965959 | WB: 1:5000 |
Chemical compound, drug | Low melt Agar | Invitrogen | Cat# 16520–050 | |
Chemical compound, drug | Carbachol | Sigma Aldrich | Cat# C4382 | |
Chemical compound, drug | All trans Retinal | Sigma Aldrich | Cat# R2500 | |
Chemical compound, drug | EcoR1 | New England Biolabs | Cat# R0101S | Restriction enzyme |
Chemical compound, drug | Xho1 | New England Biolabs | Cat# R0146S | Restriction enzyme |
Chemical compound, drug | AatII | New England Biolabs | Cat# R0117S | Restriction enzyme |
Chemical compound, drug | Eag1 | New England Biolabs | Cat# R0505S | Restriction enzyme |
Strain, strain background (Escherichia coli) | Sure Competent cells | Stratagene, Agilent Technologies | Cat# 200238 | Maintained in the lab |
Commercial assay or kit | Quick Ligation Kit | New England Biolabs | Cat# M2200S | |
Commercial assay or kit | WesternBright ECL kit | Advansta | Cat# K-12045-D20 | |
Commercial assay or kit | QuikChange II XL Site-Directed Mutagenesis Kit | Agilent | Cat# 200522 | |
Software, algorithm | Origin 8 | Origin lab | RRID:SCR_014212 | |
Software, algorithm | Fiji/ImageJ | National Institutes of Health | RRID:SCR_002285 |
Fly stocks
Request a detailed protocolDrosophila strains used in this study were reared on cornmeal media, supplemented with yeast. Flies were maintained at 25 °C, unless otherwise mentioned under 12:12 light: dark cycle. WT strain of Drosophila used was Canton S.
Single flight assay
View detailed protocolFlight assays were performed according to Manjila and Hasan, 2018. Briefly, 3–5 day old flies of either sex were tested in batches of 8–10 flies. They were anaesthesized on ice for 2–3 min and then tethered between their head and thorax using a thin metal wire and nail polish. Once recovered, mouth blown air puff was given as a stimulus to initiate flight and flight time was recorded for each fly till 15 min. For all control genotypes, GAL4 or UAS strains were crossed to wild type strain, Canton S. Flight time data is represented in the form of boxplots using Origin software (OriginLab, Northampton, MA). Each box represents 25th to 75th percentile, each open circle represents flight duration of a single fly, solid squares represent the mean and the horizontal line in each box represents the median.
For Gal80ts experiments, larvae, pupae, or adults were maintained at 18 °C and transferred to 29°C only at the stage when the UAS transgene needed to be expressed. Flight assay was done at 25°C. For adult specific expression, flies were grown at 18 °C and transferred to 29°C immediately after eclosion for 2–3 days. For experiments involving Shibirets, larvae, pupae or adults were maintained at 22°C and transferred to 29°C only at the stage when the Shibirets needs to be activated. Flight assay was done at 25°C except for adult-specific activation in which flies were shifted to 29°C 10 min before flight assay and then maintained at 29°C during the experiment.
For optogenetic experiments, flies were transferred to media containing 200 mM all-trans-retinal (ATR) and reared in dark for 2–3 days before imaging experiments.
Ex vivo live imaging
View detailed protocolAdult brains were dissected in Adult hemolymph-like (AHL) saline (108 mM NaCl, 5 mM KCl, 2 mM CaCl2, 8.2 mM MgCl2, 4 mM NaHCO3, 1 mM NaH2PO4, 5 mM trehalose, 10 mM sucrose, 5 mM Tris, pH 7.5) while larval brains were dissected in HL3 (70 mm NaCl, 5 mm KCl, 20 mm MgCl2, 10 mm NaHCO3, 5 mm trehalose, 115 mm sucrose, 5 mm HEPES, 1.5 mm Ca2+, pH 7.2). The dissected brain was mounted on culture dish with anterior side up for recording from cell while posterior side up for imaging mushroom body. They were then embedded in 6 µl of 1% low-melt agarose and bathed in AHL. Images were taken as a time series on an XY plane using a 20x objective on an Olympus FV3000 inverted confocal microscope (Olympus Corp.). Acquisition time is different for different experiments and is described in the figure legends. GCaMP6m, Arclight and GRABDA signals were captured using the 488 nm excitation laser line while 633 nm laser was used for optogenetic stimulation of Chrimson.
Raw fluorescence data were extracted from the marked ROIs using a time series analyzer plugin in Fiji (Balaji, https://imagej.nih.gov/ij/plugins/time-series.html). ΔF/F was calculated using the following formula for each time point (t): ΔF/F = (Ft-F0)/F0, where F0 is the average basal fluorescence of the first 20 frames. Out of the 2 or 3 cells visualised in the brain, the cell which responded the best was taken for further analysis in every case. For analysis of fluorescence changes with GRABDA the right lobe was chosen arbitrarily. Responses for the left lobe are included in a source data and are similar to the right lobe.
To quantify response to stimuli, we calculated area under the curve (AUC). Area under the curve was calculated from the point of stimulation till mean peak response was reached using Microsoft Excel (Microsoft). Time frame for calculating AUC is mentioned in figure legends. AUC is represented as boxplots using Origin software (OriginLab, Northampton, MA). Each box represents 25th to 75th percentile, each open circle represents flight duration of a single fly, solid squares represent the mean and the horizontal line in each box represents the median.
Generation of IP3RDN
Request a detailed protocolFive itpr residues in Drosophila itpr cDNA (Sinha and Hasan, 1999) were mutated using site directed mutagenesis kit (Agilent). The oligonucleotide CAGAGATCGGCAGCAATTGCTGCAGGAACAGTACATCC was used to change K530/R533 to Q while GTACCACGTCTTTCTGCAGACCACCGGACGCACCAG was used to change R272 to Q. All mutations were confirmed using Sanger’s sequencing. Mutated itpr cDNA was subcloned in UAS attB vector (Bischof et al., 2007). UAS ItprDN plasmid was then microinjected in fly embryos at the NCBS fly facility to obtain stable fly strains using standard protocols of fly embryo injection.
Food-seeking assay
Request a detailed protocolFood-seeking assay was performed according to Tsao et al., 2018. Briefly, 18–20 hr starved males (4 days old) of specified genotypes were introduced in petridish (dimensions) with drop of yeast solution in the centre. The yeast solution was prepared by mixing 0.2 g of yeast with 1 g of sucrose in 5 ml distilled water and incubated in a 28°C shaking incubator (170 rpm) for 16 hr. Starved males were then allowed to search for food, and it was considered having found food if it rested for 3 s or longer on food drop. Food-seeking index was calculated as: [Total assay time (600 s) - the time taken to locate food (sec)]/Total assay time (600 s).
Immunohistochemistry
Request a detailed protocolImmunohistochemistry was performed on dissected adult brains as described in Pathak et al., 2015. Briefly, brains were dissected in 1x PBS, followed by fixation in 4% paraformaldehyde for 30 min at room temperature and then 3–4 washings with 0.2% phosphate buffer, pH 7.2 containing 0.2% Triton-X 100 (PTX). They were then blocked in 0.2% PTX containing 5% normal goat serum for four hours at 4°C and incubated overnight with primary antibodies. Next day, they were washed three to four times with 0.2% PTX at room temperature, and then incubated with the respective fluorescent secondary antibodies for 2 hr at room temperature. The primary antibodies used were: rabbit anti-GFP, mouse anti-bruchpilot (anti-brp) antibody. Fluorescent secondary antibodies used at were anti-mouse Alexa Fluor 568 and anti-rabbit Alexa Fluor 488. Confocal images were obtained on the Olympus Confocal FV3000 microscope (Olympus Corp.) with a 20x or with a 40x objective. Images were visualized using Fiji.
Mean intensity fluorescence was obtained for Syt.eGFP and mRFP by averaging intensity values between anterior and posterior limits of the structure.
Western blots
Request a detailed protocolBetween 5 to 10 pupal and adult brains or adult heads of appropriate genotypes were dissected in cold PBS and were homogenized in 30 μl of homogenizing buffer (25 mm HEPES, pH 7.4, 150 mm NaCl, 5% glycerol, 1 mm DTT, 1% Triton X-100, and 1 mm PMSF). 15 μl of the homogenate was run on a 5% SDS-polyacrylamide gel. The protein was transferred to a nitrocellulose membrane by standard protocols. Membrane was then blocked with 5% skim milk followed by incubation with primary antibody at 4°C overnight. The affinity-purified anti-IP3R rabbit polyclonal antibody (IB-9075) was used at a dilution of 1:300 and mouse anti-spectrin antibody was used as a loading control for IP3R. Secondary antibodies used were anti-mouse HRP and anti-rabbit HRP. The protein was then detected on the blot by a chemiluminiscent detection solution. First the spectrin antibody was used to detect protein. The blot was then washed with 3% glacial acetic acid for 40 min and re-probed with IP3R antibody.
Statistical tests
Request a detailed protocolNon-parametric tests were employed to test significance for data that did not follow a normal distribution. Significant differences between experimental genotypes and relevant controls was tested either with the Kruskal-Wallis test followed by Dunn’s multiple comparison test (for multiple comparisons) or with Mann-Whitney U tests (for pairwise comparisons). Data with normal distribution were tested by the Student’s T-test. All statistical tests were performed using Origin 8.0 software. Statistical tests and p-values are mentioned in each figure legend. Source file two is provided with information for all the statistical tests performed.
Model in Figure 7 was created using Biorender (BioRender.com).
Data availability
All data generated or analysed during this study are included in the manuscript and supporting files.
References
-
A subset of serotonergic neurons evokes hunger in adult DrosophilaCurrent Biology 25:2435–2440.https://doi.org/10.1016/j.cub.2015.08.005
-
The role of spontaneous neurotransmission in synapse and circuit developmentJournal of Neuroscience Research 96:354–359.https://doi.org/10.1002/jnr.24154
-
Altered electrical properties in Drosophila neurons developing without synaptic transmissionThe Journal of Neuroscience 21:1523–1531.https://doi.org/10.1523/JNEUROSCI.21-05-01523.2001
-
The inositol trisphosphate/Calcium signaling pathway in health and diseasePhysiological Reviews 96:1261–1296.https://doi.org/10.1152/physrev.00006.2016
-
Functional complementation of Drosophila itpr mutants by rat Itpr1Journal of Neurogenetics 26:328–337.https://doi.org/10.3109/01677063.2012.697501
-
Cytosolic calcium coordinates mitochondrial energy metabolism with presynaptic activityJournal of Neuroscience 32:1233–1243.https://doi.org/10.1523/JNEUROSCI.1301-11.2012
-
Dendritic remodeling and growth of motoneurons during metamorphosis of Drosophila melanogasterThe Journal of Neuroscience 22:4906–4917.https://doi.org/10.1523/JNEUROSCI.22-12-04906.2002
-
Erratum: intracellular channelsCurrent Opinion in Neurobiology 4:758.https://doi.org/10.1016/0959-4388(94)90020-5
-
Neural activity in the central complex of the cockroach brain is linked to turning behaviorsJournal of Experimental Biology 216:992–1002.https://doi.org/10.1242/jeb.080473
-
Developmental analysis of the dopamine-containing neurons of the Drosophila brainJournal of Comparative Neurology 525:363–379.https://doi.org/10.1002/cne.24069
-
Regulation of neuronal physiology by Ca2+ release through the IP3RCurrent Opinion in Physiology 17:1–8.https://doi.org/10.1016/j.cophys.2020.06.001
-
Flight-correlated activity changes in neurons of the lateral accessory lobes in the brain of the locust Schistocerca gregariaJournal of Comparative Physiology A 175:597–610.https://doi.org/10.1007/BF00199481
-
A multicomponent neuronal response encodes the larval decision to pupariate upon amino acid starvationThe Journal of Neuroscience 38:10202–10219.https://doi.org/10.1523/JNEUROSCI.1163-18.2018
-
Independent optical excitation of distinct neural populationsNature Methods 11:338–346.https://doi.org/10.1038/nmeth.2836
-
Neural circuit mechanisms encoding motivational states in DrosophilaCurrent Opinion in Neurobiology 64:135–142.https://doi.org/10.1016/j.conb.2020.05.002
-
Genetic analysis in Drosophila reveals a role for the mitochondrial protein P32 in synaptic transmissionG3: Genes, Genomes, Genetics 2:59–69.https://doi.org/10.1534/g3.111.001586
-
Comparative neuroanatomy of the lateral accessory lobe in the insect brainFrontiers in Physiology 7:244.https://doi.org/10.3389/fphys.2016.00244
-
Calmodulin controls synaptic strength via presynaptic activation of calmodulin kinase IIJournal of Neuroscience 30:4132–4142.https://doi.org/10.1523/JNEUROSCI.3129-09.2010
-
Store-Operated calcium entry through orai is required for transcriptional maturation of the flight circuit in DrosophilaThe Journal of Neuroscience 35:13784–13799.https://doi.org/10.1523/JNEUROSCI.1680-15.2015
-
Calcium-permeable presynaptic AMPA receptors in cerebellar molecular layer interneuronesThe Journal of Physiology 586:5129–5145.https://doi.org/10.1113/jphysiol.2008.159921
-
STIM1 regulates somatic Ca2+ Signals and Intrinsic Firing Properties of Cerebellar Purkinje NeuronsThe Journal of Neuroscience 37:8876–8894.https://doi.org/10.1523/JNEUROSCI.3973-16.2017
-
Differential control of presynaptic CaMKII activation and translocation to active zonesJournal of Neuroscience 31:9093–9100.https://doi.org/10.1523/JNEUROSCI.0550-11.2011
-
Expression of inositol trisphosphate receptorsCell Calcium 26:237–251.https://doi.org/10.1054/ceca.1999.0090
-
Interactions between the inositol 1, 4, 5-trisphosphate and cyclic AMP signaling pathways regulate larval molting in DrosophilaGenetics 158:309–318.
-
Nerve growth cone guidance mediated by G protein-coupled receptorsNature Neuroscience 5:843–848.https://doi.org/10.1038/nn899
Article and author information
Author details
Funding
Department of Science and Technology (National fellowship)
- Gaiti Hasan
National Centre for Biological Sciences (Core Grant)
- Gaiti Hasan
National Centre for Biological Sciences (Graduate fellowship)
- Anamika Sharma
The funders had no role in study design, data collection and interpretation, or the decision to submit the work for publication.
Acknowledgements
This study was supported by grants from DST-SERB and NCBS-TIFR to GH AS was supported by a fellowship from the National Centre for Biological Sciences, TIFR. We thank the Fly Facility, Sequencing facility and Central Imaging and Flow Cytometry Facility at NCBS.
Copyright
© 2020, Sharma and Hasan
This article is distributed under the terms of the Creative Commons Attribution License, which permits unrestricted use and redistribution provided that the original author and source are credited.
Metrics
-
- 2,612
- views
-
- 225
- downloads
-
- 16
- citations
Views, downloads and citations are aggregated across all versions of this paper published by eLife.
Citations by DOI
-
- 16
- citations for umbrella DOI https://doi.org/10.7554/eLife.62297