Charge-driven condensation of RNA and proteins suggests broad role of phase separation in cytoplasmic environments
Figures
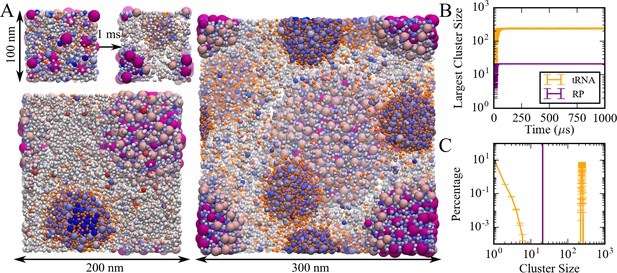
Coarse-grained simulations of a model bacterial cytoplasm.
(A) Initial and final frames for 100 nm box and final frames for 200 and 300 nm boxes are shown with tRNAs in orange, ribosomes in magenta, and other molecules colored according to their charges (blue toward positive charges; red toward negative charges). Sphere sizes are shown proportional to molecular sizes. Large pink spheres correspond to GroEL particles. (B) Size of the largest cluster vs. simulation time in 100 nm system. (C) Cluster size distributions for tRNA and RP during the last 500 µs in the 100 nm system.
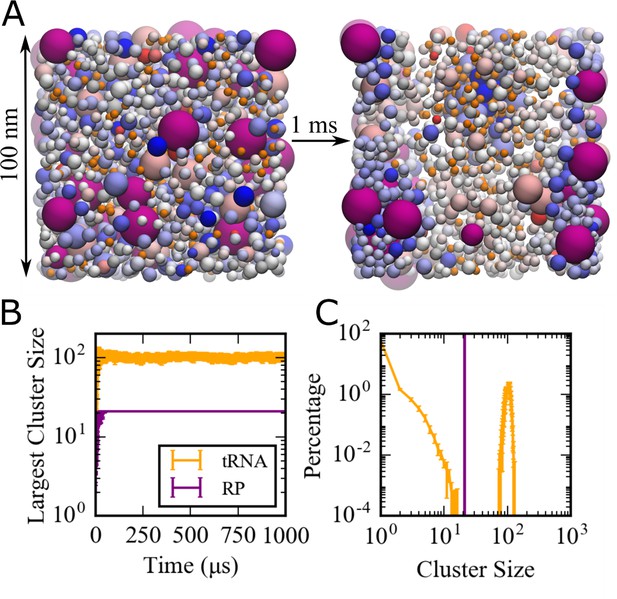
Coarse-grained simulations of a model bacterial cytoplasm with an alternative effective charge model using Equation 6.
(A) Initial and final frames for a 1 ms simulation of a 100 nm system are shown with tRNAs in orange, ribosomes in magenta, and other molecules colored according to their charges (blue toward positive charges; red toward negative charges). Sphere sizes are shown proportional to molecular sizes. Large pink spheres correspond to GroEL particles. (B) Size of the largest cluster vs. simulation time in 100 nm system. (C) Cluster size distributions for tRNA and RP during the last 500 µs.
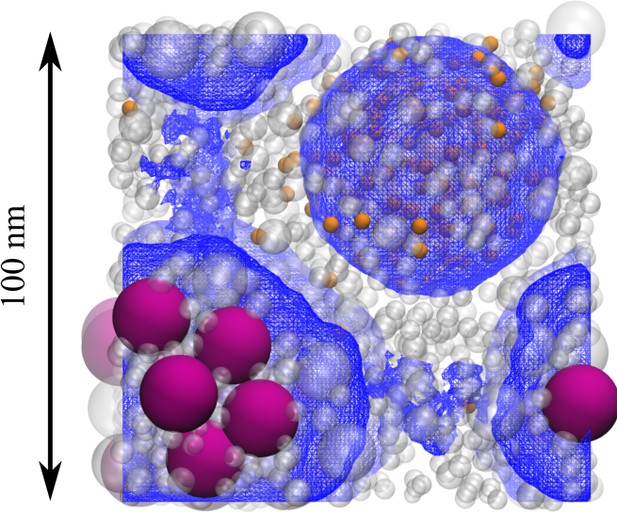
Density variation in the cytoplasmic model system during the last 500 µs of the simulation.
Grid-based contours at volume fractions exceeding 10% are indicated in blue and overlaid onto the final snapshot of the system after 1 ms. The density map was calculated using 10 nm voxel sizes and molecules were counted in a specific voxel if their volume based on their van der Waals radii was covered by that voxel.

Radial distribution curves for tRNA and RP in condensates from the center of their respective condensates.
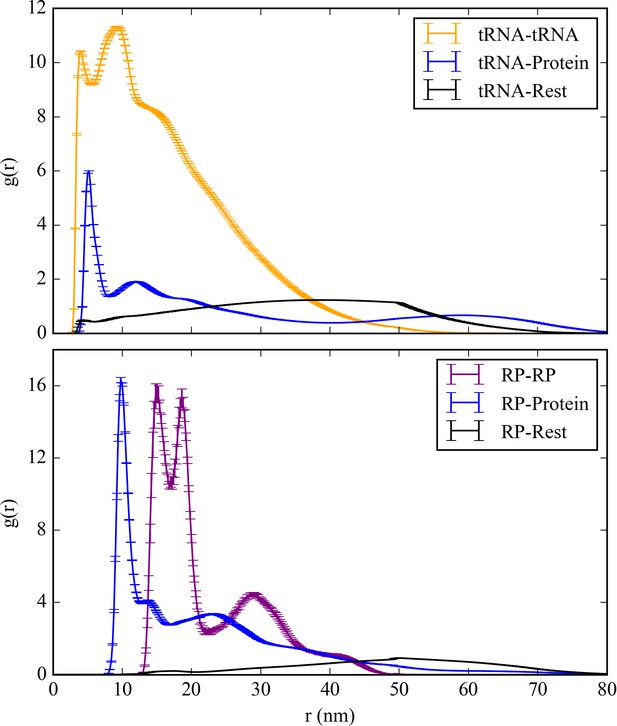
Pairwise radial distribution functions between tRNA, RP, and positively charged protein particles and any other particles in the cytoplasmic model system.
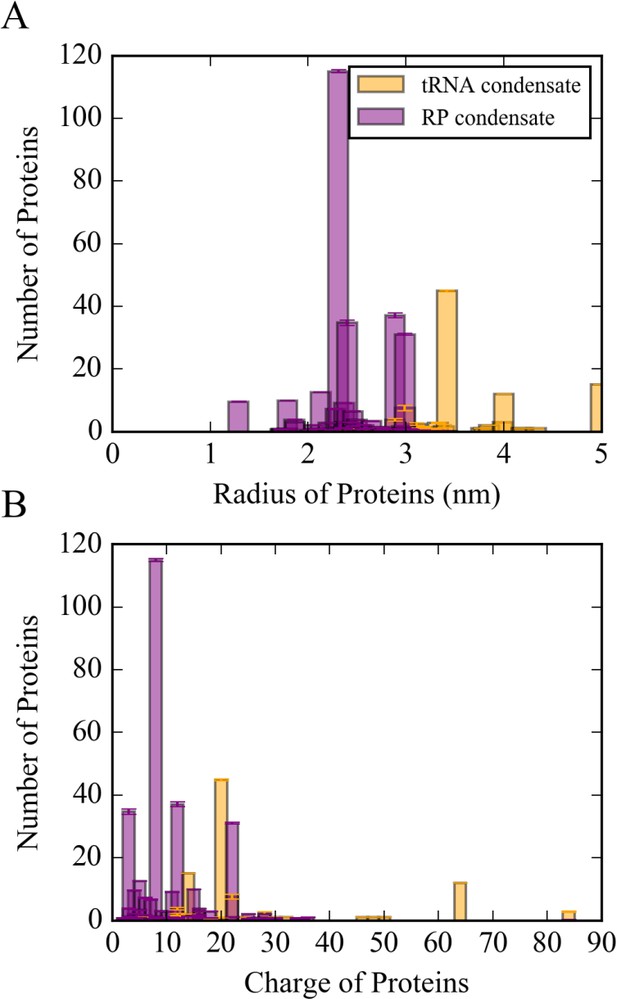
Number of proteins in the tRNA and RP condensates vs. the radius (A) and charge (B) of the proteins found in the condensates.
A 2.2 nm distance cutoff was used to identify molecules as part of the condensates.
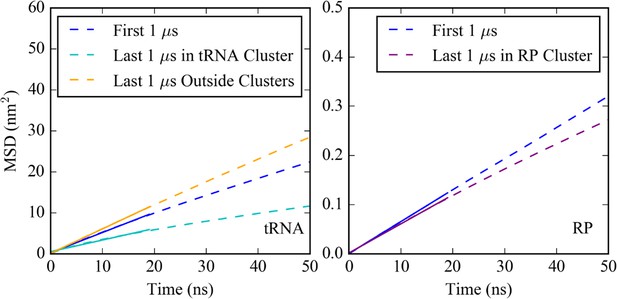
Mean square displacement (MSD) for tRNA (left) and RP (right) during the first and last 1 µs of the cytoplasmic simulations.
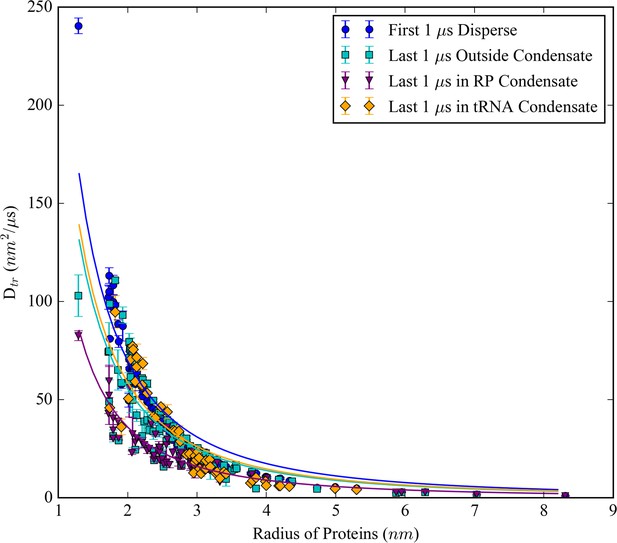
Translational diffusion of macromolecules in the cytoplasmic system as a function of the radius of the macromolecules during the first and last 1 μs of the simulations.
For the last 1 µs, the diffusion coefficients were calculated separately for molecules inside and outside the tRNA and RP condensates. Solid lines depict fitting functions as a function of the particle radius for the dispersed system (Dtr = 279/r2), outside of condensates (Dtr = 222/r2), inside tRNA condensates (Dtr = 235/r2), and inside RP condensates (Dtr = 144/r2).

Comparison of effective charge models that take into counterion condensation according to Equation 5 (orange) or Equation 6 (blue) for moderate (A) and high (B) nominal charges.
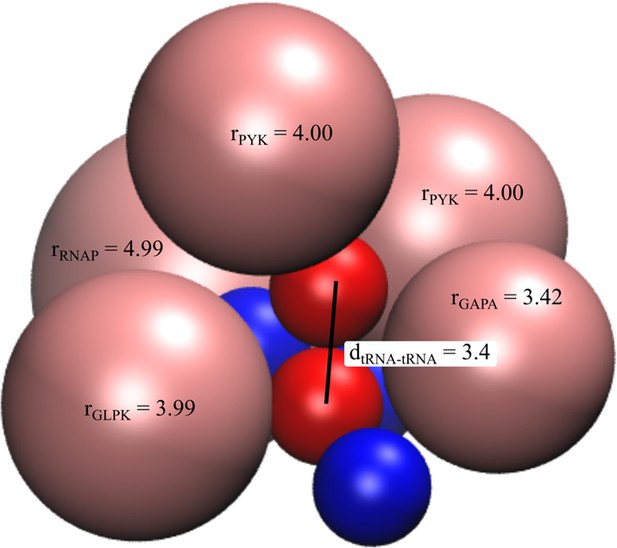
An illustrative example of packing of a tRNA pair (red) in close contact with the positively charged proteins (pink) and other tRNAs (blue) in the cytoplasmic simulations based on the last snapshot after 1 ms simulation.
Intermolecular distances (d) and molecular radii (r) are given in nm.

Radial distribution functions for tRNA-tRNA interactions in the five-component model system with different POSL radii in comparison with the cytoplasmic system.
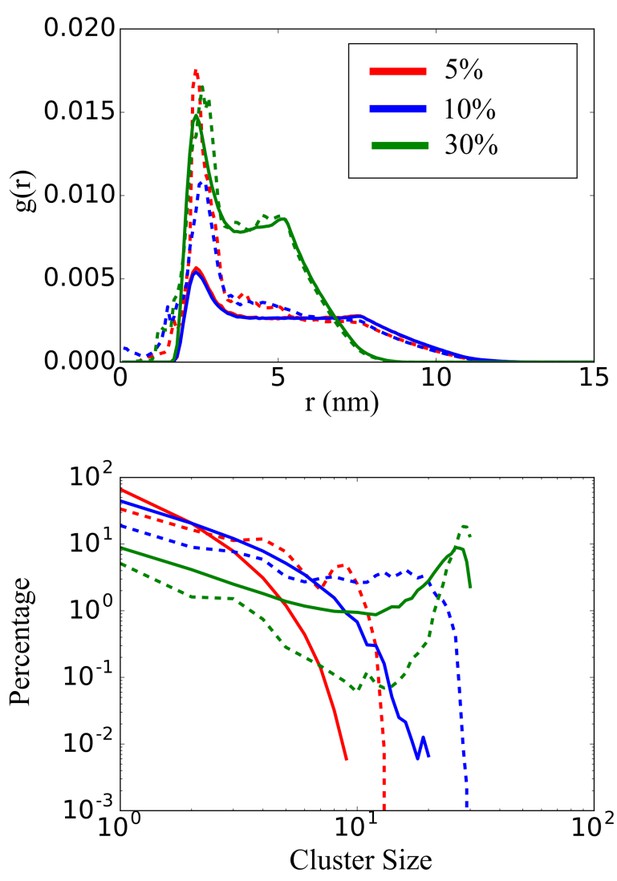
Radial distribution functions of protein-protein pairs (top) and cluster size distributions for proteins (bottom) in simulations of mixtures of villin, protein G, and ubiquitin at volume fractions of 5, 10, and 30%.
Dashed lines show results from previously published all-atom simulations (Hasnain et al., 2014). Solid lines show results from coarse-grained simulations with the spherical colloid-type model described in the Materials and methods section. A value of κ = 1.5 was applied and T = 298 K.

The tRNA cluster at the final snapshot of the cytoplasmic system.
tRNAs inside the cluster from pairs determined with a σij+0.7 nm cutoff are shown in red. Additional tRNA molecules included in the cluster with a σij+2.2 nm cutoff are shown in pink. Other tRNA molecules not considered to be part of the cluster are shown in blue, with the rest of the molecules shown in transparent white.
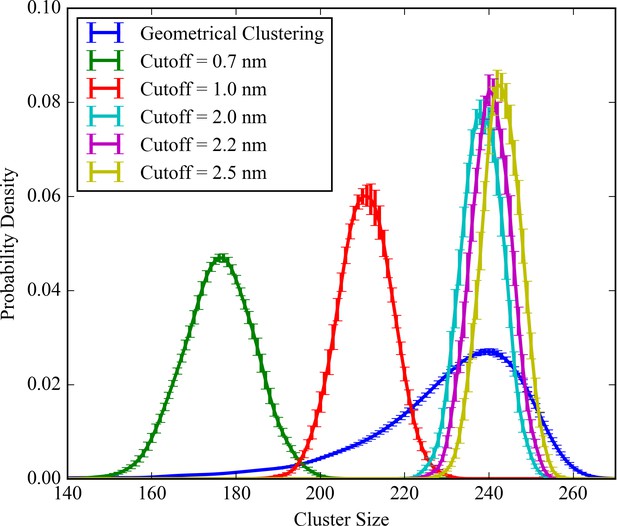
Histograms of tRNA cluster sizes for the cytoplasmic system using the geometrical clustering and based on pairwise contacts using different distance cutoffs added to σij.
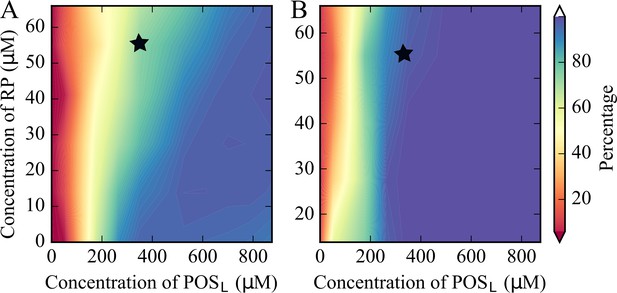
Percentage of tRNA (A) and RP (B) in largest clusters in coarse-grained simulations of the five-component model system as a function of [RP] and [POSL].
The black star indicates the conditions that match the cytoplasmic model. tRNA condensation is a phase separation process.
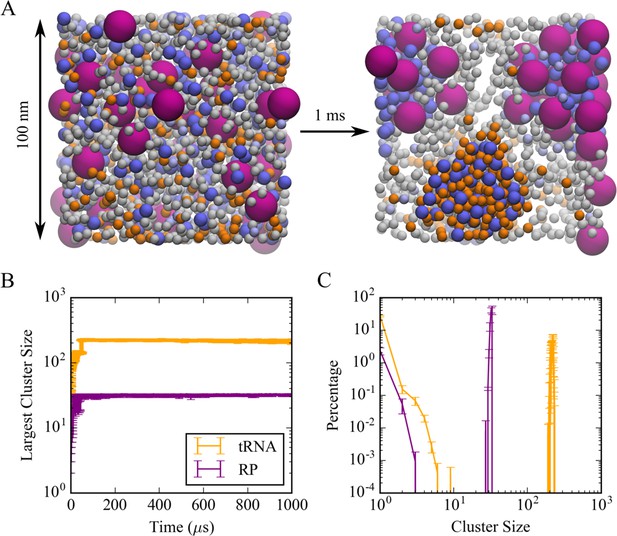
Initial and final frames of the five-component model system simulation (A); time evolution of cluster formation for tRNA and RP clusters (B); and cluster size distributions (C).

Radial distribution functions for interactions between different particle types in the five-component model.

Cluster size distribution of tRNA and RP as a function of [RP] and [POSL] in the five-component model system.
The black star indicates the conditions that match the full cytoplasmic model.
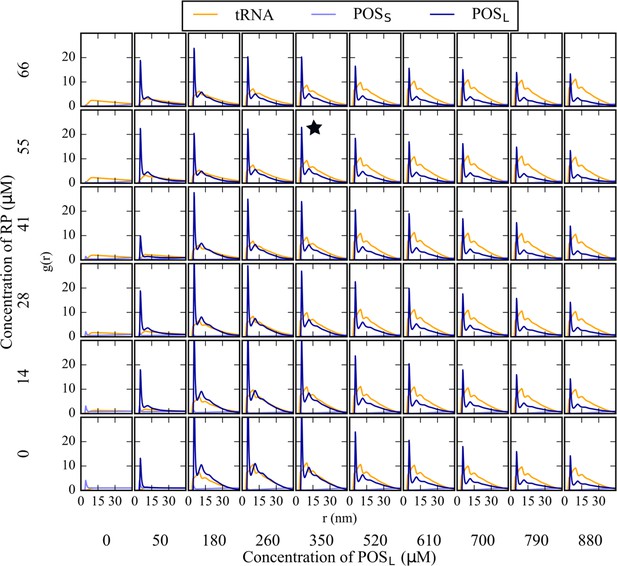
Radial distribution functions for tRNA with tRNA, POSS, and POSL as a function of [RP] and [POSL] in the five-component model system.
The black star indicates the conditions that match the full cytoplasmic model.
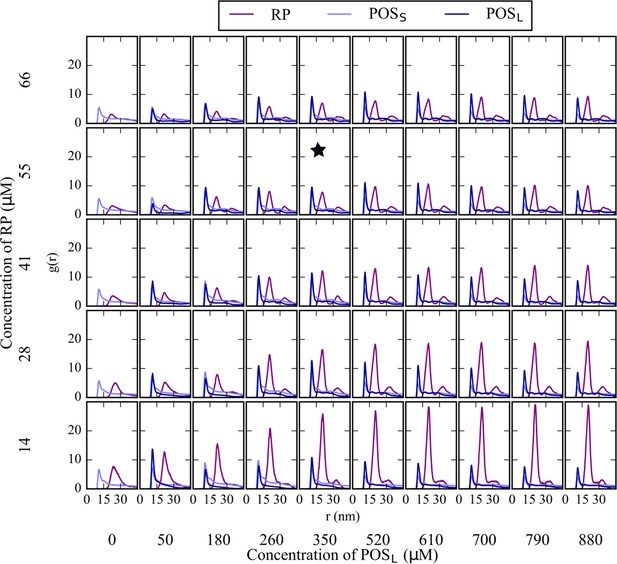
Radial distribution functions for RP with RP, POSS, and POSL as a function of [RP] and [POSL] concentration in the five-component model system.
The black star indicates the conditions that match the full cytoplasmic model.

Relative abundance of POSL and POSS in the largest tRNA and RP clusters with the five-component model as a function of [RP] and [POSL].
(A) Ratio of POSL vs. tRNA; (B) ratio of POSS vs. tRNA; (C) ratio of POSL vs. RP; (D) ratio of POSS vs. RP. The black star indicates the conditions that match the full cytoplasmic model.
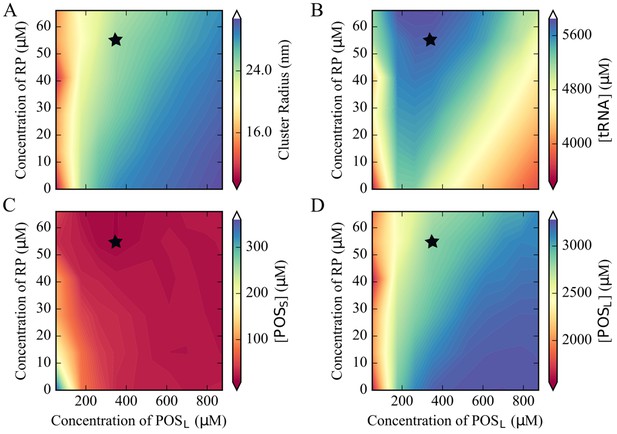
Volume-equivalent radii for largest cluster in tRNA condensates with five-component model (A); macromolecular concentrations inside tRNA condensates for tRNA (B), POSS (C), and POSL (D).
The black star indicates the conditions that match the full cytoplasmic model.
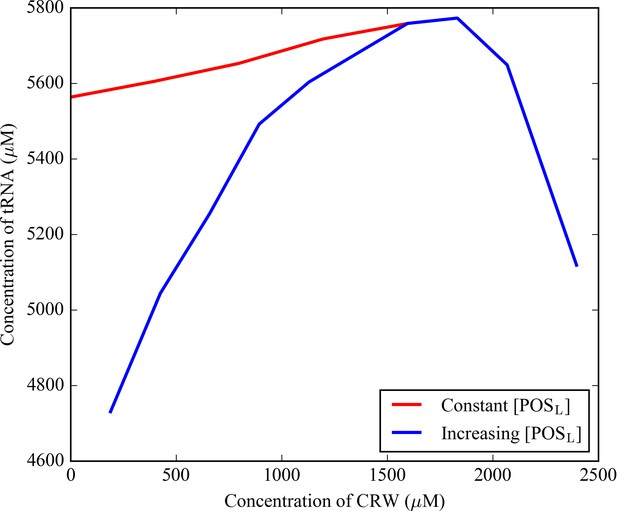
Concentration of tRNA inside the tRNA condensates as a function of [CRW] at constant and increasing values of [POSL] from simulations of the five-component model.
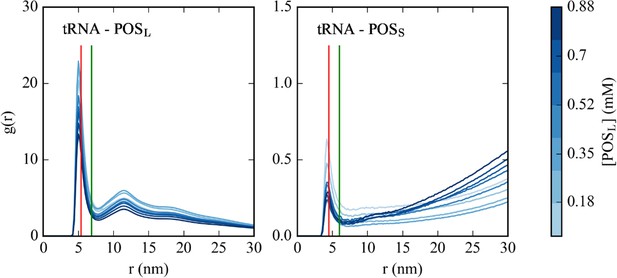
Radial distribution functions between tRNA and POSS/POSL particles in simulations of five-component model at different POSL concentrations and [RP]=55 µM.
Cutoffs based on σij+0.7 nm and σij+2.2 nm are indicated as red and green vertical lines, respectively, with σRNA = 1.55 nm, σPOSL = 3.12 nm, and σPOSS = 2.25 nm.
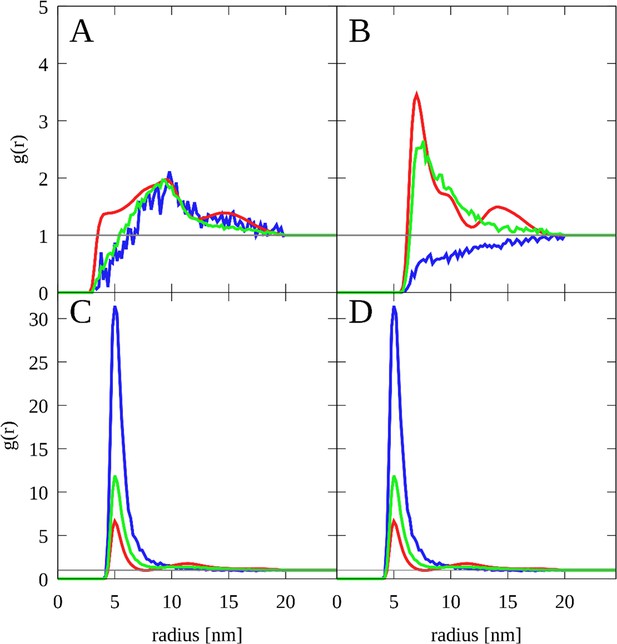
Normalized radial distribution functions for tRNA-tRNA (A), POSL-POSL (B), tRNA-POSL (C), and POSL-tRNA (D) interactions in the condensed (red), dilute (blue), and disperse (green) phases used as input for the theory model.

Probability of minimum RNA-RNA distances in the condensed phase from coarse-grained simulations of the five-component model.
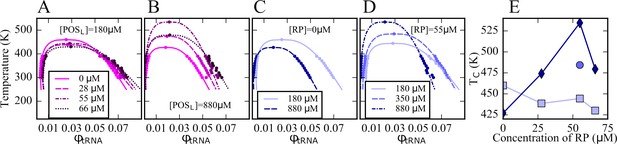
Phase diagrams for tRNA with [POSL]=180 μM and varying RP concentrations (A); with [POSL]=880 μM and varying RP concentrations (B); with [RP]=0 at two [POSL] concentrations (C); and with [RP]=55 μM and varying POSL concentrations (D); critical temperatures as a function of [RP] at [POSL]=180 μM (squares), at [POSL]=880 μM (diamonds), and at [POSL]=350 μM (sphere) (E).
The volume fractions of tRNA in the dilute and condensed phases were obtained based on the number of tRNA particles in the dilute and condensed phases normalized by the respective volumes of the two phases (see Text). Lines in A–D were fitted according to Equations 9 and 10.
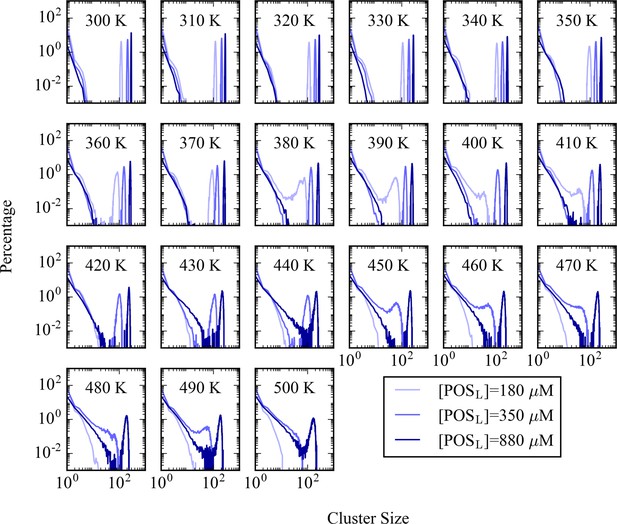
Cluster size distributions of tRNA at [RP]=55 μM and three POSL concentrations (see Legend) for temperatures between 300 and 500 K from simulations of the five-component model.
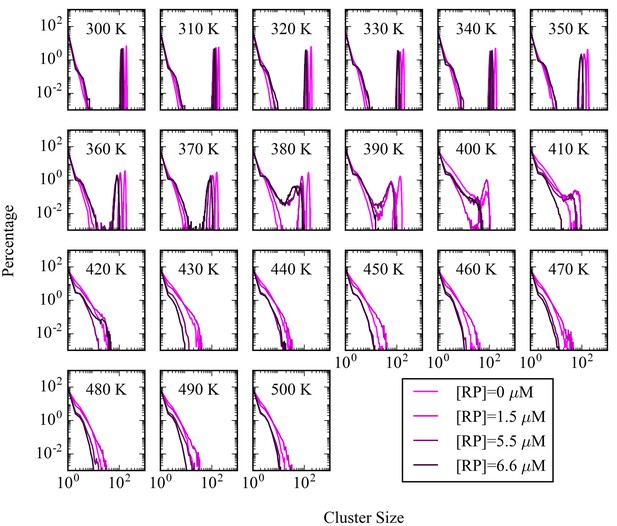
Cluster size distributions of tRNA at [POSL]=180 μM and a range of RP concentrations (see Legend) for temperatures between 300 and 500 K from simulations of the five-component model.

Cluster size distributions of tRNA at [POSL]=880 μM and a range of RP concentrations (see Legend) for temperatures between 300 and 500 K from simulations of the five-component model.
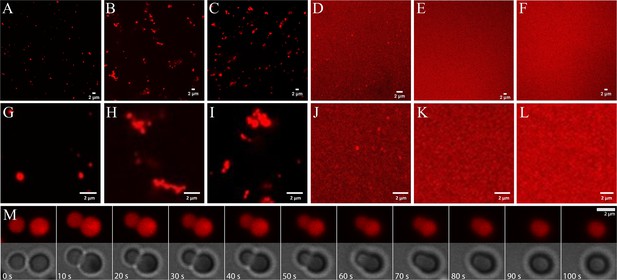
Phase separation in mixtures of J345 RNA at 0.45 mM and various globular proteins at 0.35 mM from confocal microscopy of labeled RNA: trypsin (A; G), ADH (B; H), lysozyme (C; I), LDH (D; J), myoglobin (E; K), BSA (F; L).
Time lapse of droplet merging in RNA-trypsin mixture from fluorescence and bright-field microscopy imaging (M).

Confocal microscopy of labeled J345 RNA for a mixture between J345 RNA at 0.45 mM and trypsin at 0.35 mM.
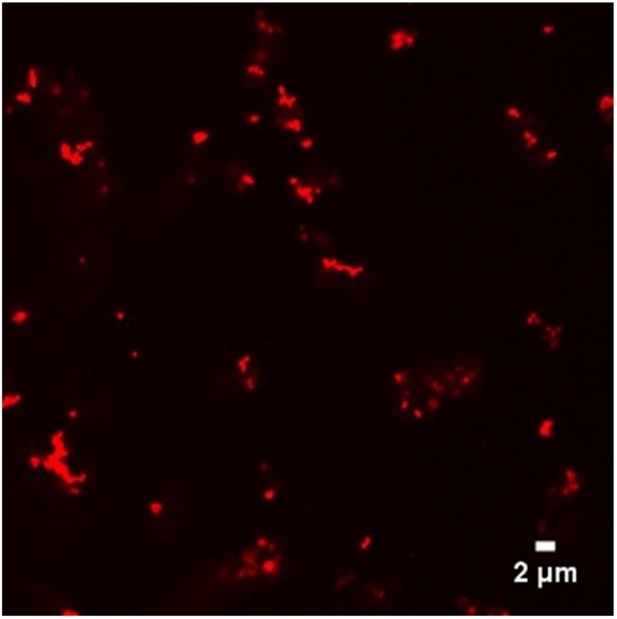
Confocal microscopy of labeled J345 RNA for a mixture between J345 RNA at 0.45 mM and alcohol dehydrogenase at 0.35 mM.
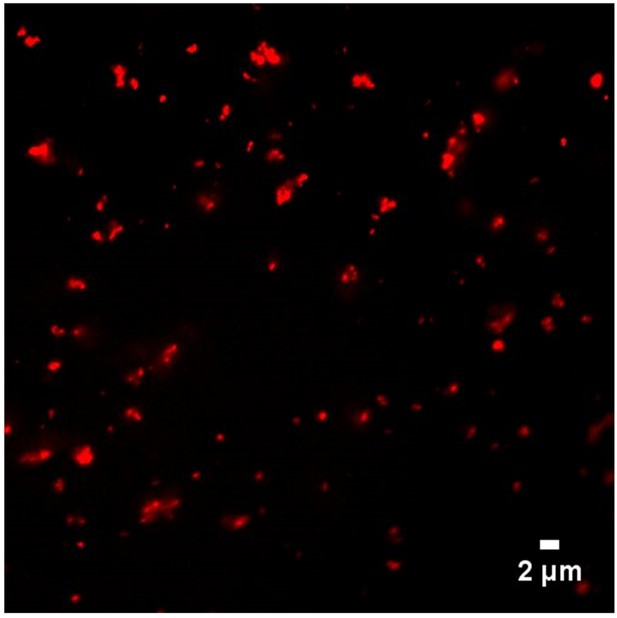
Confocal microscopy of labeled J345 RNA for a mixture between J345 RNA at 0.45 mM and lysozyme at 0.35 mM.

Confocal microscopy of labeled J345 RNA for a mixture between J345 RNA at 0.45 mM and lactate dehydrogenase at 0.35 mM.
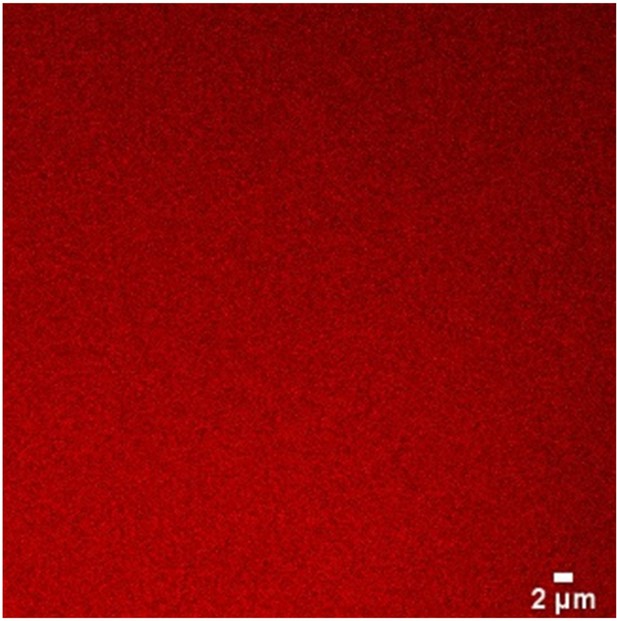
Confocal microscopy of labeled J345 RNA for a mixture between J345 RNA at 0.45 mM and myoglobin at 0.35 mM.
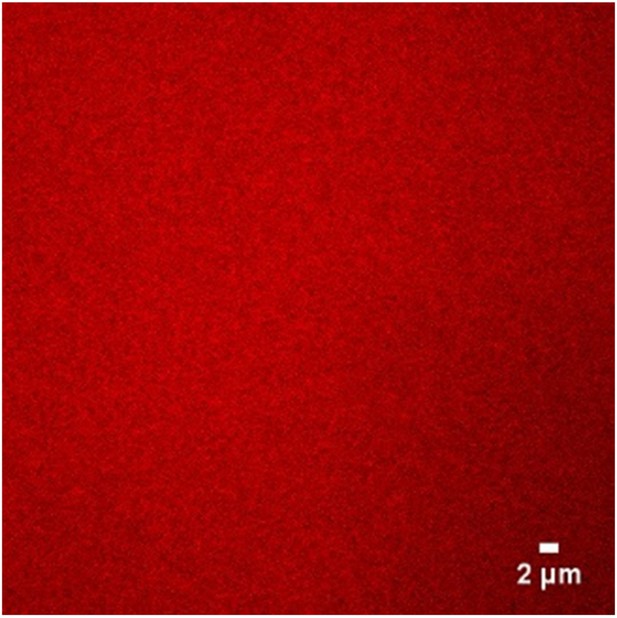
Confocal microscopy of labeled J345 RNA for a mixture between J345 RNA at 0.45 mM and bovine serum albumin at 0.35 mM.
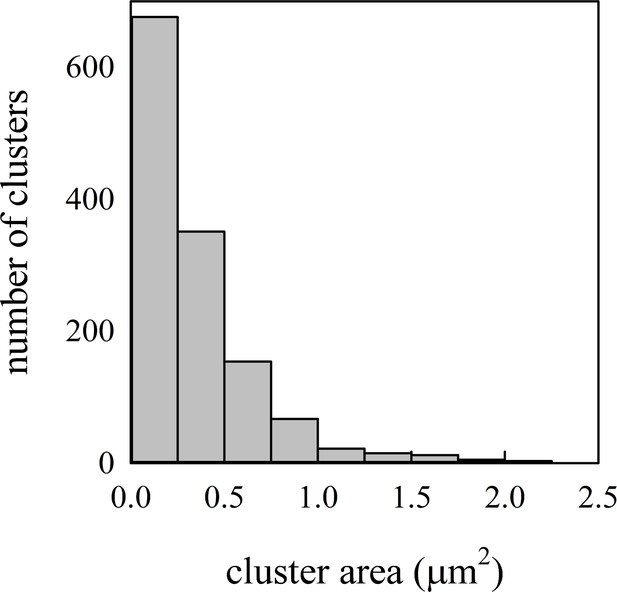
Distribution of cluster sizes from confocal microscopy of labeled J345 RNA in mixtures between J345 RNA at 0.1 mM and trypsin at 0.25 mM.
Note that the first bar represents clusters within the diffraction limit of the microscope.

Circular dichroism spectra of trypsin at 0.150 mM (black), J345 RNA, at 0.037 mM (red), and a mixture of trypsin at 0.150 mM and J345 RNA at 0.029 mM (green).
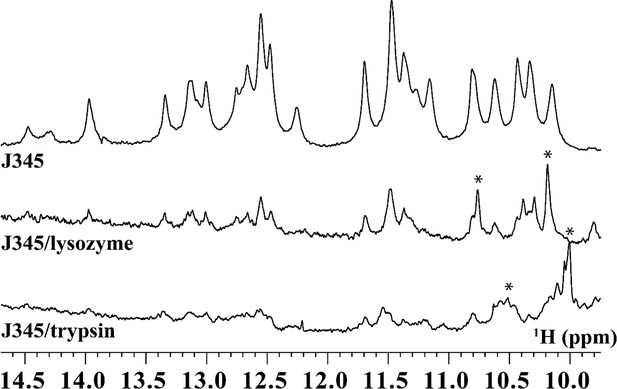
600 MHz 1H NMR spectra in 90:10 H2O:D2O for J345 RNA only (A) and mixtures of RNA with lysozyme (B) and trypsin (C).
Spectral scaling was adjusted to account for higher RNA concentration in the RNA-only sample.
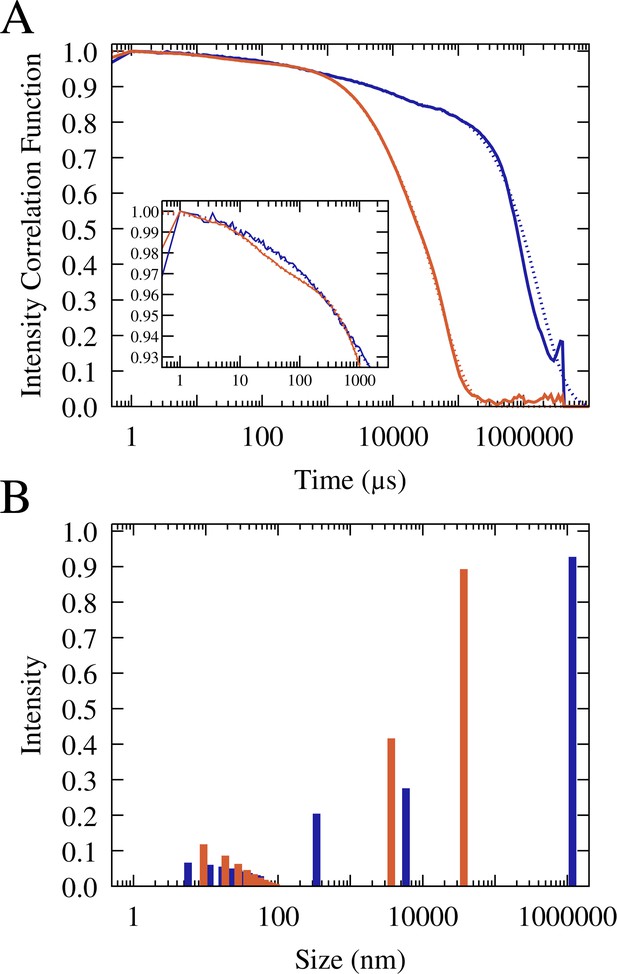
Normalized and averaged scattering intensity correlation functions from triplicate dynamic light scattering experiments of mixtures of 0.1 mM J345 RNA with 0.166 mM trypsin (orange) and 0.4 mM RNA with 0.675 mM lysozyme (blue) (A).
Scattering intensity as a function of particle size from multi-exponential fits to the correlation functions (shown as dotted lines in A) for trypsin (orange) and lysozyme (blue) (B).
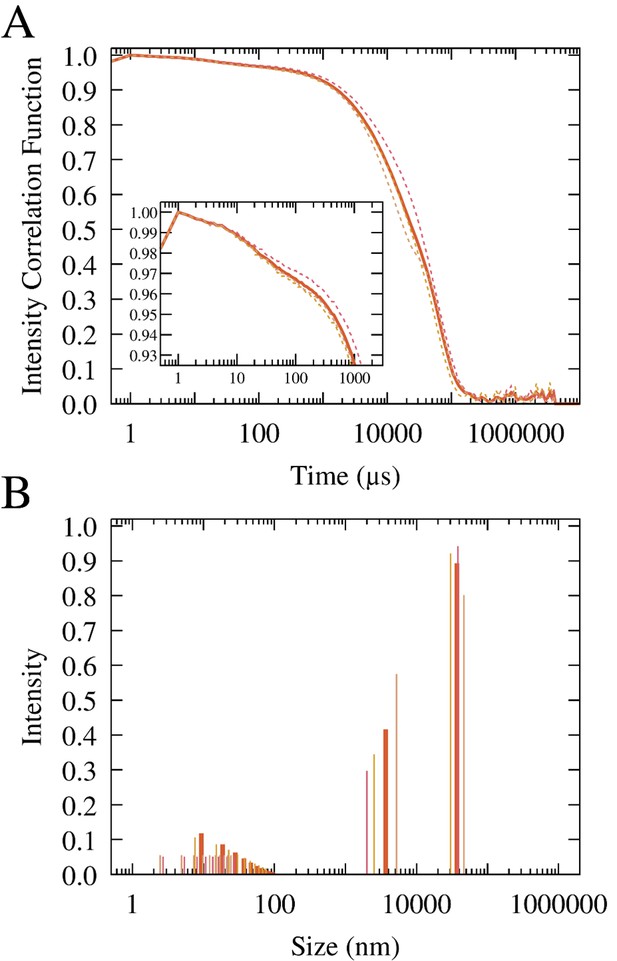
Dynamic light scattering results for RNA-trypsin condensates.
Scattering intensity correlation functions (A) and intensities as a function of particle size from multi-exponential fits (B) from individual dynamic light scattering experiments (dashed/thin lines) of mixtures of 0.1 mM J345 RNA with 0.166 mM trypsin compared with the analysis based on averaged data (thick lines).
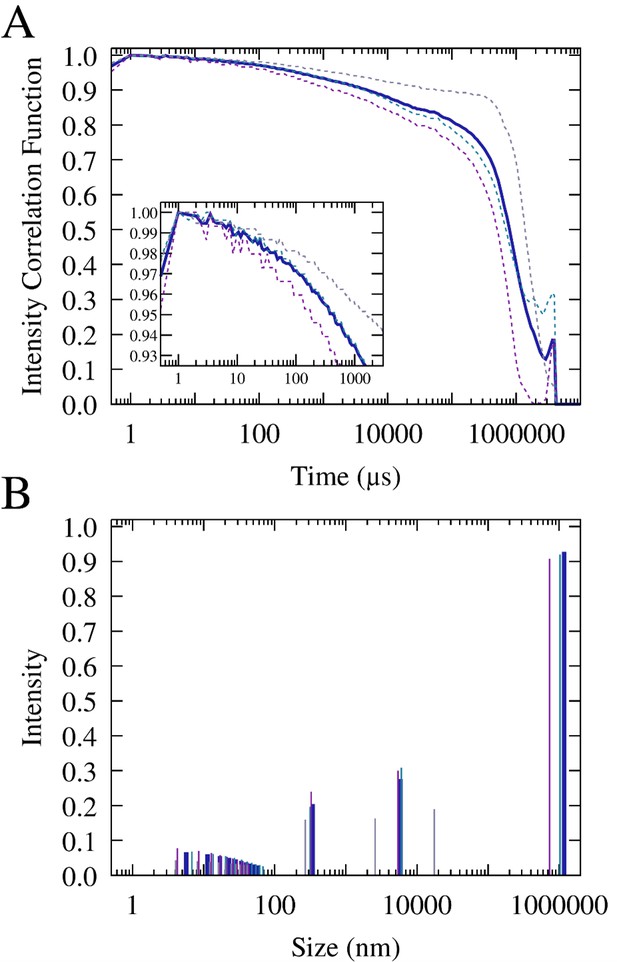
Dynamic light scattering results for RNA-lysozyme condensates.
Scattering intensity correlation functions (A) and intensities as a function of particle size from multi-exponential fits (B) from individual dynamic light scattering experiments (dashed/thin lines) of mixtures of 0.4 mM J345 RNA with 0.675 mM lysozyme compared with the analysis based on averaged data (thick lines).
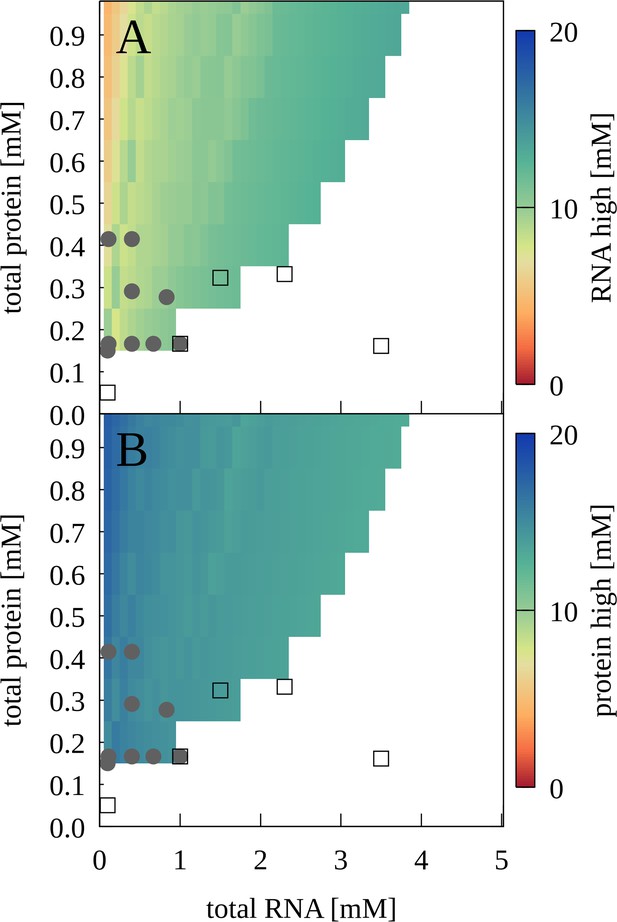
Phase separation for mixtures of J345 RNA and trypsin as a function of total protein and RNA concentrations from experiment and theory.
Grey filled circles indicate concentrations for which phase separation was observed experimentally based on confocal microscopy; empty squares indicate concentrations for which microscopy imaging did not show phase separation. Colors indicate predicted concentrations from theory for RNA (A) and proteins (B) in the condensed phases. No phase separation is predicted for white areas.
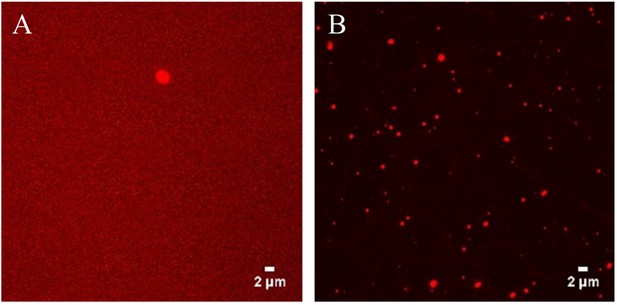
Confocal microscopy of labeled J345 RNA for mixtures between J345 RNA at 0.1 mM and trypsin at 0.05 mM (A) and at 0.15 mM (B).
The single bright spot in (A) is attributed to contamination rather than phase separation.
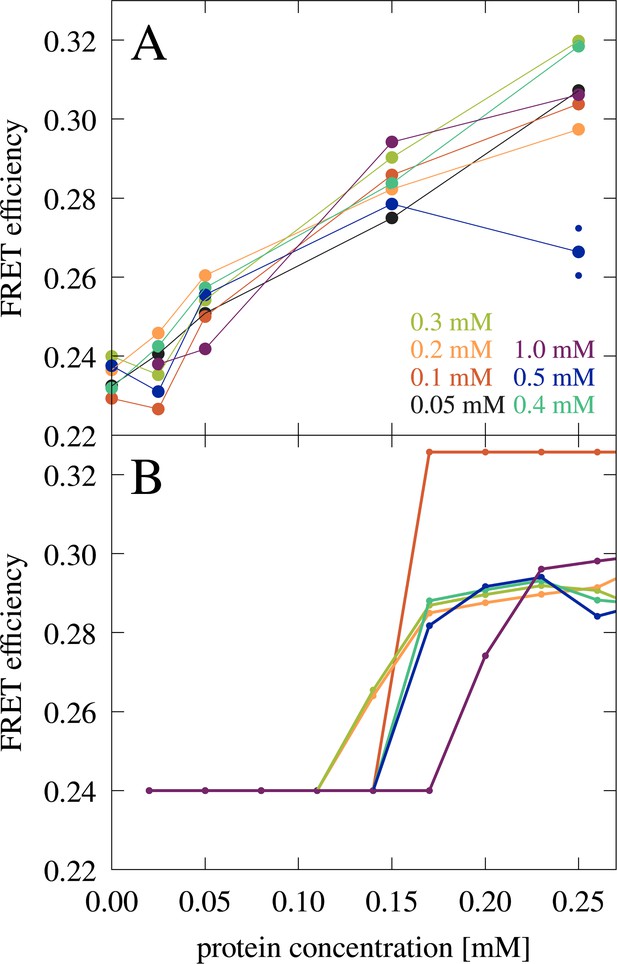
FRET efficiency in mixtures of J345 RNA with trypsin as a function of protein concentration at different RNA concentrations (as indicated by color).
The average of two measurements is shown for 0.25 mM protein and 0.5 mM RNA concentrations with smaller points indicating individual measurements. (A) FRET efficiency estimated from the fraction of RNA in the condensed phase from theory (B). In every measurement, the concentration of Cy3- and Cy5-labeled RNA is constant, 8 µM and 42 µM, respectively.

Snapshots after 1 ms for binary RNA-protein mixtures at T = 298K, with κ = 0.7 and using effective charges according to Equation 5.
[RNA]=0.493 mM and [protein]=0.350 mM. Orange and blue spheres show RNA and proteins, according to size. Concentrations inside the condensates were [RNA:lysozyme]=20.2:20.2 mM; [RNA:trypsin]=16.5:15.2 mM; [RNA:LDH]=9.6:7.2 mM; [RNA:ADH]=9.5:6.7 mM.

Snapshots from CG simulations after 1 ms for binary RNA-protein mixtures at T = 298K, with κ = 0.75 using Equation 6 to obtain effective charges.
[RNA]=0.493 mM and [protein]=0.350 mM. Orange and blue spheres show RNA and proteins, according to size. Concentrations inside the condensates were [RNA:lysozyme]=18.6:18.4 mM; [RNA:trypsin]=15.6:14.8 mM; [RNA:LDH]=8.8:7.2 mM; [RNA:ADH]=8.7:6.6 mM.

Concentrations of RNA (A) and proteins (B) in dilute and condensed phases as a function of temperature with κ = 1.17.
rRNA = 1.47 nm, qRNA = −46, [RNA]=0.45 mM, [protein]=0.35 mM. Colors indicate proteins: trypsin (blue), alcohol dehydrogenase (violet), lysozyme (red), lactate dehydrogenase (tan), myoglobin (green), cytochrome C (dark red).
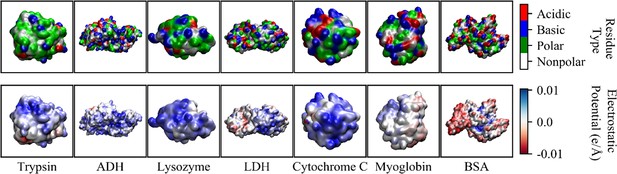
Charge distribution on protein surfaces based on amino acid residue types (top; basic: blue, acidic: red, polar: green, hydrophobic: white) and electrostatic potentials calculated via a Poisson-Boltzmann continuum model (bottom) with coloring according to the sign of the potential (positive: blue, negative: red).
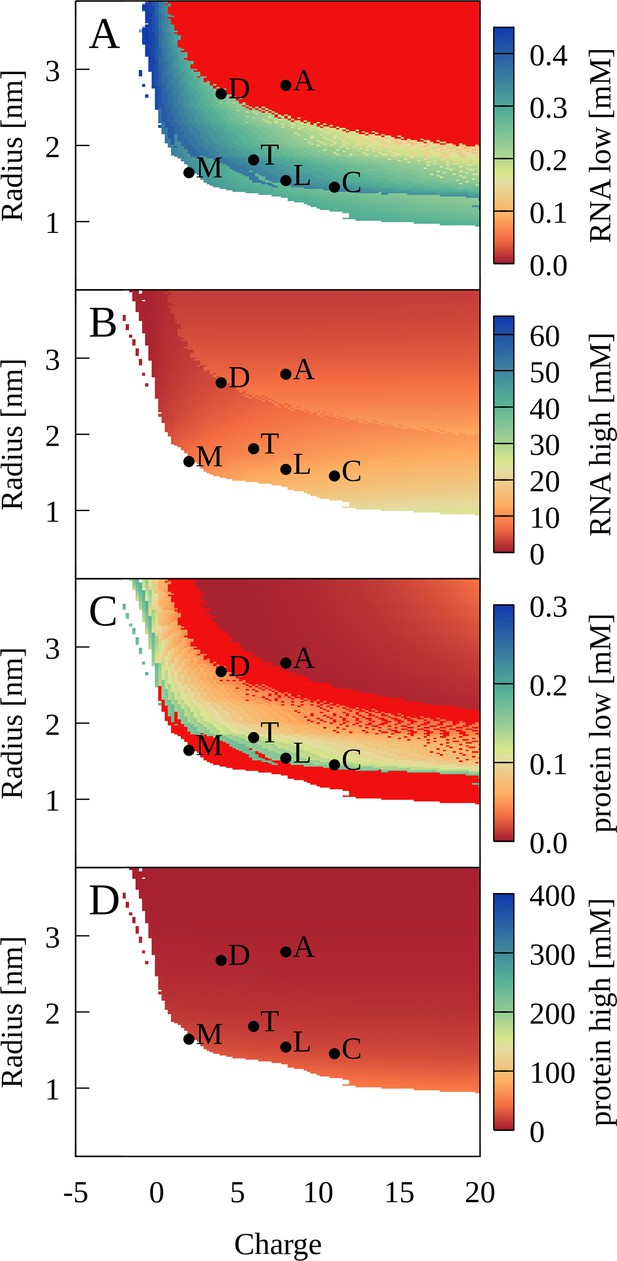
Phase separation for binary RNA-protein mixtures as a function of protein charge and radius from theory.
Colors show [RNA] (A, B) and [protein] (C, D) in dilute (A, C) and condensed (B, D) phases. Red indicates zero concentration. [RNA]=0.45 mM, [protein]=0.35 mM, κ = 1.17, and T = 298 K. Corresponding properties for proteins are denoted as follows: myoglobin (M); trypsin (T); lysozyme (L); cytochrome C (C); LDH (D); ADH (A).

Phase separation for mixtures between RNA and alcohol dehydrogenase as a function of total protein and RNA concentrations.
Colors indicate predicted concentrations for RNA (A, B) and proteins (C, D) in dilute (A, C) and condensed (B, D) phases. Bright red color indicates zero concentration (i.e. no phase coexistence for that component); no phase separation is predicted for white areas. rRNA = 1.47 nm, qRNA = −46, rprotein = 2.79 nm, qprotein = 8. The Debye-Hückel screening term was set to κ = 1.17 and T = 298 K.

Phase separation for mixtures between RNA and lactate dehydrogenase as a function of total protein and RNA concentrations.
Colors indicate predicted concentrations for RNA (A, B) and proteins (C, D) in dilute (A, C) and condensed (B, D) phases. Bright red color indicates zero concentration (i.e. no phase coexistence for that component); no phase separation is predicted for white areas. rRNA = 1.47 nm, qRNA = −46, rprotein = 2.68 nm, qprotein = 4. The Debye-Hückel screening term was set to κ = 1.17 and T = 298 K.
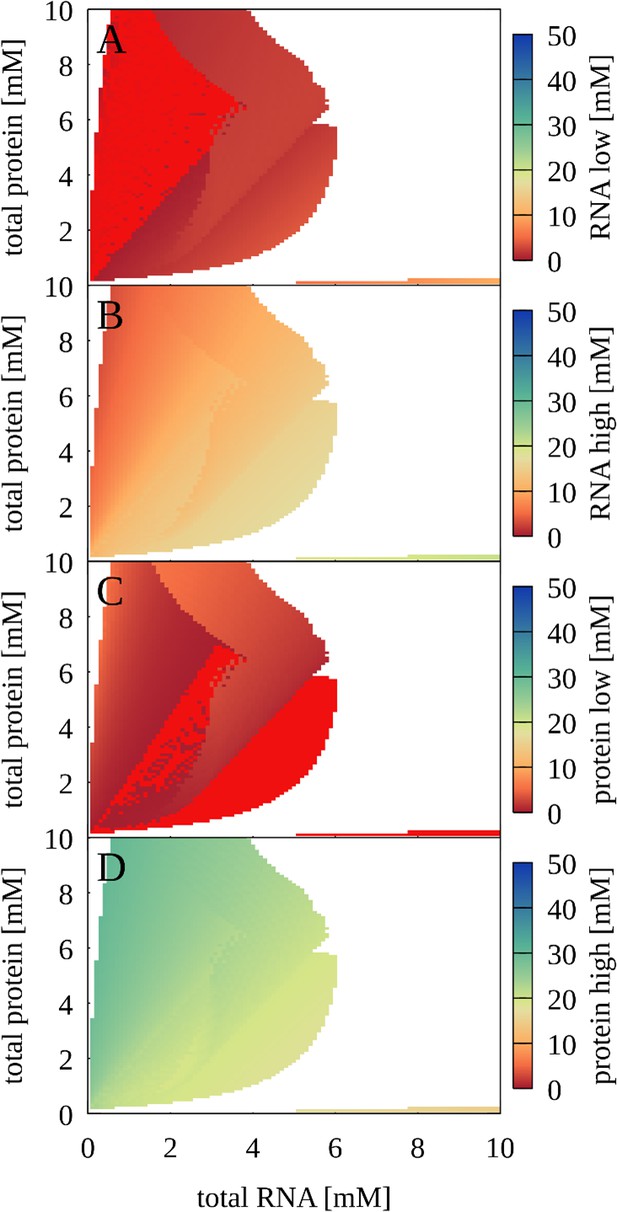
Phase separation for mixtures between RNA and lysozyme as a function of total protein and RNA concentrations.
Colors indicate predicted concentrations for RNA (A, B) and proteins (C, D) in dilute (A, C) and condensed (B, D) phases. Bright red color indicates zero concentration (i.e. no phase coexistence for that component); no phase separation is predicted for white areas. rRNA = 1.47 nm, qRNA = −46, rprotein = 1.54 nm, qprotein = 8. The Debye-Hückel screening term was set to κ = 1.17 and T = 298 K.
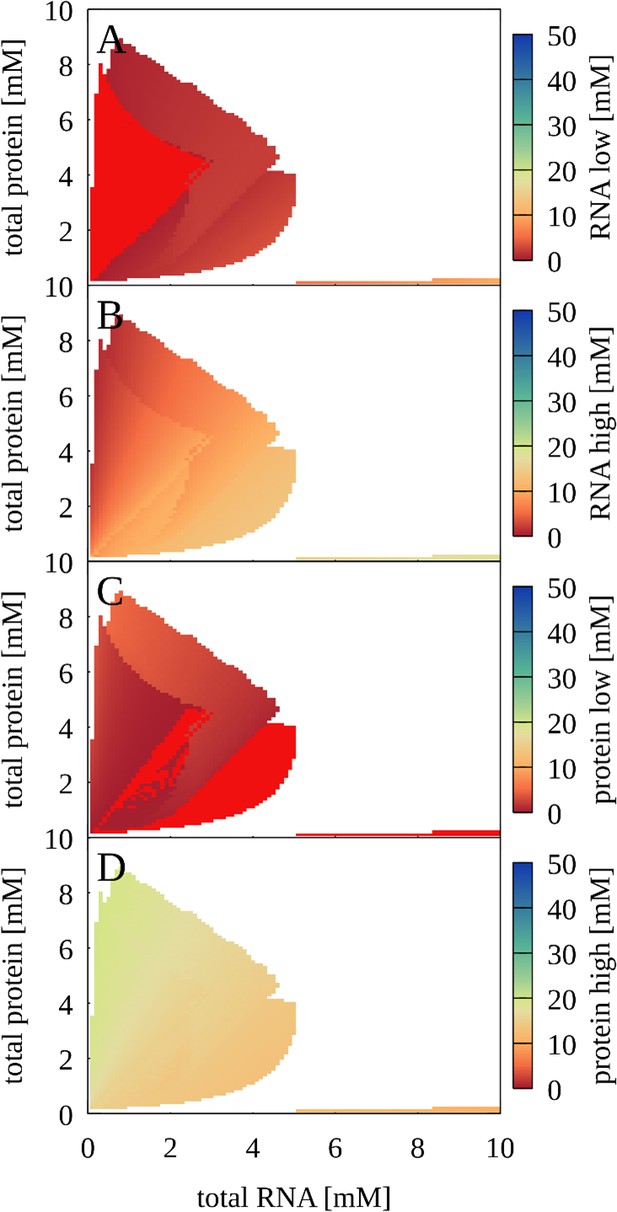
Phase separation for mixtures between RNA and trypsin as a function of total protein and RNA concentrations.
Colors indicate predicted concentrations for RNA (A, B) and proteins (C, D) in dilute (A, C) and condensed (B, D) phases. Bright red color indicates zero concentration (i.e. no phase coexistence for that component); no phase separation is predicted for white areas. rRNA = 1.47 nm, qRNA = −46, rprotein = 1.81 nm, qprotein = 6. The Debye-Hückel screening term was set to κ = 1.17 and T = 298 K.
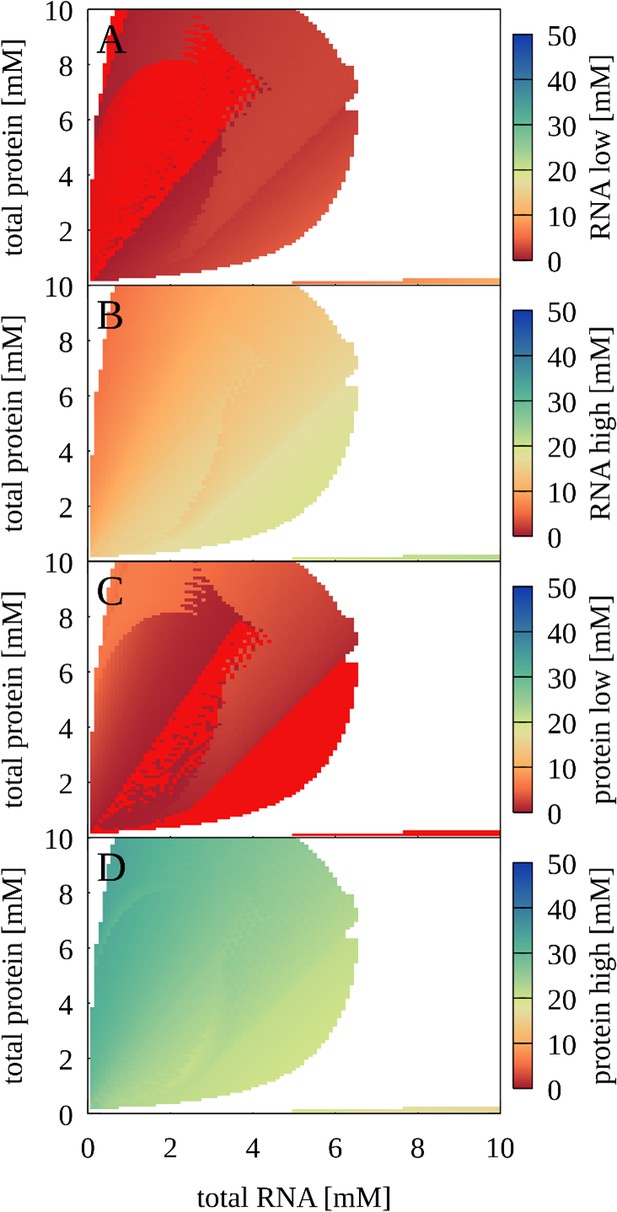
Phase separation for mixtures between RNA and cytochrome C as a function of total protein and RNA concentrations.
Colors indicate predicted concentrations for RNA (A, B) and proteins (C, D) in dilute (A, C) and condensed (B, D) phases. Bright red color indicates zero concentration (i.e. no phase coexistence for that component); no phase separation is predicted for white areas. rRNA = 1.47 nm, qRNA = −46, rprotein = 1.45 nm, qprotein = 11. The Debye-Hückel screening term was set to κ = 1.17 and T = 298 K.

Phase separation for mixtures between RNA and myoglobin as a function of total protein and RNA concentrations.
Colors indicate predicted concentrations for RNA (A, B) and proteins (C, D) in dilute (A, C) and condensed (B, D) phases. Bright red color indicates zero concentration (i.e. no phase coexistence for that component); no phase separation is predicted for white areas. rRNA = 1.47 nm, qRNA = −46, rprotein = 1.64 nm, qprotein = 2. The Debye-Hückel screening term was set to κ = 1.17 and T = 298 K.
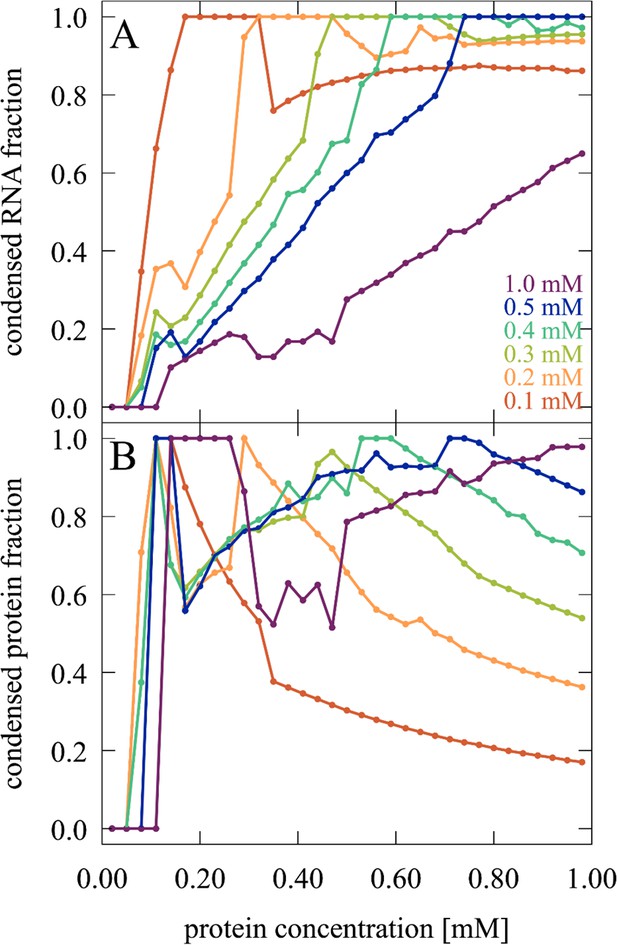
Fraction of RNA (A) and protein (B) in the condensed phases predicted by the theory model for trypsin as a function of protein concentration at different total RNA concentrations.
Results represent averages over three subsequent values from values obtained at protein concentrations at increments of 0.01 mM.
Videos
Simulation of bacterial cytoplasm model.
Trajectory of the 100 nm system during the last 1 µs of a 1 ms simulation with tRNAs in orange, ribosomes in magenta, and other molecules colored according to their charges (blue toward positive charges; red toward negative charges). Sphere sizes are shown proportional to molecular sizes. Large pink spheres correspond to GroEL particles.
Merging of trypsin-RNA liquid condensate droplets.
Video of two representative examples of liquid droplet dynamics in trypsin-RNA mixtures with J345 RNA at 0.45 mM and proteins at 0.35 mM from confocal microscopy of fluorescent-labeled RNA (left) and corresponding bright-field imaging (right). Time evolution is accelerated 25x (i.e. the movies correspond to about 100 s in real time).
Merging of trypsin-RNA liquid condensate droplets.
Video of two representative examples of liquid droplet dynamics in trypsin-RNA mixtures with J345 RNA at 0.45 mM and proteins at 0.35 mM from confocal microscopy of fluorescent-labeled RNA (left) and corresponding bright-field imaging (right). Time evolution is accelerated 25x (i.e. the movies correspond to about 100 s in real time).
Tables
Multi-exponential fits of dynamic light scattering correlation functions.
System* | Clusters | Size 1 | Size 2 | Size 3 | Size 4 | χ2 | ||||||
---|---|---|---|---|---|---|---|---|---|---|---|---|
Dc (nm) | ac | tc | D1 (nm) | a1 | D2 (nm) | a2 | D3 (µm) | a3 | D4 (µm) | a4 | *10−3 | |
Lysozyme #1 | 6.8 | 0.076 | 9.4 | 314.5 | 0.197 | 6061 | 0.309 | 1037.0 | 0.919 | 0.362 | ||
Lysozyme #2 | 4.3 | 0.085 | 10.5 | 325.4 | 0.240 | 5416 | 0.300 | 730.4 | 0.908 | 0.91 | ||
Lysozyme #3 | 4.0 | 0.045 | 21.7 | 270.0 | 0.160 | 2585 | 0.163 | 17.6 | 0.190 | 28,373.6 | 0.948 | 0.13 |
Lysozyme avg. | 5.6 | 0.073 | 10.4 | 339.4 | 0.204 | 5848 | 0.275 | 1184.7 | 0.927 | 0.16 | ||
Trypsin #1 | 7.6 | 0.129 | 5.0 | 2544 | 0.345 | 30,167 | 0.921 | 1.6 | ||||
Trypsin #2 | 2.7 | 0.051 | 186,625 | 2003 | 0.297 | 38,323 | 0.942 | 1.46 | ||||
Trypsin #3 | 2.4 | 0.055 | 106,796 | 5210 | 0.575 | 46,527 | 0.801 | 1.05 | ||||
Trypsin avg. | 9.3 | 0.162 | 3.2 | 3680 | 0.417 | 36,967 | 0.893 | 2.31 |
-
*All systems are mixtures between protein and J345 RNA.
Simulation systems for coarse-grained model validation.
System | Villin | Protein G | Ubiquitin | Box (nm) | ||||||
---|---|---|---|---|---|---|---|---|---|---|
Volume percentage | G/L | mM | Np* | G/L | mM | Np* | G/L | mM | Np* | |
5% | 9.7 | 2.3 | 5 | 14.3 | 2.3 | 5 | 19.8 | 2.3 | 5 | 15.3 |
10% | 19.0 | 4.5 | 10 | 28.2 | 4.5 | 10 | 39.0 | 4.5 | 10 | 15.4 |
30% | 57.9 | 13.8 | 30 | 85.7 | 13.8 | 30 | 118.6 | 13.8 | 30 | 10.6 |
-
*Number of proteins.
Additional files
-
Supplementary file 1
Components in cytoplasmic model, CG parameters, and self-diffusion.
Macromolecular components in a previously established model of the bacterial cytoplasm of Mycoplasma genitalium (Nguemaha and Zhou, 2018) with their molecular properties and corresponding CG model parameters. Self-diffusion rates extracted from the CG simulations are reported for different parts of the trajectory and different parts of the system (tRNA clusters, RP clusters, outside clusters).
- https://cdn.elifesciences.org/articles/64004/elife-64004-supp1-v2.xlsx
-
Supplementary file 2
Five-component model simulations.
List of the five-component model simulations with molecular compositions, CG parameters, and simulation conditions.
- https://cdn.elifesciences.org/articles/64004/elife-64004-supp2-v2.xlsx
-
Transparent reporting form
- https://cdn.elifesciences.org/articles/64004/elife-64004-transrepform-v2.pdf