Instantaneous movement-unrelated midbrain activity modifies ongoing eye movements
Figures
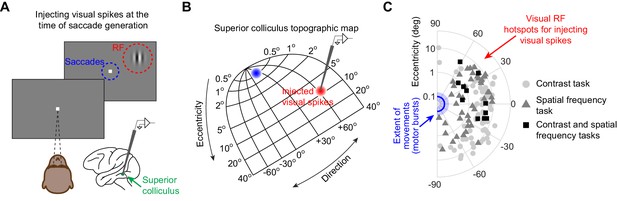
Injecting arbitrary, movement-unrelated spiking activity into the SC map around the time of saccade generation.
(A) A monkey steadily fixated while we presented an eccentric stimulus in a recorded neuron’s RF (red). In experiment 1, the stimulus consisted of a vertical grating of 2.2 cycles/deg spatial frequency, and the stimulus contrast was varied across trials (Chen et al., 2015). In experiment 2, the stimulus consisted of a high contrast vertical grating having either 0.56, 2.2, or 4.4 cycles/deg spatial frequency (Khademi et al., 2020). The stimulus location was spatially dissociated from the motor range of microsaccades being generated (blue). This allowed us to experimentally inject movement-unrelated ‘visual’ spikes into the SC map around the time of microsaccade generation. (B) We injected ‘visual’ spikes at eccentric retinotopic locations (red) distinct from the neurons that would normally exhibit motor bursts for microsaccades (blue). The shown SC topographic map is based on our earlier dense mappings revealing both foveal and upper visual field tissue area magnification (Hafed et al., 2021). (C) Across experiments 1 and 2, we measured ‘visual’ spikes from a total of 128 neurons with RF hotspots indicated by the symbols. The blue line and shaded area denote the mean and 95% confidence interval, respectively, of all microsaccade amplitudes that we observed. The neurons in which we injected ‘visual’ spikes (symbols) were not involved in generating these microsaccades (Figure 1—figure supplement 1; also see Khademi et al., 2020). The origin of the shown log-polar plot corresponds to 0.03 deg eccentricity (Hafed and Krauzlis, 2012). Across experiments 1 and 2, 11 neurons were run on both experiments, 73 neurons were run on only experiment 1, and 44 neurons were run on only experiment 2.
-
Figure 1—source data 1
Excel table with the source data for this figure.
- https://cdn.elifesciences.org/articles/64150/elife-64150-fig1-data1-v2.xlsx
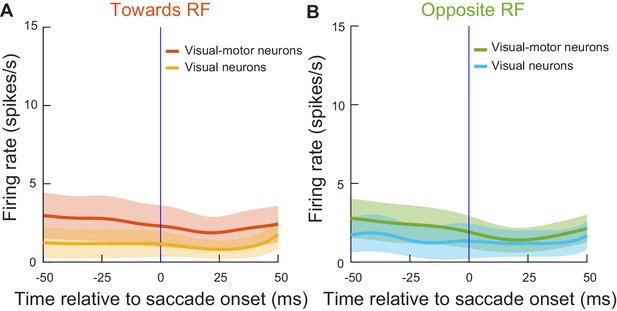
Injected ‘visual’ spikes in our experiments were in neurons that were not directly involved in generating the microsaccades that were being altered in our main analyses.
(A) Mean firing rate of all of our visual neurons (30 neurons) or visual-motor neurons (54 neurons) from experiment one aligned to saccade onset for eye movements directed towards the RF direction. The movements were all selected during a baseline pre-stimulus period (−100 to −25 ms from stimulus onset) in the absence of any visual stimuli inside the RF’s. (B) Similar analysis for the same neurons but with microsaccades directed opposite the RF direction. In all cases, the neurons did not show any bursts for microsaccade generation, confirming that we were only measuring visual bursts and not concurrent microsaccade-related activity in Figures 2–6 (also see Figures 8–12). This also means that there was no merging of visual bursts with motor bursts into distorted motor bursts for the microsaccades in this study that were modified in amplitude (e.g. Figures 2–12). For the neurons from experiment 2, a similar analysis (and with similar conclusions) was already documented earlier (Khademi et al., 2020). Error bars denote 95% confidence intervals.
-
Figure 1—figure supplement 1—source data 1
Excel table with the source data for this figure.
- https://cdn.elifesciences.org/articles/64150/elife-64150-fig1-figsupp1-data1-v2.xlsx
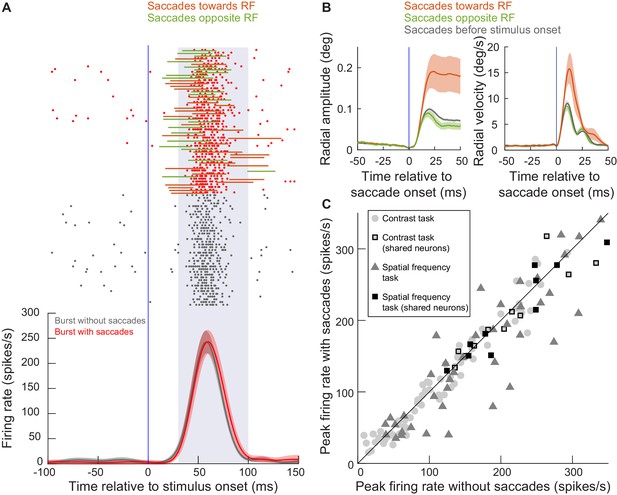
SC visual bursts still occurred intra-saccadically.
(A) We measured the firing rate of an example neuron (from experiment 1) when a stimulus appeared inside its RF without any nearby microsaccades (gray firing rate curve and spike rasters) or when the same stimulus appeared while microsaccades were being executed around the time of visual burst occurrence (red firing rate curve and spike rasters). The stimulus eccentricity was 3.4 deg. For the red rasters, each trial also has associated with it an indication of microsaccade onset and end times relative to the visual burst (horizontal lines; colors indicate whether the microsaccade was towards the RF or opposite it as per the legend). For all of the movements, the visual burst overlapped with at least parts of the movements. Error bars denote 95% confidence intervals, and the shaded region between 30 and 100 ms denotes our estimate of visual burst interval. There was no statistically significant difference between peak firing rate with and without microsaccades (p=0.67, t-test). The numbers of trials and microsaccades can be inferred from the rasters. (B) For the same example session in A, we plotted the mean radial amplitude (left) and mean radial eye velocity (right) for the microsaccades towards or opposite the RF in A. The black curves show baseline microsaccade amplitude and peak velocity (for movements occurring within 100 ms before stimulus onset). Movements towards the RF were increased in size when they coincided with a peripheral visual burst; our subsequent analyses provide a mechanism for this increase. Opposite microsaccades are also shown, and they were slightly truncated. Error bars denote s.e.m. (C) At the population level, we plotted peak firing rate with saccades detected during a visual burst (y-axis) or without saccades around the visual burst (x-axis). The different symbols show firing rate measurements in either experiment 1 (contrast task) or experiment 2 (spatial frequency task); all neurons from each experiment are shown. Note that some neurons were run on both tasks sequentially in the same session (Figure 1), resulting in a larger number of symbols than total number of neurons.
-
Figure 2—source data 1
Excel table with the source data for this figure.
- https://cdn.elifesciences.org/articles/64150/elife-64150-fig2-data1-v2.xlsx
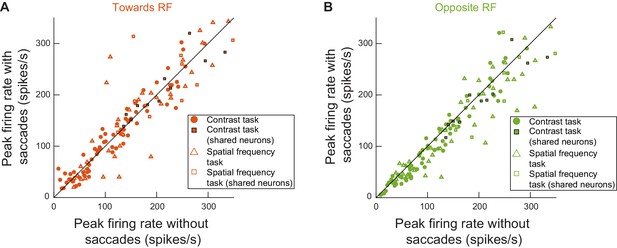
Visual bursts in the SC could happen intra-saccadically whether the movement being generated was towards the recorded neurons’ RF locations or opposite them.
Peak firing rate for all of the recorded neurons in experiments 1 and 2 when the visual burst was happening with or without microsaccades (similar analyses to Figure 2C, but now separating movement directions relative to RF locations; Methods). (A) Microsaccades towards RF locations. (B) Microsaccades opposite RF locations. Here, the peak firing rate was slightly reduced compared to the peak firing rate without microsaccades (t(134) = 4.6611, p<7.5045*10−6). Critically, for both A and B, the visual burst was still clearly present. Therefore, regardless of movement direction, intra-saccadic SC visual bursts could still occur. Like in Figure 2C, data from both experiments 1 and 2 are shown together, but with different symbols (see legend). Note that for statistics in this figure only, we pooled all measurements even if the same neuron contributed multiple measurements when it was run on both tasks. This is because modulations of visual bursts are secondary, from the perspective of the current study, to the fact that the visual bursts still happened regardless of microsaccade direction (also see Figures 8–12).
-
Figure 2—figure supplement 1—source data 1
Excel table with the source data for this figure.
- https://cdn.elifesciences.org/articles/64150/elife-64150-fig2-figsupp1-data1-v2.xlsx
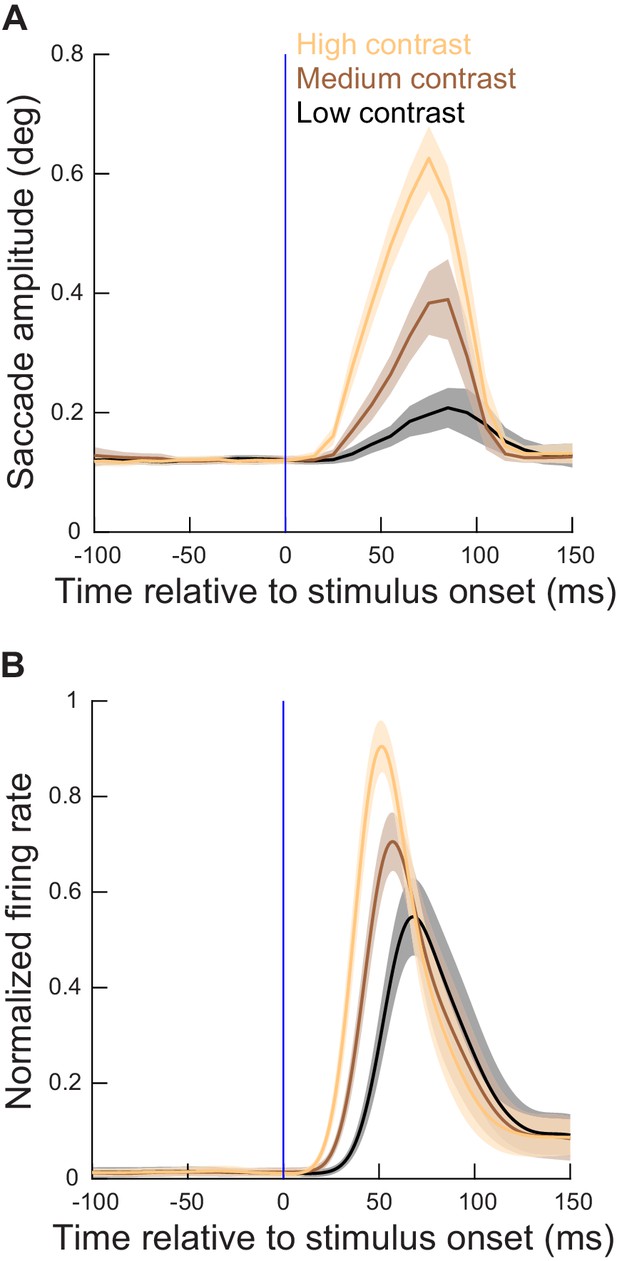
Microsaccade metrics were altered when the movements coincided with SC visual bursts, and the alteration was related to SC visual burst strength.
(A) Time course of microsaccade amplitude in the contrast task (experiment 1) relative to stimulus onset (for neurons with eccentricities ≤ 4.5 deg). The data were subdivided according to stimulus contrast (three different colors representing the three highest contrasts in our task). Movement amplitudes were small (microsaccades) in the baseline pre-stimulus interval, but they sharply increased after stimulus onset, reaching a peak at around 70–80 ms. Moreover, the metric alteration clearly depended on stimulus contrast. N = 288, 206, and 222 microsaccades for the highest, second highest, and lowest contrast, respectively. (B) Normalized firing rates relative to stimulus onset for the extra-foveal neurons (≤4.5 deg preferred eccentricity) that we recorded simultaneously in experiment one with the eye movement data in A. The alterations in movement metrics in A were strongly related, in both time and amplitude, with the properties of the SC visual bursts. Figure 3—figure supplement 1 shows the results obtained from more eccentric neurons and stimuli (>4.5 deg), and Figure 3—figure supplement 2 shows similar observations from the spatial frequency task (experiment 2). A subsequent figure (Figure 4—figure supplement 3) described the full dependence on eccentricity in our data. Error bars denote 95% confidence intervals.
-
Figure 3—source data 1
Excel table with the source data for this figure.
- https://cdn.elifesciences.org/articles/64150/elife-64150-fig3-data1-v2.xlsx
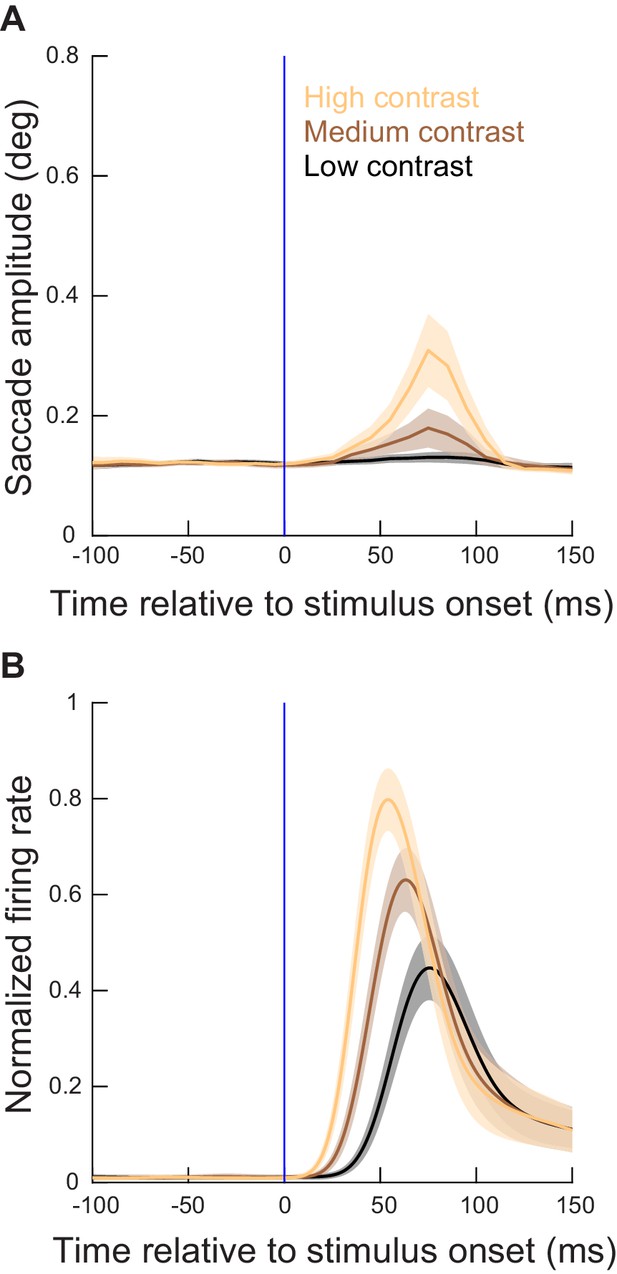
More eccentric stimuli relative to the generated movement amplitudes had weaker effects on metric alterations than the less eccentric stimuli of Figure 3.
(A) Time courses of microsaccade amplitudes relative to stimulus onset (from experiment 1) when the visual stimuli were presented at eccentricities > 4.5 deg (and <20 deg; Figure 1). N = 263, 289, and 458 microsaccades for the highest, second highest, and lowest contrast, respectively. The figure is otherwise formatted identically to Figure 3. As can be seen, there were weaker effects of more eccentric stimuli on microsaccades, even though the stimuli were made bigger to fill the RF’s (Materials and methods), and also even though the raw visual bursts showed similar properties to the more central neurons’ visual bursts (B and Figure 3—figure supplement 3). Also see Figure 4—figure supplement 3. (B) From the same experiment (contrast task), normalized firing rates of the more eccentric neurons relative to stimulus onset. The raw firing rates are shown in Figure 3—figure supplement 3, and, together with the current figure, they demonstrate that there was a weaker impact of more eccentric spiking activity on microsaccades; that is, the more eccentric bursts were similar in strength to the more central bursts, but they still had a smaller impact on microsaccade amplitudes in A. Note that, consistent with Hafed and Ignashchenkova, 2013; Buonocore et al., 2017; Malevich et al., 2020b, microsaccade amplitudes at the time of SC visual bursts were decreased relative to baseline (by a small amount) for movements that were opposite the stimulus locations (see Figure 6). This suggests that visual bursts opposite a planned movement might hamper the movement’s execution (Buonocore et al., 2017).
-
Figure 3—figure supplement 1—source data 1
Excel table with the source data for this figure.
- https://cdn.elifesciences.org/articles/64150/elife-64150-fig3-figsupp1-data1-v2.xlsx
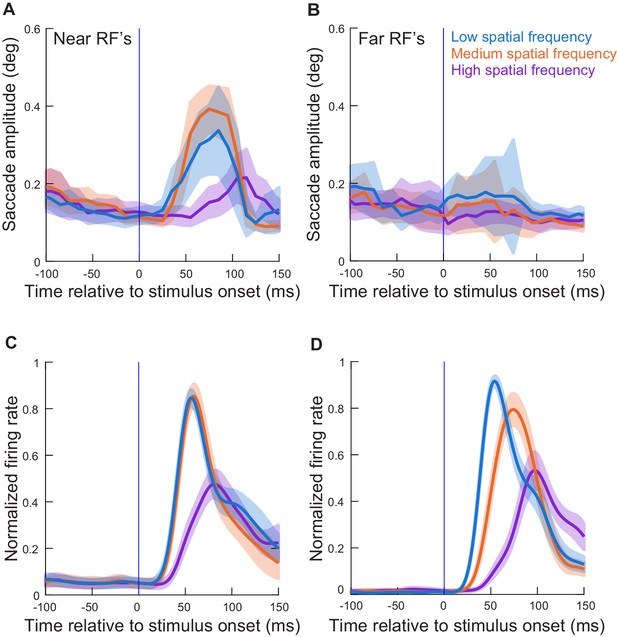
Results similar to those in Figure 3 and Figure 3—figure supplement 1 but with the spatial frequency task (experiment 2).
(A, C) Similar analyses to those in Figure 3A,B, but for the neurons recorded during the spatial frequency task (experiment 2). As in Figure 3A,B, the neurons here had preferred eccentricities ≤ 4.5 deg. (B, D) Similar analyses to A, C, but now for the neurons with preferred eccentricities > 4.5 deg. The impacts on microsaccade amplitudes were now much weaker, consistent with Figure 3—figure supplement 1. All other conventions are similar to Figure 3 and Figure 3—figure supplement 1. Note that in A, C, the amplitude effects were smaller than those in Figure 3, likely because the different stimulus types and sizes activated different numbers of overall neurons simultaneously (Figure 6 and Figure 6—figure supplement 1 show that per-neuron spike times relative to amplitude effects were highly similar across the two tasks).
-
Figure 3—figure supplement 2—source data 1
Excel table with the source data for this figure.
- https://cdn.elifesciences.org/articles/64150/elife-64150-fig3-figsupp2-data1-v2.xlsx
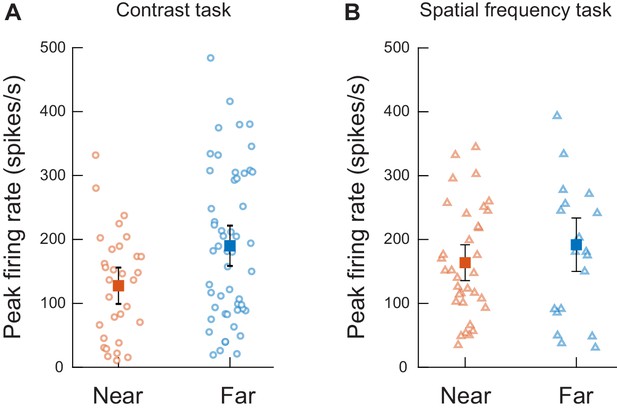
Despite smaller effects on microsaccade amplitudes (Figure 3—figure supplements 1 and 2), more eccentric visual bursts were not weaker than more central ones.
(A) Peak firing rate measurements from experiment one for neurons with an RF location ≤4.5 deg (Near, orange) or >4.5 deg (Far, blue) from fixation. Each dot represents one neuron. Solid squares represent the averages for each group. Error bars represents two standard errors of the mean. In this example (for clarity of the figure), the visual stimuli presented to the neurons were gratings with the second highest contrast only. N = 31 and 53 neurons, respectively, for the more central and more eccentric neurons (t(83) = −2.648 p=0.01). (B) Similar analyses and results, shown here only for the lowest spatial frequency (for clarity) from experiment 2. N = 34 and 21 neurons, respectively, for the more central and more eccentric neurons (t(53) = 1.20 p=0.23).
-
Figure 3—figure supplement 3—source data 1
Excel table with the source data for this figure.
- https://cdn.elifesciences.org/articles/64150/elife-64150-fig3-figsupp3-data1-v2.xlsx
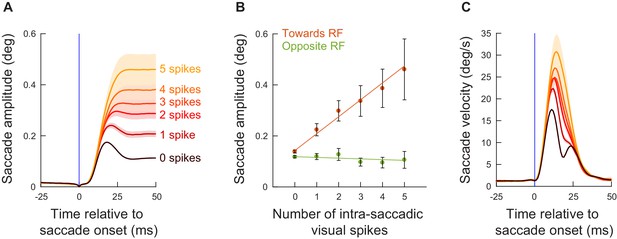
The number of exogenous, movement-unrelated ‘visual’ spikes to occur intra-saccadically linearly added to the executed movement’s amplitude.
(A) For every recorded neuron from experiment 1 (Figure 3A,B) and every microsaccade to occur near the visual burst interval (Figure 2), we counted the number of spikes recorded from the neuron that occurred intra-saccadically (0–20 ms after movement onset). We did this for movements directed towards the RF location (Figure 1C; Materials and methods). We then plotted radial eye position (aligned to zero in both the x- and y-axes) relative to saccade onset after categorizing the movements by the number of intra-saccadic spikes. When no spikes were recorded during the eye movement, saccade amplitudes were small (darkest curve). Adding ‘visual’ spikes into the SC map during the ongoing movements systematically increased movement amplitudes. Error bars denote s.e.m. (B) To summarize the results in A, we plotted mean saccade amplitude against the number of intra-saccadic ‘visual’ spikes for movements directed towards the RF locations (faint red dots). There was a linear increase in amplitude with each additional spike per recorded neuron (orange line representing the best linear fit of the underlying raw data). Even intra-saccadic spikes from visual neurons (more dissociated from the motor output of the SC than visual-motor neurons) were still associated with increased amplitudes (Figure 4—figure supplement 1). For movements opposite the RF locations (faint green dots and green line), there was no impact of intra-saccadic ‘visual’ spikes on movement amplitudes. The numbers of movements contributing to each x-axis value are 1772, 383, 237, 145, 113, and 78 (towards) or 1549, 238, 104, 63, 36, 23 (opposite) for 0, 1, 2, 3, 4, and 5 spikes, respectively. (C) For the movements towards the RF locations (A), peak radial eye velocities also increased, as expected (Buonocore et al., 2017). Error bars denote one standard error of the mean (A, C) and 95% confidence intervals (B). Figure 4—figure supplement 2 shows results for intra-saccadic spikes from more eccentric neurons (>4.5 deg), and Figure 4—figure supplement 3 shows the full dependence on neuronal preferred eccentricity. Finally, Figure 4—figure supplement 4 shows the same analyses of B but for the data from experiment 2.
-
Figure 4—source data 1
Excel table with the source data for this figure.
- https://cdn.elifesciences.org/articles/64150/elife-64150-fig4-data1-v2.xlsx
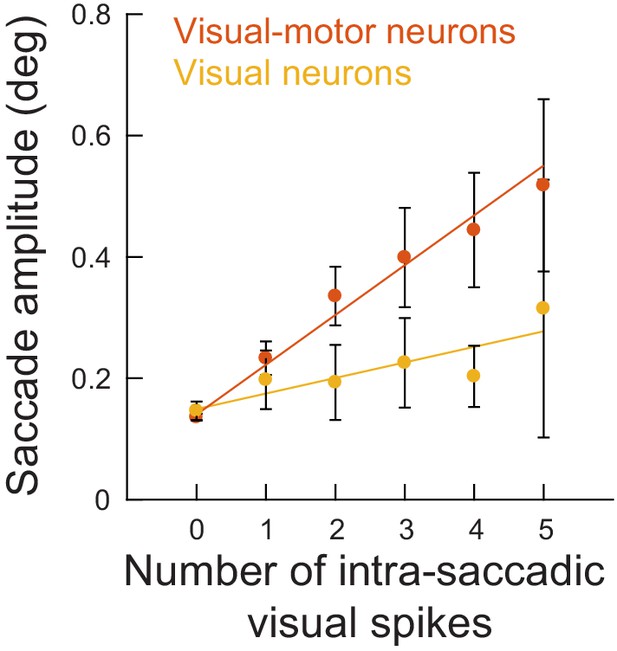
Same analysis as in Figure 4B (for movements toward RF’s), but separating visual and visual-motor neurons.
Even visual neurons (≤4.5 deg eccentricity), which are more dissociated from the SC motor output than visual-motor neurons, were still associated with an increase in microsaccade amplitude for injected intra-saccadic ‘visual’ spikes. The influence of visual-motor neurons was larger than the influence of visual neurons because the former are better connected to the SC’s motor output (Mohler and Wurtz, 1976); therefore, the correlation between any one such neuron and the global output behavior of the animal is expected to be larger (this is analogous to the concept of choice probability in other research fields Britten et al., 1996; Nienborg and Cumming, 2006). Error bars denote 95% confidence intervals.
-
Figure 4—figure supplement 1—source data 1
Excel table with the source data for this figure.
- https://cdn.elifesciences.org/articles/64150/elife-64150-fig4-figsupp1-data1-v2.xlsx
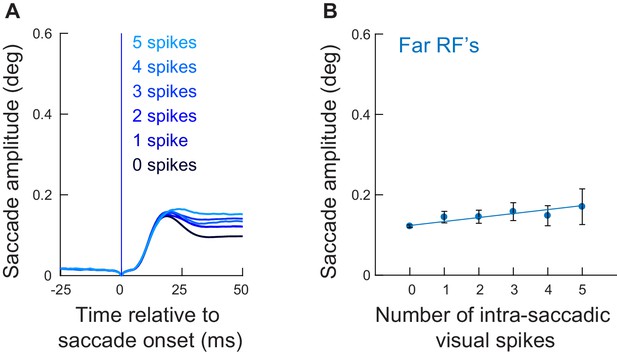
Intra-saccadic ‘visual’ spikes from more eccentric neurons in experiment one still linearly increased microsaccade amplitudes, but with a much weaker effect size.
(A) Radial eye position relative to saccade onset grouped by the number of ‘visual’ spikes counted in the interval 0–20 ms, for eye movements going towards the recorded neuron’s RF location (similar to Figure 4A). In this analysis, the RF was always located at an eccentricity >4.5 deg (Figure 1C). We used the same grouping and color conventions as in Figure 4A but in gradients of blue instead of red. When no spikes were recorded during the eye movement, saccade amplitudes were relatively small (darkest blue curve). Adding visual spikes in the SC map during the ongoing movements slightly increased their amplitudes (1–5, color-coded from dark to light blue), but the effect was much milder than for neurons closer in eccentricity to the foveal movement endpoints (Figure 4A). Note, however, that with proper temporal alignment of visual spikes with microsaccade onsets, even these more eccentric neurons could have a strong impact on microsaccade amplitudes (see Figure 6—figure supplement 2). (B) Mean saccade amplitude as a function of the number of intra-saccadic visual spikes (faint blue dots), similar in formatting to Figure 4B. There was a linear increase in amplitude relative to the number of injected visual spikes (blue line), similar to Figure 4B for the more central neurons. However, the slope of the effect was significantly lower (slope: 0.0098876; t = 6.7195, p=2.024−11). The solid lines represent the best linear fit of the underlying raw data. Error bars denote 95% confidence intervals. The numbers of movements contributing to each x-axis value are 3458, 684, 375, 244, 169, and 155 for 0, 1, 2, 3, 4, and 5 spikes, respectively.
-
Figure 4—figure supplement 2—source data 1
Excel table with the source data for this figure.
- https://cdn.elifesciences.org/articles/64150/elife-64150-fig4-figsupp2-data1-v2.xlsx
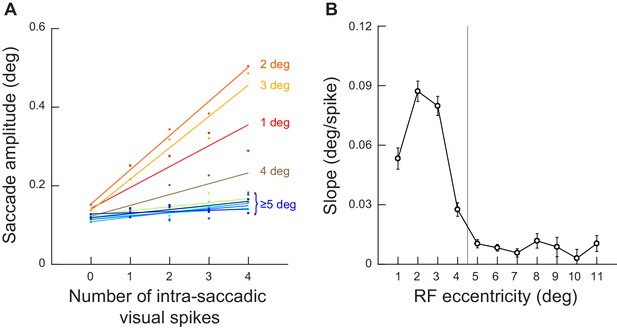
Injected visual spikes always increased microsaccade amplitudes, but the effectiveness was diminished with larger neuronal eccentricities.
(A) Analysis similar to that in Figure 4B (for the towards movements) but now for different neuronal preferred eccentricities (the different colors; Materials and methods). Visual spikes in neurons at eccentricities larger than approximately 4–5 deg had much lower slopes than visual spikes in more central neurons. Importantly, the slope of the relationship between injected intra-saccadic visual spikes and microsaccade amplitude was always positive across all of the tested eccentricities, meaning that there was still a positive impact of the more eccentric neurons, albeit weaker in magnitude (also see Figure 6—figure supplement 2). (B) The slopes of the curves in A now drawn as a function of neuronal preferred eccentricity (Materials and methods). There were diminishing returns with larger distances between neuronal preferred eccentricity and microsaccade amplitudes, but the slope was always positive. That is, even the most eccentric neurons were still associated with a modest impact in terms of increasing the executed movement amplitudes (an example is seen in Figure 4—figure supplement 2 and also in Figure 6—figure supplement 2). Also note how the curve of slope dependence on neuronal preferred eccentricity justifies our choice in most analyses to focus on eccentricities ≤ 4.5 deg. Error bars denote s.e.m.
-
Figure 4—figure supplement 3—source data 1
Excel table with the source data for this figure.
- https://cdn.elifesciences.org/articles/64150/elife-64150-fig4-figsupp3-data1-v2.xlsx
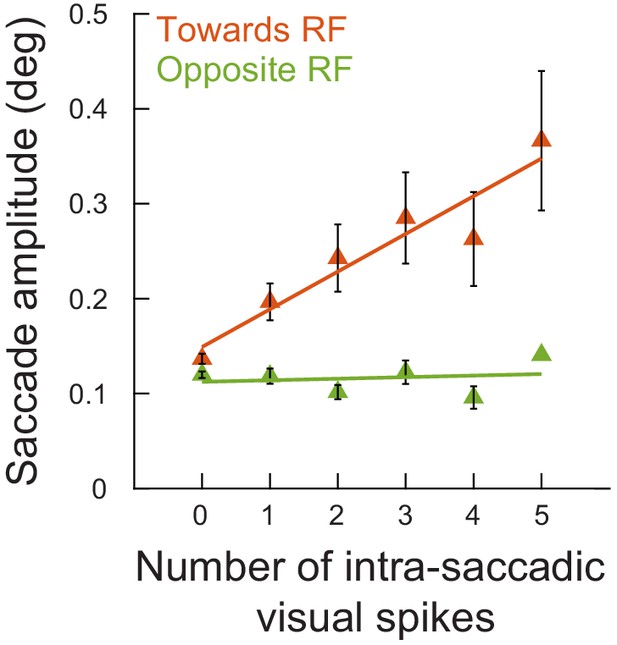
The analysis of Figure 4B but during the spatial frequency task (experiment 2).
This figure is identically formatted to Figure 4B, but this time showing results from experiment 2 (movements toward and away from the RF locations and for neurons ≤ 4.5 deg in preferred eccentricity). Very similar observations were made in both tasks. The numbers of movements contributing to each x-axis point are 403, 86, 36, 30, 18, and 15 microsaccades (towards) and 519, 81, 37, 21, 22, and one microsaccades (opposite) for 0, 1, 2, 3, 4, and 5 spikes, respectively.
-
Figure 4—figure supplement 4—source data 1
Excel table with the source data for this figure.
- https://cdn.elifesciences.org/articles/64150/elife-64150-fig4-figsupp4-data1-v2.xlsx
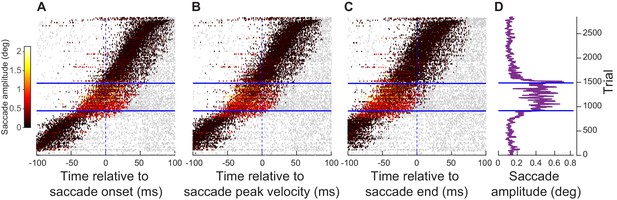
Exogenous, movement-unrelated SC spikes had the greatest impact on movement metrics when they occurred peri-saccadically.
(A) Individual trial spike rasters across all neurons ≤ 4.5 deg in eccentricity and all movements towards RF locations from experiment 1. The spike rasters are sorted based on the time of the visual burst (peak firing rate after stimulus onset) relative to saccade onset (bottom left: trials with visual bursts earlier than microsaccades; top right: trials with visual bursts later than microsaccades). The spike rasters are plotted in gray except during the interval 30–100 ms after stimulus onset (our visual burst interval; Figure 2) to highlight the relative timing of the visual burst to movement onset. Spikes in the visual burst interval are color-coded according to the observed movement amplitude on a given trial (legend on the left). As can be seen, microsaccades were enlarged when extra-foveal SC spiking (stimulus-driven visual bursts) occurred right before and during the microsaccades (see marginal plot of movement amplitudes in D). (B) Same as A, and with the same trial sorting, but with burst timing now aligned to movement peak velocity. (C) Same as A, B, and with the same trial sorting, but with burst timing now aligned to movement end. The biggest amplitude effects occurred when the exogenous ‘visual’ spikes occurred pre- and intra-saccadically, but not post-saccadically. (D) Microsaccade amplitudes (20-trial moving average) on all sorted trials in A–C. Blue horizontal lines denote the range of trials for which there was a significant increase in movement amplitudes (Materials and methods). Note that the numbers of trials are evident in figure. Figure 5—figure supplement 1 shows similar results from experiment 2, and Figure 5—figure supplement 2 shows similar results from the far neurons of experiment 1.
-
Figure 5—source data 1
Excel table with the source data for this figure.
- https://cdn.elifesciences.org/articles/64150/elife-64150-fig5-data1-v2.xlsx
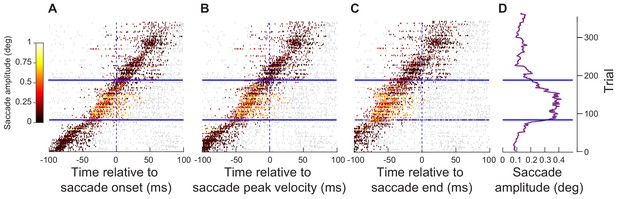
Analyses similar to those in Figure 5 but from experiment 2.
This figure is formatted similarly to Figure 5, but now using data from experiment 2 (the spatial frequency task; neurons ≤ 4.5 deg in preferred eccentricity). Very similar results can be seen.
-
Figure 5—figure supplement 1—source data 1
Excel table with the source data for this figure.
- https://cdn.elifesciences.org/articles/64150/elife-64150-fig5-figsupp1-data1-v2.xlsx
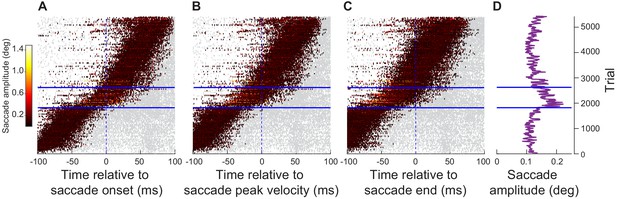
Analyses similar to Figure 5 but for the far neurons of experiment 1.
The same temporal relationship between the peripheral visual bursts and the microsaccade onset times existed for the movements that had increased amplitudes. The only difference in this case was that the overall behavioral impact on the movement amplitudes (D) was smaller than with the near visual bursts (consistent with Figure 3—figure supplement 1). Note that this could reflect a lower likelihood of proper temporal alignment of far peripheral visual spikes with the population motor bursts for microsaccades, according to the novel hypothesis of Jagadisan and Gandhi, 2019. Indeed, in Figure 6 and Figure 6—figure supplement 2, we found that with proper temporal alignment, the impacts of individual movement-unrelated spiking on microsaccade amplitudes were quantitatively similar for both near and far neurons.
-
Figure 5—figure supplement 2—source data 1
Excel table with the source data for this figure.
- https://cdn.elifesciences.org/articles/64150/elife-64150-fig5-figsupp2-data1-v2.xlsx
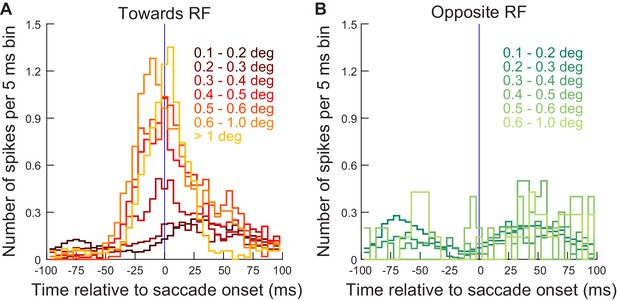
Exogenous, movement-unrelated ‘visual’ spikes affected movement metrics when they occurred within approximately ±30 ms from movement onset.
(A) For the different microsaccade amplitude ranges from Figure 5 (color-coded curves), we counted the number of exogenous spikes occurring from a recorded extra-foveal SC neuron (≤4.5 deg) within any given 5 ms time bin around movement onset (range of times tested: −100 ms to +100 ms from movement onset). The lowest two microsaccade amplitude ranges (0.1–0.2 and 0.2–0.3 deg) reflected baseline amplitudes during steady-state fixation (e.g. Figure 3), and they were not correlated with additional extra-foveal spiking activity around their onset (two darkest red curves). For all other larger microsaccades, they were clearly associated with precise timing of extra-foveal ‘visual’ spikes occurring within approximately ±30 ms from movement onset, regardless of movement size. The data shown are from experiment 1; similar observations were made from experiment 2 (Figure 6—figure supplement 1). The number of movements contributing to this figure is the same as in Figure 5. (B) Same as A but for movements opposite the recorded neuron’s RF locations. There were fewer spikes during the peri-saccadic interval, suggesting that it was easier to trigger eye movements when there was no activity present in the opposite SC. Figure 6—figure supplement 2 shows similar results from the far neurons (>4.5 deg eccentricity) of the same experiment (experiment 1).
-
Figure 6—source data 1
Excel table with the source data for this figure.
- https://cdn.elifesciences.org/articles/64150/elife-64150-fig6-data1-v2.xlsx
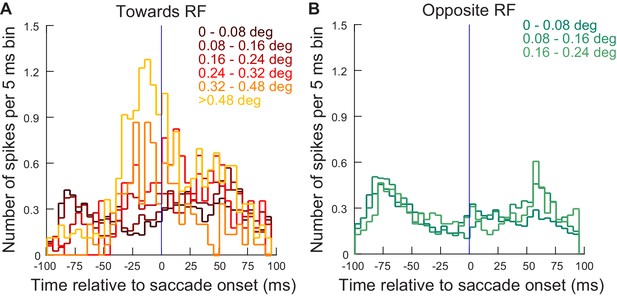
Same analysis as in Figure 6 but for the neurons recorded during experiment 2 (≤4.5 deg).
Very similar observations could be made.
-
Figure 6—figure supplement 1—source data 1
Excel table with the source data for this figure.
- https://cdn.elifesciences.org/articles/64150/elife-64150-fig6-figsupp1-data1-v2.xlsx
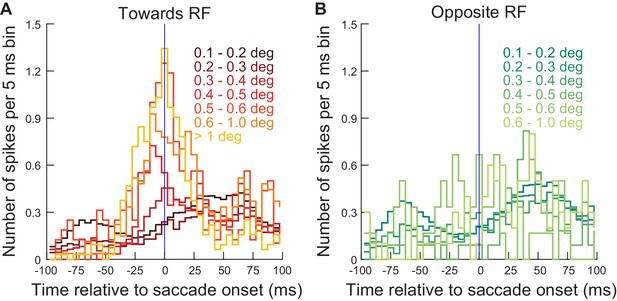
Same as Figure 6 but for the far neurons from experiment 1.
This figure is formatted similarly to Figure 6, but now using data from the far neurons of the same experiment (>4.5 deg eccentricity). Note how the same temporal alignment is seen as in Figure 6. Moreover, quantitatively, panel A shows that with proper temporal alignment, the same eye movement amplitude increases as in Figure 6A were expected to occur from the same numbers of injected ‘visual’ spikes, even with these far neurons with eccentricity >4.5 deg. Therefore, the weaker global behavioral effect with far stimuli (e.g. Figure 3—figure supplement 1) likely reflected more variability in the population temporal responses of the peripheral neurons’ visual bursts when compared to the central neurons’ visual bursts (see Discussion). Otherwise, within the proper time window, the spiking impact was similar in this figure as in the main Figure 6 with nearer neurons’ visual bursts.
-
Figure 6—figure supplement 2—source data 1
Excel table with the source data for this figure.
- https://cdn.elifesciences.org/articles/64150/elife-64150-fig6-figsupp2-data1-v2.xlsx
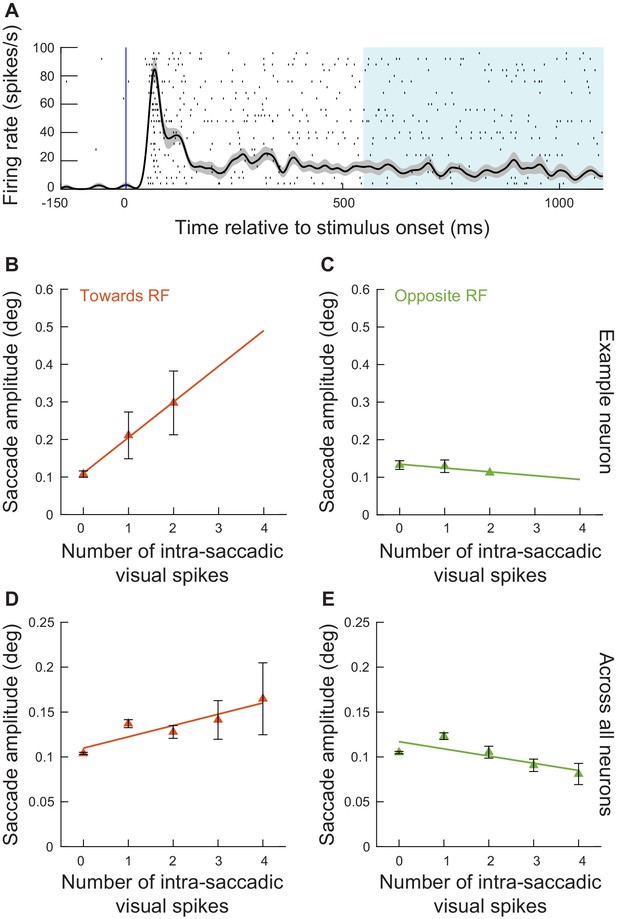
Exogenous, movement-unrelated spikes influenced eye movement metrics even when they did not occur within stimulus-driven ‘visual’ bursts.
(A) In experiment 2, we had a prolonged period of fixation after stimulus onset. This meant that there was low-level discharge present in the SC long after the end of the initial ‘visual’ burst, as shown in this example neuron (spike raster and average firing rate across trials with the preferred spatial frequency in the RF; error bars denote s.e.m.). This allowed us to select all microsaccades occurring >550 ms after stimulus onset, and to ask whether movement-unrelated SC spiking activity that was coincident with these movements still influenced their metrics. (B) For the example neuron in A and for microsaccades > 550 ms after stimulus onset and towards the RF, we performed an analysis like that of Figure 4B. There was a positive correlation between the number of intra-saccadic spikes and movement amplitude. Note that we combined trials with the lowest two spatial frequencies to increase the numbers of observations in this analysis. The numbers of movements contributing to each x-axis point are 62, 10, and 4 microsaccades for 0, 1, and 2 spikes, respectively. (C) Same as B but for movements opposite the RF from the same session. The numbers of movements contributing to each x-axis point are 77, 20, and 1 microsaccades for 0, 1, and 2 spikes, respectively. (D) Relationship between the number of intra-saccadic SC ‘sustained’ spikes (i.e. not part of ‘visual’ bursts) and microsaccade amplitudes for eye movements triggered >550 ms after grating onset from all sessions of experiment 2. We included trials from all spatial frequencies. For each microsaccade towards the RF location, we counted how many ‘sustained’ spikes were emitted by a given recorded neuron in the interval 0–20 ms after microsaccade onset. We then plotted microsaccade amplitude as a function of intra-saccadic ‘sustained’ spikes. Even when the spikes occurred outside of ‘visual’ bursts, they still had an influence on movement metrics. The numbers of movements contributing to each x-axis point are 4009, 747, 226, 62, and 26 microsaccades for 0, 1, 2, 3, and 4 spikes, respectively. (E) Same as D but for movements opposite the RF location. There was no increase in microsaccade amplitude. The numbers of movements contributing to each x-axis point are 4114, 721, 157, 45, and 16 microsaccades for 0, 1, 2, 3, and 4 spikes, respectively. Error bars in B–D denote 95% confidence intervals.
-
Figure 7—source data 1
Excel table with the source data for this figure.
- https://cdn.elifesciences.org/articles/64150/elife-64150-fig7-data1-v2.xlsx
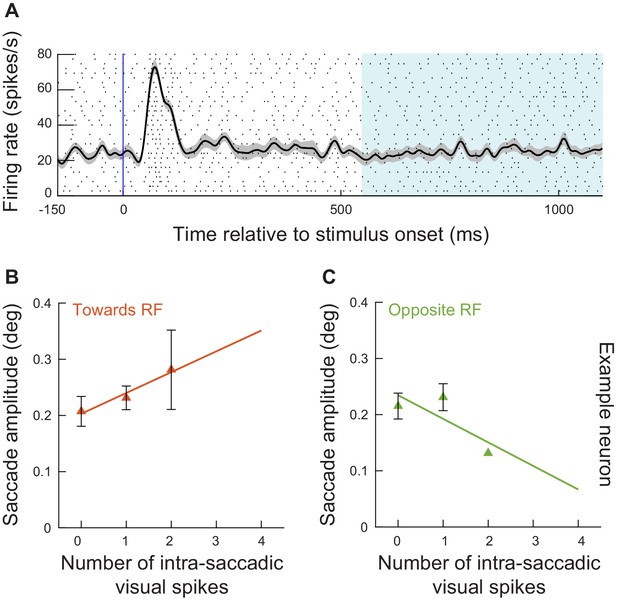
A second example neuron from experiment 2.
(A-C) This figure shows similar analyses to Figure 7A–C but for a second example neuron. Consistent results were observed on an individual neuron basis. The numbers of movements contributing to each x-axis point are 27, 52, and 5 microsaccades (B), or 42, 42, and 1 microsaccades (C) for 0, 1, and 2 spikes, respectively.
-
Figure 7—figure supplement 1—source data 1
Excel table with the source data for this figure.
- https://cdn.elifesciences.org/articles/64150/elife-64150-fig7-figsupp1-data1-v2.xlsx
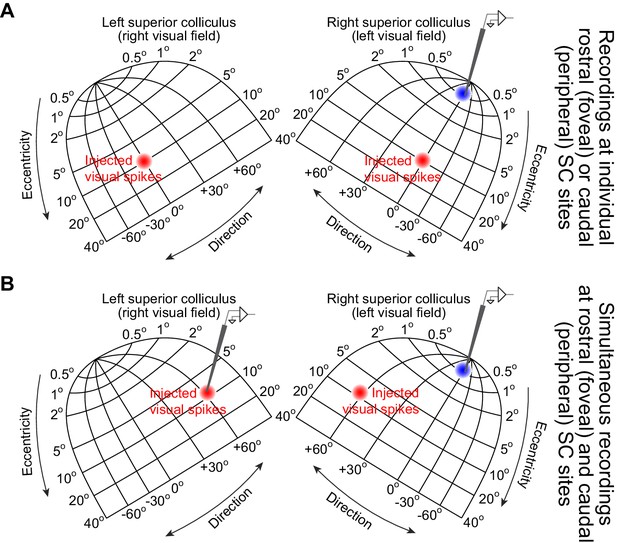
Exploring both movement-related and stimulus-driven SC discharge at the time of microsaccade triggering.
(A) We inserted microelectrode arrays into either the rostral SC (example shown in the right rostral SC) or the caudal SC. We then ran a behavioral fixation task in which the monkey fixated and a peripheral stimulus appeared on either side of fixation (Materials and methods). This meant that we injected ‘visual’ bursts in either the right or left caudal SC across trials (red), allowing us to measure either rostral SC or caudal SC activity when the injected ‘visual’ spikes occurred coincidentally with triggered microsaccades (same logic as in Figure 1). The caudal SC recordings were meant to support the earlier figures by demonstrating that intra-saccadic visual bursts could still occur in the SC; the rostral SC recordings were meant to investigate what happens to movement-related bursts at the time of the peripheral visual bursts. (B) In yet another set of experiments, and using the same behavioral task, we inserted two sets of microelectrode arrays simultaneously into both the rostral and caudal SC together. This allowed us to confirm the results from A using simultaneous rostral and caudal recordings. The shown topographic map of the SC is based on our earlier dense mappings, demonstrating both foveal (Chen et al., 2019) and upper visual field (Hafed and Chen, 2016) tissue area magnification.
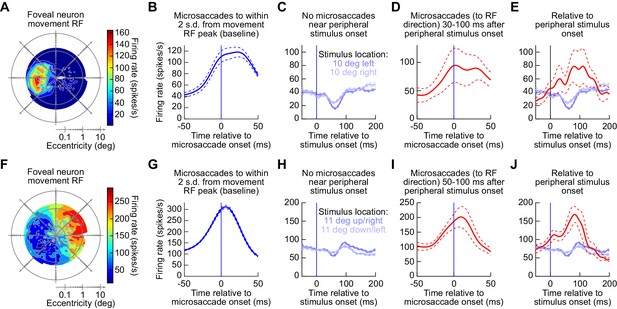
Rostral SC activity still exhibited bursts for microsaccades at the same time as caudal SC visual bursts.
(A) Example movement-related RF of a rostral SC neuron in the right SC (obtained from an RF mapping task; Materials and methods). Peak peri-microsaccadic firing rate is shown as a function of microsaccade radial amplitude and direction. Movement dimensions are plotted on log-polar axes (Hafed and Krauzlis, 2012), and the origin represents 0.03 deg radial amplitude. The neuron preferred leftward horizontal microsaccades. (B) After obtaining a movement RF like in A, we fitted the RF with a two-dimensional gaussian function (Materials and methods), and we then selected all microsaccades to a region within 2 s.d. of the fitted gaussian’s peak. We then plotted firing rates as a function of time from microsaccade onset, confirming movement-related discharge (Hafed et al., 2009; Willeke et al., 2019). (C) When a peripheral stimulus appeared in the main task of experiment three and no microsaccades occurred within −50–200 ms from stimulus onset, the neuron reduced its activity, consistent with earlier reports (Munoz and Istvan, 1998; Dorris et al., 2007; Hafed and Krauzlis, 2008). (D) However, the same neuron still exhibited a movement-related burst if the microsaccades towards its movement RF occurred within the visual burst interval associated with stimulus onset. (E) Thus, when aligned to peripheral stimulus onset, the neuron could either reduce its activity if microsaccades did not occur (blue curves), or it could increase its activity if microsaccades to the movement RF occurred (red). (F–J) Similar observations for a second example rostral SC neuron, this time in the left rostral SC. Note that for this particular example neuron, the visual burst interval that we picked was slightly modified because of a rarity of microsaccades of appropriate direction, but the same conclusions were reached as in the first example neuron (also see Figure 11). Error bars denote s.e.m.
-
Figure 9—source data 1
Excel table with the source data for this figure.
- https://cdn.elifesciences.org/articles/64150/elife-64150-fig9-data1-v2.xlsx
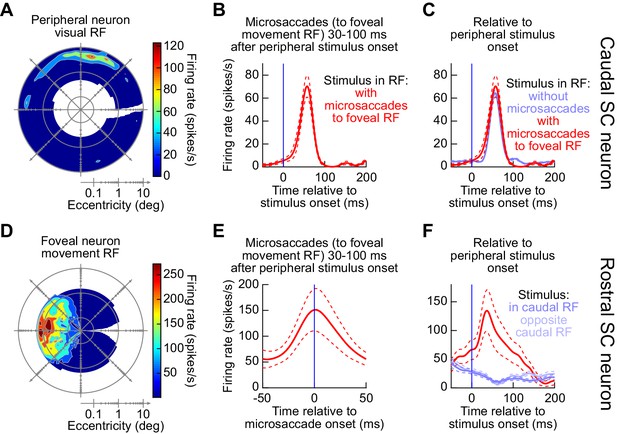
Simultaneous rostral and caudal.
SC recordings confirmed the simultaneity of peripheral visual bursts and foveal movement-related bursts when microsaccades were triggered around the time of peripheral visual bursts. (A) Visual RF of an example neuron from an experiment with both caudal and rostral microelectrode arrays inserted into the SC. This example neuron was recorded from the caudal array inserted into the left SC. The RF mapping task revealed a preferred eccentricity of ~6 deg. (B) The neuron still exhibited a robust visual response for a stimulus appearing inside its visual RF (at an eccentricity of ~6 deg) even when there were simultaneous microsaccades (towards the movement RF) occurring 30–100 ms after stimulus onset (i.e. coincident with the time of the visual burst). (C) For the same neuron, the visual burst was similar with and without microsaccades occurring within the visual burst interval. (D) A foveal movement-related RF of a simultaneously recorded neuron, this time from the second microelectrode array inserted into the right rostral SC. The shown map was obtained from the RF mapping task (Methods). (E) In the main task of experiment 3, for microsaccades towards the movement RF occurring within the visual burst interval (i.e. coincident with the visual burst in B), the neuron still exhibited a robust microsaccade-related discharge. (F) This means that relative to peripheral stimulus onset, this neuron actually had a burst (red) rather than a decrease (blue) in firing rate at the same time as the peripheral visual burst in the caudal SC (C). The blue firing rate curves show the same neuron’s response when the peripheral stimulus onset occurred in the absence of any microsaccades (same conventions as in Figure 9). Therefore, microsaccades occurring at the time of peripheral visual bursts were associated with readout of two burst loci: one in the rostral SC associated with the triggered movement and one in the caudal SC associated with visual stimulus onset. Error bars denote s.e.m.
-
Figure 10—source data 1
Excel table with the source data for this figure.
- https://cdn.elifesciences.org/articles/64150/elife-64150-fig10-data1-v2.xlsx
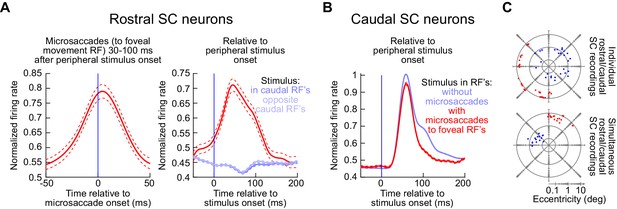
Population summary of the experiments in Figures 8–10.
(A) Left panel: movement-related firing rate for all rostral SC neurons when microsaccades towards the movement RF occurred 30–100 ms after peripheral stimulus onset (i.e. coincident with peripheral visual burst occurrence). For each neuron, we first calculated the microsaccade-related discharge for the preferred microsaccades in the RF mapping task and then divided by this maximum firing rate to normalize the activity of individual trials from the main task. We then averaged across all average normalized firing rates of individual neurons to obtain a population response (error bars denote s.e.m.). Right panel: When the same data were aligned to stimulus onset (as opposed to microsaccade onset), we could see that the rostral SC clearly exhibited bursts at the same time as peripheral visual bursts when microsaccades occurred (red). Figure 12 shows paired measurements of raw firing rates of all rostral neurons at the time of microsaccade onset, with or without peripheral visual bursts; it confirms that the rostral SC movement-related bursts were similar whether there was a peripheral visual burst or not. When microsaccades did not occur, peripheral stimulus onsets in either direction suppressed rostral SC activity (blue curves). (B) For all caudal SC neurons, we first averaged the firing rate across trials after a stimulus appeared inside the RF (again from the RF mapping task). We then normalized all trial firing rates by this measurement, and we then pooled neurons by averaging their individual normalized firing rate curves in the main task of experiment 3 (to obtain a population average response; error bars denote s.e.m.). Consistent with all of our earlier results, peripheral visual bursts still occurred even when coincident microsaccades occurred (red). Note that the red curve was slightly suppressed. This is because in most of our experiments (see C), the microsaccade site was opposite in direction from the caudal SC site. This is a condition known to be associated with suppressed visual bursts (Chen et al., 2015); also see Figure 2—figure supplement 1. (C) RF hotspot locations from all recording sites in these experiments (top: individual microelectrode array in either the caudal or rostral SC; bottom: simultaneous caudal and rostral SC recording arrays). The numbers of neurons are described in Materials and methods.
-
Figure 11—source data 1
Excel table with the source data for this figure.
- https://cdn.elifesciences.org/articles/64150/elife-64150-fig11-data1-v2.xlsx
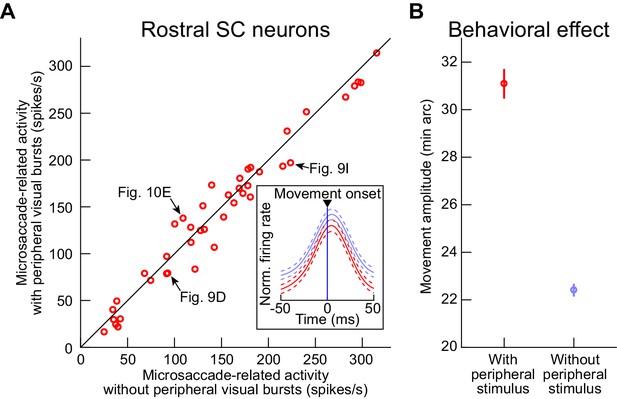
Similarity of microsaccade-related motor bursts at the time of peripheral visual bursts.
(A) For each rostral SC neuron from Figures 8–11, we measured the average firing rate in the interval within ±15 ms from microsaccade onset. We did this for microsaccades directed towards the rostral RF hotspot (Materials and methods). We then plotted this firing rate for the movements with which there was no peripheral stimulus onset (baseline microsaccades 500–1500 ms before stimulus onset; x-axis; Materials and methods) and also when the microsaccades occurred 30–100 ms after peripheral stimulus onset (that is, coincident with the peripheral visual bursts; y-axis). There was no statistically significant difference between the two measurements (p=0.12539; paired ranksum test; N = 42 neurons). The example rostral SC neurons from Figures 9 and 10 are highlighted with black arrows, and the inset shows the peri-movement population firing rates from all within-neuron paired measurements: red replicates the plot of Figure 11A, and blue shows the activity for baseline pre-stimulus microsaccades (error bars denote 95% confidence intervals). (B) For all of the sessions of experiment 3, we measured baseline microsaccade amplitudes and the amplitudes of microsaccades that were coincident with the peripheral visual bursts (occurring 30–100 ms after stimulus onset). In both cases, we picked microsaccades with directions towards the peripheral stimulus locations, because it is these microsaccades that are enlarged in size (e.g. Figures 2 and 3). Microsaccade amplitudes were significantly larger for the movements coincident with the peripheral visual bursts (p=7.9073×10−27; t-test; N = 650 microsaccades with peripheral stimulus onset, and N = 1956 baseline microsaccades). This occurred even though the peripheral stimuli were more eccentric than 4.5 deg, consistent with our earlier results (e.g. Figure 3—figure supplement 1 and Figure 6—figure supplement 2). Error bars denote s.e.m.
-
Figure 12—source data 1
Excel table with the source data for this figure.
- https://cdn.elifesciences.org/articles/64150/elife-64150-fig12-data1-v2.xlsx