The vascular niche controls Drosophila hematopoiesis via fibroblast growth factor signaling
Abstract
In adult mammals, hematopoiesis, the production of blood cells from hematopoietic stem and progenitor cells (HSPCs), is tightly regulated by extrinsic signals from the microenvironment called ‘niche’. Bone marrow HSPCs are heterogeneous and controlled by both endosteal and vascular niches. The Drosophila hematopoietic lymph gland is located along the cardiac tube which corresponds to the vascular system. In the lymph gland, the niche called Posterior Signaling Center controls only a subset of the heterogeneous hematopoietic progenitor population indicating that additional signals are necessary. Here we report that the vascular system acts as a second niche to control lymph gland homeostasis. The FGF ligand Branchless produced by vascular cells activates the FGF pathway in hematopoietic progenitors. By regulating intracellular calcium levels, FGF signaling maintains progenitor pools and prevents blood cell differentiation. This study reveals that two niches contribute to the control ofDrosophila blood cell homeostasis through their differential regulation of progenitors.
Introduction
In adult mammals, HSPCs in the bone marrow ensure the constant renewal of blood cells. The cellular microenvironment of HSPCs, called ‘niche’, regulates hematopoiesis under both homeostatic and immune stress conditions (Asada et al., 2017; Calvi et al., 2003; Calvi and Link, 2015; He et al., 2014; Kiel et al., 2005; Kobayashi et al., 2016; Morrison and Scadden, 2014; Zhao and Baltimore, 2015). Recent studies have revealed significant molecular and functional heterogeneity within the HSPC pool (for review Haas et al., 2018). These findings challenge the differential contribution of niche cell types to HSPC diversity. Given the high conservation of regulatory networks between insects and vertebrates, Drosophila has become an important model to study how hematopoiesis is controlled (Evans et al., 2003; Hartenstein, 2006). Insect blood cells, or hemocytes, are related to the mammalian myeloid lineage. In Drosophila, three blood cell types are produced: plasmatocytes that are macrophages involved in phagocytosis, crystal cells involved in melanisation and wound healing and lamellocytes required for encapsulation of pathogens too large to be destroyed by phagocytosis. Lamellocytes represent a cryptic cell fate since they only differentiate at the larval stage and in response to specific immune challenges such as wasp parasitism (Lemaitre and Hoffmann, 2007). The lymph gland is the larval hematopoietic organ and is composed of paired lobes, one pair of anterior lobes and several pairs of posterior lobes, aligned along the anterior part of the cardiac tube (CT) which corresponds to the vascular system (Figure 1a and Lanot et al., 2001). In third instar larvae, the anterior lobes comprise three zones: a medullary zone (MZ) containing hematopoietic progenitors, a cortical zone (CZ) composed of differentiated blood cells, and a small group of cells called the Posterior Signaling Center (PSC) (Figure 1a and Crozatier et al., 2004; Jung et al., 2005). The PSC produces a variety of signals that regulate lymph gland homeostasis (for review see Banerjee et al., 2019; Letourneau et al., 2016; Yu et al., 2018). Recently we established that cardiac cells produce the ligand Slit which, through the activation of Robo receptors in the PSC, controls the proliferation and clustering of PSC cells and in turn their function (Morin-Poulard et al., 2016). Furthermore, the MZ progenitor population is heterogeneous and a subset of progenitors called ‘core progenitors’ which express the knot/Collier (Kn/Col) and the thioester-containing protein-4 (tep4) genes is aligned along the cardiac tube and are maintained independently from the PSC (Figure 1a and Baldeosingh et al., 2018; Benmimoun et al., 2015; Oyallon et al., 2016). Altogether, these data led us to ask whether signals derived from cardiac cells are involved in the control of lymph gland homeostasis, i.e. the balance between progenitors and differentiated blood cells, independently from the PSC. To address this possibility we performed a candidate RNAi screen in cardiac cells to identify new potential signaling pathways involved in the crosstalk between the vascular and the hematopoietic organs.
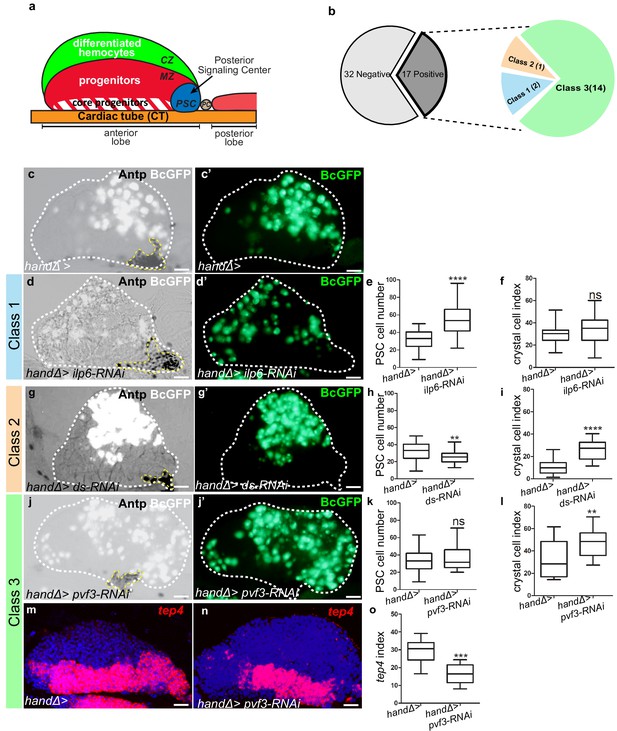
Lymph gland organization and RNAi screen results.
(a) Representation of lymph gland anterior and posterior lobes from third instar larvae. The anterior lobe is composed of progenitors (red) and core progenitors (hatched red), and the cortical zone (CZ, green). The PSC is blue and the cardiac tube (CT)/vascular system, is orange. PC corresponds to pericardial cell. (b) Summary of the screen performed by expressing RNAi in cardiac cells using the handΔ-gal4 driver. The number of genes corresponding to the different classes of phenotype is given. Subsequent panels illustrate the control and observed lymph gland defects (c, d, g, j). Anterior lobe and PSC are delimited by white and yellow dashed lines, respectively. Black-cell-GFP (BcGFP, white) labels crystal cells and Antp (black) the PSC. (c’, d’, g’, j’) BcGFP is in green; (e, h, k) PSC cell numbers; (f, i, l) Crystal cell index. (c–f) Reducing ilp6 in cardiac cells (d, d’) augments PSC cell number (e) without affecting crystal cell differentiation (f); this defines class 1. (g–i) Knocking down dachsous (ds) in cardiac cells (g, g’) decreases PSC cell number (h) and increases crystal cell index (i); this defines class 2. (j–l) Reducing pvf3 in cardiac cells (j, j’) does not modify PSC cell number (k) but increases crystal cell differentiation (l); this defines class 3. (m, n) tep4 (red) labels core progenitors. Decrease in tep4 expression is observed when pvf3 is knocked down in cardiac cells. (o) tep4 index. For all quantifications and figures, statistical analysis t-test (Mann-Whitney nonparametric test) was performed using GraphPad Prism five software. Error bars represent SEM and *p<0,1;**p<0,01; ***p<0,001; ****p<0,0001 and ns (not significant). In all confocal pictures nuclei are labeled with Topro (blue) and scale bars = 20 µm.
-
Figure 1—source data 1
Results of the RNAi ligand screen RNAi was expressed in cardiac cells by using the handΔ-gal4 and/or NP1029-gal4 driver.
Crystal cells were labeled by BcGFP, PSC cells were immune-stained with Antp antibody, and to visualize the core progenitors tep4 in situ hybridization was performed. In most cases, 2 RNAi lines were tested per ligand, and at least 15 lymph glands per RNAi were analyzed. Crystal cell index and PSC cell number were established. The green and red colored boxes indicate an increase and a decrease, respectively, compared to the control. Black dashes indicate that no difference was observed compared to the control. A white box indicates that this condition was not tested. Most RNAi lines that gave a modification in crystal cell index with the handΔ-gal4 driver were also analyzed with another cardiac cell driver NP1029-gal4, and proPO antibody immunostainings were performed to visualize crystal cells. Finally, for all RNAi lines that led to a defect in crystal cell differentiation with the handΔ-gal4 driver, tep4 in situ hybridizations were performed and the tep4 index was established.
- https://cdn.elifesciences.org/articles/64672/elife-64672-fig1-data1-v1.xlsx
-
Figure 1—source data 2
Results of the RNAi ligand screen.
- https://cdn.elifesciences.org/articles/64672/elife-64672-fig1-data2-v1.xlsx
Here we show that several signals produced by cardiac cells contribute to maintain lymph gland homeostasis. We investigated in more detail the role of the Fibroblast Growth Factor (FGF) ligand Branchless (Bnl). FGF signaling is conserved during evolution and is less complex in Drosophila than in humans. Ligand binding to a FGF receptor (FGFR) promotes its dimerization, which results in its tyrosine-phosphorylation, thus providing a scaffold to recruit different partners (Muha and Müller, 2013; Ornitz and Itoh, 2015). In mammals, ligand binding to the FGFR activates Ras/Raf-Mek-MAPK, PI3K/AKT, and PLCγ-Ca2+ signaling pathways (Turner and Grose, 2010).The Drosophila genome encodes two FGF receptors, Breathless (Btl) and Heartless (Htl), and three ligands, Bnl, Thisbe (Ths) and Pyramus (Pyr), (Beiman et al., 1996; Glazer and Shilo, 1991; Gryzik and Müller, 2004; Klämbt et al., 1992; Sutherland et al., 1996). Htl is activated by Ths and Pyr, while Btl is activated by Bnl. We established that Bnl is expressed in cardiac cells and signals to its receptor Breathless (Btl) expressed in progenitors. Bnl/Btl-FGF activation controls progenitor intracellular Ca2+ concentration, probably by activating Phospholipase Cγ (PLCγ) which regulates endoplasmic reticulum Ca2+ stores. Altogether, these data strongly support the conclusion that the cardiac tube plays a role similar to a niche by regulating lymph gland hematopoiesis.
Results
A cardiac screen identifies genes controlling lymph gland homeostasis
To investigate the role of cardiac cells in the control of lymph gland hematopoiesis, we performed a functional screen based on the expression, in cardiac cells, of RNAis directed against transcripts encoding known Drosophila ligands. For this we used the cardiac handΔ-gal4 driver which is expressed in cardiac cells throughout the three larval stages (Figure 1—figure supplement 1a–c’ and Monier et al., 2005; Morin-Poulard et al., 2016) to screen a collection of RNAi lines corresponding to 49 Drosophila ligands (Figure 1—source data 2). As read-outs, we analyzed blood cell differentiation with the crystal cell reporter BcGFP (Tokusumi et al., 2009), and PSC cell numbers and morphology by performing Antennapedia (Antp) immunostaining (Mandal et al., 2007). Compared to the control, 17 RNAi lines showed lymph gland homeostasis defects that were classified into three groups (Figure 1b). Class 1: Increased PSC cell numbers but no effect on crystal cell differentiation (Figure 1c–f). Two RNAis against ilp6 and spätzle4 transcripts belong to this class (source data). Class 2: Decreased PSC cell numbers and increased crystal cell differentiation (Figure 1g–i). Only one RNAi against dachsous (ds) belongs to this class (source data) Class 3: No effect on PSC cell numbers but increased crystal cell differentiation (Figure 1j–l); 14 RNAis belong to this class. The class three phenotype strongly suggested that signals from cardiac cells could control crystal cell differentiation independently from the PSC. We extended the analysis of the 14 corresponding genes by labeling the core progenitors with tep4 in situ hybridization (Krzemień et al., 2007). Reduced tep4 expression was observed for 12 RNAi treatments out of 14 (Figure 1m–o andsource data), indicating that the corresponding genes are required in cardiac cells to maintain tep4 expression in lymph gland progenitors and to prevent crystal cell differentiation. To avoid any bias due to the handΔ-gal4 driver, we also tested NP1029-gal4, an independent cardiac cell driver (Figure 1—figure supplement 1d–f’ and Monier et al., 2005; Morin-Poulard et al., 2016). Among the 14 RNAi candidates, nine gave a similar phenotype with both drivers (source data). In conclusion, our functional screen allowed us to identify nine ligands involved in communication between cardiac cells and hematopoietic progenitors to control lymph gland homeostasis.
The FGF ligand Bnl from cardiac cells controls lymph gland homeostasis
One candidate identified in our screen was Bnl. Previous studies have shown that Htl-FGF signaling is required during both early embryogenesis for lymph gland specification (Grigorian et al., 2013; Mandal et al., 2007) and in L3 larvae to control lymph gland progenitors (Dragojlovic-Munther and Martinez-Agosto, 2013). However, no role for bnl in the lymph gland has been described yet. Since bnl knock-down in cardiac cells significantly enhanced crystal cell differentiation in the lymph gland we decided to pursue an analysis of the Bnl-FGF pathway. Since Bnl is a diffusible ligand, we first documented bnl mRNA expression by in situ hybridization. bnl is expressed in cardiac and pericardial cells (Figure 2a–a’’), in agreement with previously published data (Jarecki et al., 1999). We also observed a weak bnl expression in MZ progenitors (as labeled by domeMESO >GFP in Figure 2a–a”), in differentiating hemocytes (as labeled by hml >GFP) and in a subset of crystal cells (marked by BcGFP) whereas no expression was detected in the PSC (Figure 2—figure supplement 1a–c”). In a heterozygous bnl loss-of-function mutant context where one copy of bnl (bnlP2/+) is missing, we observed an increased number of crystal cells compared to the control (Figure 2b–d). To specifically knock down bnl in cardiac cells, we expressed bnl-RNAi under the control of the cardiac tube specific driver handΔgal4. bnl loss-of-function experiments were performed from the L2 larval stage on, after the cardiac tube had formed, to avoid possible cardiac tube morphological defects (see MM and Figure 2—figure supplement 1d–e). bnl down-regulation in cardiac cells resulted in increased differentiation of both crystal cells and plasmatocytes (Figure 2e–f,i and Figure 2—figure supplement 1f–h). Increased crystal cell differentiation was also observed using another independent bnl-RNAi line (Figure 2—figure supplement 1i–k) and with the alternative NP1029-gal4 driver (Figure 2—figure supplement 1l–n). Applying bnl knockdown only after the L2 stage by using the GAL80 ts system (McGuire et al., 2004) led to a similar crystal cell differentiation defect (Figure 2—figure supplement 1o–q). We then analyzed MZ progenitors when bnl was knocked down in cardiac cells, using DomeMESO-RFP that labels all progenitors, and tep4 and Col that are expressed in the core progenitors (Krzemień et al., 2007; Oyallon et al., 2016). Compared to wild type, a reduced expression of the three markers was observed in handΔ>bnl-RNAi lymph glands (Figure 2j–r), indicating that Bnl from cardiac cells non-cell autonomously controls MZ progenitor maintenance. Altogether, these data indicate that Bnl produced in the cardiac tube acts in third instar larvae to control lymph gland homeostasis.
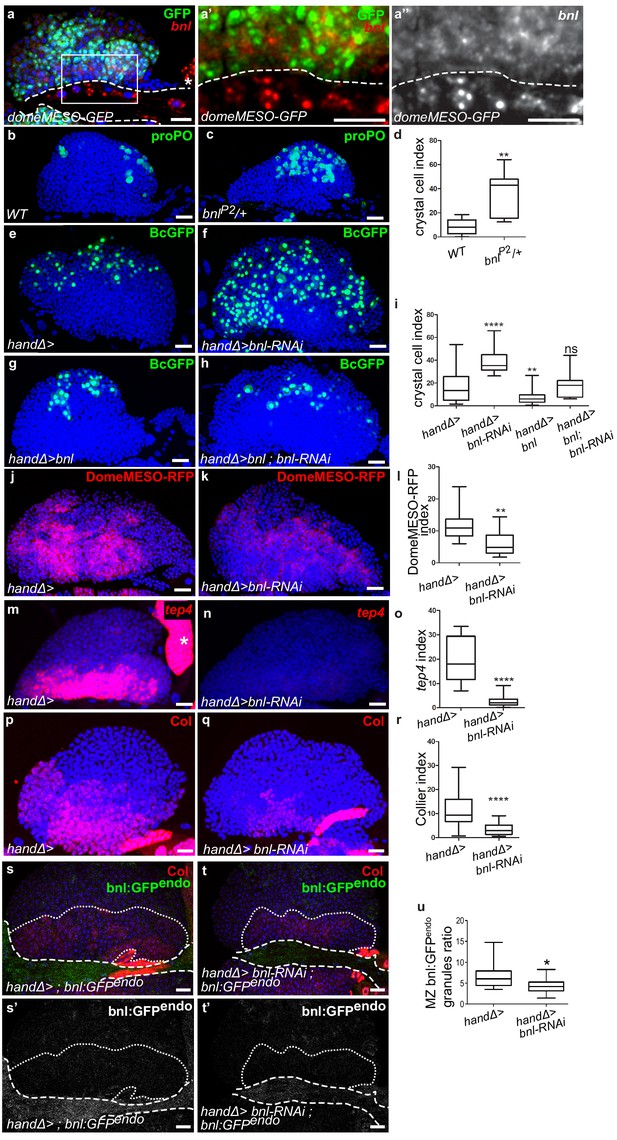
Ligand Bnl is expressed in cardiac cells and controls lymph gland homeostasis.
(a) A maximum projection of 5 confocal lymph gland sections, bnl (red) is expressed in cardiac cells and MZ progenitors that express domeMESO-GFP (green). (a’, a’’) An enlarged view, bnl is red (a’) or white (a”). A white dashed line indicates the cardiac tube. * indicates a pericardiac cell. (b, c) proPO (green) labels crystal cells. bnlP2/+ heterozygous mutant lymph glands have an increased number of crystal cells (c) compared to the control (b). (e–f, g–h) Black-cell GFP (BcGFP, green) labels crystal cells. (d, i) Crystal cell index. Co-expression of bnl and bnl-RNAi in cardiac cells restores the wildtype number of crystal cells (i). (j, k) DomeMESO-RFP (red) labels MZ progenitors. Compared to the control (j) barely detectable DomeMESO-RFP levels are observed when bnl is knocked down in cardiac cells (k). (l) DomeMESO-RFP index. (m, n) tep4 labels core progenitors. Compared to the control (m) lower levels of tep4 (red) are observed when bnl is knocked down in cardiac cells (n). (o) tep4 index. (p–q) Col labels core progenitors. Compared to the control (p) lower levels of Col are observed in the core progenitors when bnl is knocked down in cardiac cells (q). (r) Col index. (s–t’) Maximum projection of 5 confocal sections of the lymph gland expressing bnl:GFP endo (green) and Col immunostaining that labels MZ progenitors (red). Compared to the control (s, s’) a decrease in bnl:GFP endo in green (t) and white (t’) is observed when bnl is knocked down in cardiac cells. Fine and thick dashed lines indicate the MZ and CT contours, respectively. (u) Bnl:GFPendo granules ratio in the MZ.
-
Figure 2—source data 1
Quantification of Figure 2.
- https://cdn.elifesciences.org/articles/64672/elife-64672-fig2-data1-v1.xlsx
Since bnl is transcribed in MZ progenitors, though at low levels, we also analyzed its function in these cells. Reduction of bnl expression in progenitors (dome >bnl-RNAi) led to a significant increase in crystal cell differentiation as well as a decrease in tep4 expression (Figure 2—figure supplement 2a–f), indicating that Bnl produced by MZ progenitors is required to maintain their identity and to prevent their differentiation. Altogether, these data show that Bnl is produced by both MZ and cardiac cells, and that both sources are required in the control of lymph gland homeostasis.
To determine whether Bnl produced by cardiac cells contributes to the pool of Bnl present in the MZ, we analyzed endogenous Bnl distribution. We used the bnl:GFPendo knock-in allele that recapitulates bnl expression (Du et al., 2018). In agreement with in situ bnl detection, bnl:GFPendo was found in cardiac cells and in MZ progenitors (Figure 2s–s’). However, when bnl was knocked down only in the cardiac tube (handΔ>bnl-RNAi, bnl:GFPendo; Figure 2t–u) we overserved a reduction of bnl:GFPendo both in cardiac cells and MZ progenitors thus establishing that Bnl produced by cardiac cells contributes to the global MZ Bnl pool. The concomitant increased hemocyte differentiation suggests that Bnl levels contributed by the heart are required for lymph gland homoeostasis. To further support this conclusion, we overexpressed bnl only in cardiac cells (handΔ>bnl) which led to reduced crystal cell numbers (Figure 2i), and this confirms that the level of Bnl produced by cardiac cells controls hemocyte differentiation. Finally, rescue of crystal cell numbers (Figure 2g–i) and of progenitor marker expression (Figure 2—figure supplement 2g–i) was observed with a simultaneous expression of bnl and bnl-RNAi in cardiac cells (handΔ>bnl; bnl-RNAi). Altogether, these data establish that Bnl produced by cardiac cells is required for lymph gland hematopoiesis.
Since the PSC controls lymph gland cell differentiation (Benmimoun et al., 2015; Morin-Poulard et al., 2016; Oyallon et al., 2016; Tokusumi et al., 2010), we also looked at PSC cells by analyzing the expression of the PSC marker Antp (Mandal et al., 2007) when bnl was downregulated in cardiac cells. No PSC cell number or clustering defects were observed (Figure 2—figure supplement 2j–l). Hh expression in the PSC regulates progenitors and blood cell differentiation (Baldeosingh et al., 2018; Mandal et al., 2007; Tokusumi et al., 2010). The hhF4-GFP reporter transgene (Tokusumi et al., 2010) was expressed in PSC cells in the handΔ>bnl-RNAi context similar to the control (Figure 2—figure supplement 2m–o). These data strongly suggest that cardiac cell Bnl neither affects PSC cell numbers nor Hh activity, and likely acts directly on MZ progenitors to control lymph gland homeostasis. Altogether, these data indicate that although it is transcribed in many lymph gland cells, bnl expression in cardiac cells plays an essential role in the control of lymph gland homeostasis.
The FGF receptor Btl expressed in progenitors, controls lymph gland homeostasis
Bnl activates the FGF pathway by binding Btl (Kadam et al., 2009). To document endogenous Btl expression in the lymph gland, we used the btl:cherryendo knock-in allele which recapitulates Btl expression (Du et al., 2018). Strong btl:cherryendo expression was observed in cardiac cells and lower levels in MZ progenitors (as labeled by domeMESO-GFP in Figure 3a–a”). Whereas no expression was detected in PSC cells, a very faint expression occurs in a subset of crystal cells and in most differentiating blood cells (labeled by BcGFP and Hml >GFP, respectively, in Figure 3—figure supplement 1a–c”).
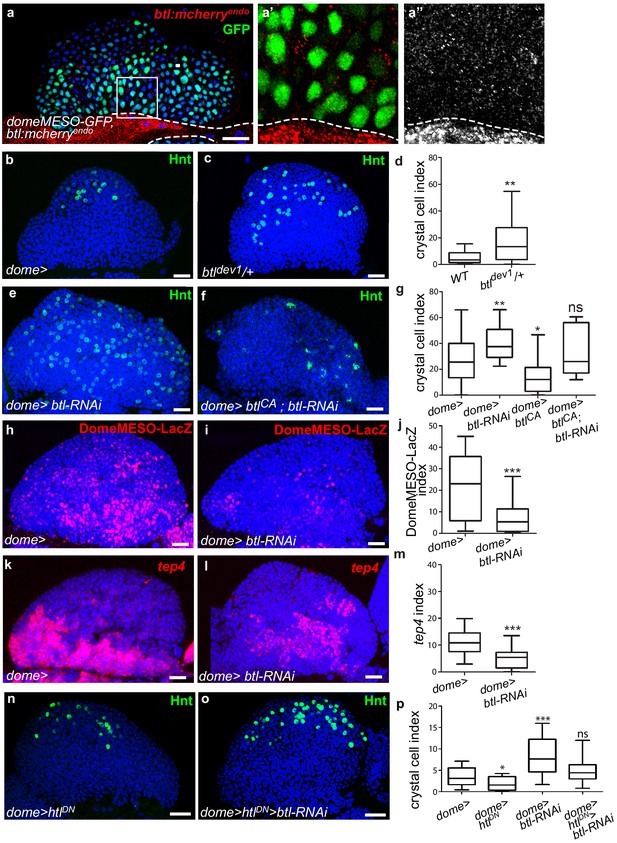
Receptor Btl is expressed in hematopoietic progenitors and required to control lymph gland homeostasis.
(a) A maximum projection of 5 confocal lymph gland sections of larvae expressing btl:cherryendo (red) and domeMESO-GFP that labels MZ progenitors (green). (a’, a’’) An enlarged view, btl:cherryendo red (a’) or white (a”). Dashed lines indicate the cardiac tube contour. btl:cherryendo is expressed in cardiac cells and MZ progenitors. (b–c, e–f) Hindsight (Hnt, green) labels crystal cells. Crystal cell differentiation is increased in btldev1/+ heterozygous mutant larvae (c) compared to the control (b). (e, f) Crystal cell numbers increase when btl is knocked down in progenitors (e) and crystal cell differentiation is rescued when a constitutive activated btl receptor (btlCA) is expressed in the btl-RNAi context (f). (d, g) Crystal cell index. (h, i) DomeMESO-LacZ (red) labels MZ progenitors. Compared to the control (h) barely detectable domeMESO-LacZ levels are observed when btl is knocked down in progenitors (i). (k, l) Lower levels of tep4 (red) are observed when btl is knocked down in progenitors (l) compared to the control (k). (j, m) DomeMESO-LacZ and tep4 index, respectively. (n, p) Crystal cell numbers decrease when a dominant negative htl receptor (htlDN) is knocked down in progenitors (n) and crystal cell differentiation is increased when htlDN is co-expressed with btl-RNAi (o). (p) Crystal cell index.
-
Figure 3—source data 1
Quantification of Figure 3.
- https://cdn.elifesciences.org/articles/64672/elife-64672-fig3-data1-v1.xlsx
To determine whether btl is required for lymph gland homeostasis, we looked at crystal cell differentiation in a heterozygous loss-of-function mutant context, where one copy of btl is mutated (btldev1/+). The resulting crystal cell index was higher than in controls (Figure 3b–d), revealing that btl controls lymph gland homeostasis. We then knocked down btl by expressing RNAi in either MZ progenitors (dome>) or cardiac cells (handΔ>). btl downregulation in cardiac cells did not significantly affect crystal cell numbers or MZ progenitors (Figure 3—figure supplement 1d–i). In contrast, knocking down btl in MZ progenitors led to increased crystal cell (Figure 3e,g) and plasmatocyte numbers (Figure 3—figure supplement 1j–l), together with a reduced expression of the two progenitor markers domeMESO-LacZ and tep4 (Figure 3h–m). We then performed rescue experiments with a constitutively active form of Btl (btlCA, Parés and Ricardo, 2016). The expression of btlCA in progenitors (dome >btlCA) led to reduced crystal cell numbers, i.e., a phenotype opposite to that of btl loss-of-function (Figure 3g). The co-expression of btlCA and btl-RNAi in progenitors (dome >btl-RNAi>btlCA; Figure 3f,g) rescued crystal cell differentiation, confirming that Btl is required in MZ progenitors. Finally, we examined whether PSC cells were affected when btl was knocked down in progenitors. No difference in PSC cell numbers or clustering was observed compared to the control (Figure 3—figure supplement 1m–o). We conclude that btl expression in MZ progenitors is required to control lymph gland homeostasis.
As opposed to Btl-FGF inhibition, Htl-FGF pathway knock-down in MZ progenitors was reported to block blood cell differentiation (Dragojlovic-Munther and Martinez-Agosto, 2013). To investigate the relationship between the two pathways in the MZ, we performed epistasis experiments. Expression of a dominant–negative form of Htl (dome >HtlDN) in progenitors led to a decrease in crystal cell differentiation, in agreement with a previous report (Dragojlovic-Munther and Martinez-Agosto, 2013). Simultaneous expression of HtlDN and btl-RNAi (dome >HtlDN > btl-RNAi) restored a wildtype number of crystal cells (Figure 3n–p). These data indicate that there is no hierarchy between Btl-FGF and Htl-FGF pathways. They also suggest that their simultaneous activity in MZ progenitors ensures a robust regulation of hemocyte differentiation.
In conclusion, downregulating Btl in MZ progenitors causes a defect in lymph gland homeostasis similar to that caused by Bnl downregulation in cardiac cells. This strongly suggests that Bnl/Btl-FGF signaling mediates inter-organ communication between MZ progenitors and the vascular system. This leads us to propose that by acting directly on MZ progenitors, the cardiac tube plays a role similar to a niche.
Bnl secreted by cardiac cells is taken up by lymph gland progenitors
Bnl originating from cardiac cells and acting on MZ progenitors raised the question of its mode of diffusion. To investigate this question, we expressed a functional GFP-tagged version of Bnl (UAS-Bnl::GFP, Lin, 2009) in cardiac cells. In addition to the expected GFP detection in these cells, discrete GFP positive cytoplasmic punctate dots/granules were detected in MZ progenitors (Figure 4a–a’’), indicating that Bnl::GFP can propagate from cardiac to lymph gland cells. Many cytoplasmic Bnl-GFP positive punctate dots in the MZ were Btl:Cherry positive (Figure 4b–b”). To further characterize these Bnl-GFP dots, we labeled recycling vesicles and late endosomes, using the ubi-Rab11-cherryFP reporter and Rab7 immunostaining, respectively. We found that many Rab11-positive and Rab7-positive vesicles co-localized with Bnl-GFP in the MZ (Figure 4c–d”). The simplest explanation is that Bnl::GFP secreted by cardiac cells is internalized by MZ progenitors, likely through receptor-mediated endocytosis.
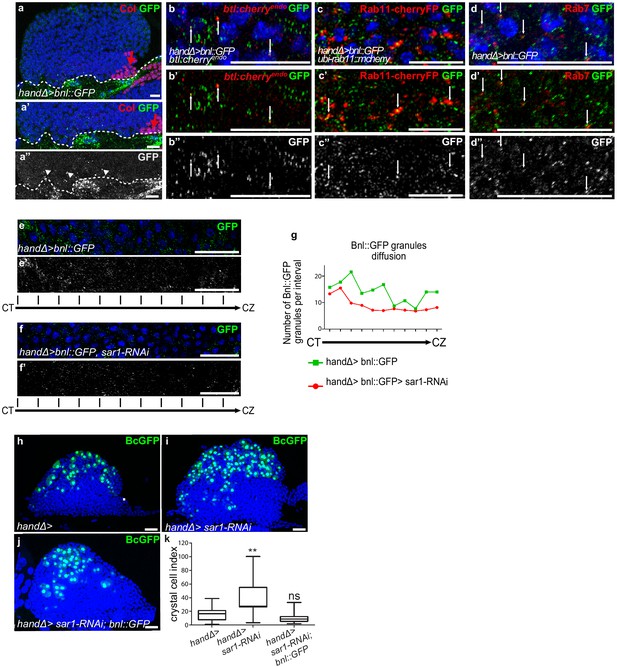
Ligand Bnl secreted by cardiac cells controls lymph gland crystal cell differentiation.
(a) Active bnl::GFP fusion protein is expressed in cardiac cells using handΔ-gal4 driver. Dashed lines indicate cardiac tube and the PSC is labeled by Collier (Col, red and red arrow). (a’, a’’) An enlarged view; Bnl::GFP is green (a’) or white (a”). Bnl::GFP positive granules are detected in cardiac and lymph gland cells (arrowheads). (b–b”) Enlargement of MZ area close to the cardiac tube in larvae expressing Bnl::GFP fusion protein (green) in cardiac cells (handΔ-gal4 > Bnl::GFP) and Btl:mcherryendo (red). Bnl::GFP cytoplasmic punctate dots (green in b-b’ and white in b’) co-localize with Btl:mcherryendo (yellow and arrows in b’). (c,c”) Enlargement of MZ area close to the cardiac tube in larvae expressing ubi-Rab11cherryFP (red), a marker for recycling endocytic vesicles; Bnl::GFP fusion protein (green) is expressed in cardiac cells (handΔ-gal4 > Bnl::GFP). Bnl::GFP cytoplasmic punctate dots (green in c-c’ and white in c’) co-localize with ubi-Rab11cherryFP (yellow and arrows in c’). (d–d”) Enlargement of MZ area close to the cardiac tube in larvae expressing Bnl::GFP fusion protein (green) in cardiac cells (handΔ-gal4 > Bnl::GFP) and Rab7 immunostainings (red in d, d’ and white in d’). (d–d’) Bnl::GFP cytoplasmic punctuate dots co-localize with Rab7 positive dots (yellow and arrows in d’). (e–f’) Enlargement of lymph gland cross sections extending from the cardiac tube (CT) to the cortical zone (CZ). Bnl::GFP fusion protein, expressed in cardiac cells (handΔ-gal4 > Bnl::GFP) is green (e, f) and white (e’, f’). Knocking down sar1 in cardiac cells (f, f’) leads to a decrease in Bnl::GFP cytoplasmic punctate dots compared to the control (e, e’). (g) Quantification of Bnl::GFP cytoplasmic punctate dots/granules. (h, j) BcGFP (green) labels crystal cells. Knocking down sar1 in cardiac cells (i) increases crystal cell numbers compared to the control (h). Crystal cell differentiation rescue is observed when bnl::GFP is co-expressed with sar1-RNAi (j, k). (k) Crystal cell index.
-
Figure 4—source data 1
Quantification of Figure 4.
- https://cdn.elifesciences.org/articles/64672/elife-64672-fig4-data1-v1.xlsx
To further confirm the role of Bnl secreted by cardiac cells, we impaired endoplasmic reticulum (ER) vesicle formation by knocking down the Secretion-associated Ras-related GTPase1 (Sar1) specifically in cardiac cells. The Sar1GTPase plays a key role in the biogenesis of transport vesicles and acts by regulating vesicular trafficking (Saito et al., 2017; Yorimitsu et al., 2014). The simultaneous expression of sar1-RNAi and Bnl::GFP in cardiac cells using the handΔ-gal4 driver (handΔ>bnl::GFP >sar1 RNAi) resulted in reduced bnl::GFP cytoplasmic punctate dots in MZ progenitors compared to the control (Figure 4e–g), further confirming that cardiac cells secrete Bnl. Previous data had established that the Slit ligand, produced by cardiac cells, activates Robo receptors in the PSC and as a consequence controls PSC cell proliferation and clustering (Morin-Poulard et al., 2016). Consistent with the impairment of cardiac cell secretion when sar1 is knocked down in cardiac cells (handΔ>and NP1029>sar1 RNAi, Figure 4—figure supplement 1a–f), we also observed an increase in PSC cell numbers as well as a slight defect in their clustering, likely an effect of Slit/Robo impairment. These data indicate that sar1 knock-down in cardiac cells impairs their secretory capacity and therefore Bnl secretion.
We then analyzed the consequences on lymph gland blood cell differentiation. When sar1 was knocked down in cardiac cells using handΔ or NP1029 drivers (Figure 4h–i,k and Figure 4—figure supplement 1g–i) crystal cell numbers were higher than the control, indicating that cardiac cell secretion capacity is necessary for lymph gland hematopoiesis. To determine whether the overexpression of bnl in cardiac cells can compensate for decreased secretion, we performed rescue experiments. Crystal cell differentiation was improved when sar1-RNAi and bnl::GFP were simultaneously expressed in cardiac cells (Figure 4j,k). In conclusion, these results indicate that Bnl secreted by cardiac cells is likely taken up by MZ progenitors to activate Btl-FGF signaling, which in turn regulates lymph gland homeostasis.
FGF activation in progenitors regulates their calcium levels
The next step was to address how Bnl/Btl-FGF signaling in progenitors controls lymph gland homeostasis. Depending on the cellular context, the FGF pathway activates the MAPK or PI3K pathways, or PLCγ that controls intracellular Ca2+ levels (Ornitz and Itoh, 2015). Inactivation of MAPK or PI3K in lymph gland progenitors leads to a phenotype opposite to that of knock-down of btl in progenitors or of bnl in cardiac cells (Dragojlovic-Munther and Martinez-Agosto, 2013). This strongly suggests that Bnl/Btl-FGF signaling in progenitors does not involve MAPK or PI3K activity. It was previously reported that intracellular Ca2+ levels regulate hematopoietic progenitor maintenance: reduction of cytosolic Ca2+ in lymph gland progenitors leads to the loss of progenitor markers and to increased blood cell differentiation (Shim et al., 2013). Since Bnl/Btl-FGF knock-down and reduction of Ca2+ in progenitors induce similar lymph gland defects, we asked whether both mechanisms were functionally linked. We investigated Ca2+ levels within MZ progenitors using the Ca2+ sensor GCaMP3, which emits green fluorescence only with high Ca2+levels (Nakai et al., 2001). This sensor is expressed under the control of the dome-gal4 driver. In agreement with previous reports, high Ca2+ levels were detected in MZ progenitors (Figure 5a and Shim et al., 2013). Knocking down btl in MZ progenitors starting from L1 stage led in third instar larvae to decreased fluorescence compared to the control, indicating a reduction in Ca2+ levels (Figure 5a–c). Since no difference with control larvae could be observed at L2 stage (Figure 5—figure supplement 1a–c), we conclude that the Bnl/Btl-FGF pathway is not required for MZ progenitor specification but is required in third instar larvae to regulate Ca2+ levels. We then asked whether restoring high Ca2+ levels in progenitors could rescue the lymph gland defects due to reduced Bnl/Btl-FGF function. When free Ca2+ was high within the progenitors, such as following overexpression of Calmodulin dependent kinase II (CaMKII; dome >CaMKII) or of IP3R (Tep4 >UAS-IP3R) which controls ER-mediated Ca2+ release into the cytosol (Shim et al., 2013), a significant decrease in crystal cell numbers was observed compared to the control (Figure 5d–h and Figure 5—figure supplement 1d–h). This indicates that high Ca2+ levels in progenitors inhibit blood cell differentiation, which is in agreement with previously published data (Shim et al., 2013). Simultaneous btl reduction and Ca2+ increase in progenitors, through CaMKII or IP3R overexpression (dome >CamKII;btl-RNAi Figure 5g–h and tep4 >IP3R>btl-RNAi; Figure 5—figure supplement 1g–h), leads to a decrease in crystal cell numbers compared to the sole btl-RNAi knockdown. Overall, these data suggest that in MZ progenitors, regulation of Ca2+ levels functions downstream of the Bnl/Btl-FGF pathway. In vertebrates, FGF activation can recruit and activate PLCγ, which induces Ca2+ release from the ER (Ornitz and Itoh, 2015). small wing (sl) encodes the sole Drosophila PLCγ homolog (Thackeray et al., 1998). To investigate the role of PLCγ in the lymph gland, we analyzed crystal cell differentiation in strong hypomorphic sl2 mutants. Compared to the control, increased crystal cell differentiation was observed (as labeled by Hnt Figure 5j,m). We then addressed the role of PLCγ specifically in progenitors. Knocking down PLCγ in progenitors (dome >sl-RNAi) led to increased crystal cell differentiation compared to the control (Figure 5i,l), revealing that PLCγ in MZ progenitors regulates lymph gland hemocyte differentiation. Finally, we performed epistasis experiments to decipher the relationship between sl and the Btl-FGF pathway. When btlCA was expressed in progenitors in a sl2 mutant context (sl2; dome >bltCA; Figure 5k,n), the crystal cell index was similar to that in the sl2 mutant alone. These data establish that sl acts downstream of the Bnl/Btl-FGF pathway. Altogether, our data support the hypothesis that in MZ progenitors, Bnl/Btl-FGF signaling leads to the activation of PLCγ, which controls Ca2+ levels and in turn hemocyte differentiation.
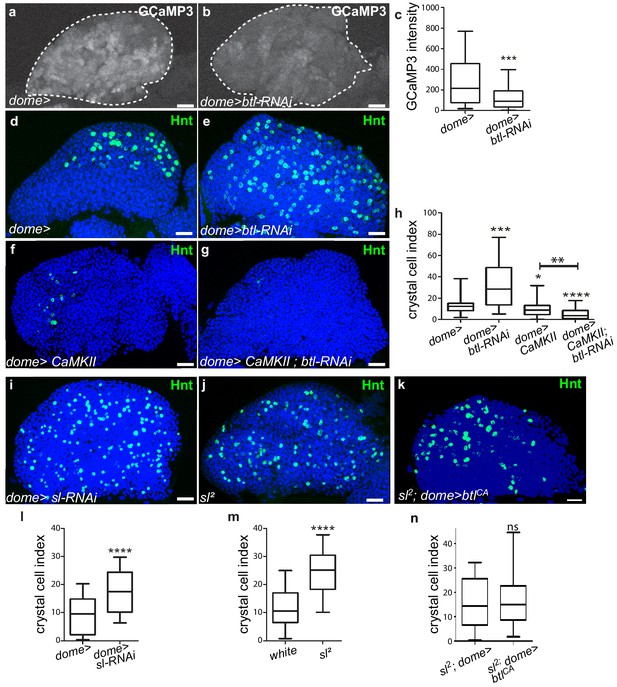
Btl receptor interacts genetically with CamKII to control blood cell differentiation by preventing high Ca2+ levels in progenitors.
(a, b) GCaMP3 Ca2+ sensor (dome >UAS-GCaMP3) is white. GCaMP3 intensity decreases when btl is knocked down in MZ progenitors (b) compared to the control (a). (c) Quantification of GCaMP3 intensity. (d–g, i–k) Hnt (green) labels crystal cells. Crystal cell differentiation decrease is observed when Ca2+ levels increased due to CaMKII expression in progenitors (dome >CaMKII, f) compared to the control (d). Co-expression of CaMKII and btl-RNAi in progenitors (dome >CaMKII; btl-RNAi, g) leads to a decrease in crystal cell number compared to the btl knock-down alone (e). (h, l–n) Crystal cell index. Crystal cell differentiation increase is observed in sl2 homozygous mutant larvae (j, m) and when sl is knocked down in progenitors (i, l) compared to the control (d). (k, n) No difference in crystal cell index is observed in sl2 homozygous mutant larvae and in a sl2 homozygous mutant where btlCA is expressed in MZ progenitors (sl2; dome >btlCA).
-
Figure 5—source data 1
Quantification of Figure 5.
- https://cdn.elifesciences.org/articles/64672/elife-64672-fig5-data1-v1.xlsx
Discussion
The control of HSPCs by a specific microenvironment called ‘niche’ is established both in mammals and in Drosophila. The niche is defined by its capacity to directly regulate, through signals, stem cells and progenitors. In the mammalian bone marrow HSPCs are under the control of the endosteal and vascular niches (Asada et al., 2017; Calvi et al., 2003; Calvi and Link, 2015; He et al., 2014; Kiel et al., 2005; Morrison and Scadden, 2014). In Drosophila, lymph gland studies have so far concentrated on the PSC acting as a niche. However, a subset of lymph gland progenitors (core progenitors), which express Col and tep4 and are aligned along the cardiac tube, is maintained in the lymph gland even when the PSC function is impaired, suggesting that other signals alongside those from the PSC are required (Baldeosingh et al., 2018; Benmimoun et al., 2015; Oyallon et al., 2016). Here, we report that cardiac cells play a role similar to a niche, since they directly control core progenitor maintenance. We show that communication between the vascular system and the lymph gland involves Bnl/Btl-FGFsignaling. Bnl secreted by cardiac cells activates Bnl/Btl-FGF in progenitors, which in turn controls hemocyte homeostasis. Our data indicate that Bnl/Btl-FGF signaling regulates lymph gland homeostasis by controlling calcium levels in progenitors via PLCγ activation (Figure 6). In a previous study, we showed that signals from the cardiac tube, namely Slit, can act on the PSC, but that no cellular communication between the cardiac tube and MZ progenitors is involved (Morin-Poulard et al., 2016). Now we establish that cardiac cells regulate the extent of progenitor differentiation in the lymph gland. Therefore, two separate niches (the PSC and the cardiac tube) control lymph gland homeostasis. While the PSC acts only on a subset of MZ progenitors (Baldeosingh et al., 2018; Oyallon et al., 2016), the cardiac tube directly regulates core progenitors and in turn all MZ progenitors (Figure 6).The identification of two niches that differentially regulate lymph gland progenitors sheds further light on the parallels existing between Drosophila lymph gland and mammalian bone marrow hematopoiesis.
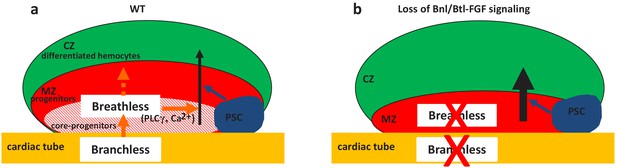
Two niches control lymph gland homeostasis.
(a–b) Schematic representation of third instar larvae lymph gland anterior lobes. Progenitors and core progenitors are in red and hatched red, respectively. The cortical zone (CZ) is in green, the PSC and the cardiac tube (CT)/vascular system are in blue and orange, respectively. (a) In a wildtype (WT) lymph gland, under normal conditions the PSC, the first niche identified, regulates the maintenance of the progenitor pool except for core progenitors (blue arrow). Here, we show that by directly acting on core progenitors (orange arrow) the cardiac tube corresponds to a second niche present in the lymph gland. Bnl produced by cardiac cells activates its receptor Btl in progenitors. Btl-FGF activation regulates intracellular Ca2+ levels via PLCγ, and controls the maintenance of core progenitors and in turn the whole progenitor pool. (b) When bnl or btl are knocked down in cardiac cells and progenitors, respectively, an increase in blood cell differentiation in the CZ is observed at the expense of the progenitor pool.
Btl-FGF signaling regulates trachea morphogenesis, which builds the insect respiratory system (Glazer and Shilo, 1991; Klämbt et al., 1992; Muha and Müller, 2013; Sato and Kornberg, 2002; Sutherland et al., 1996). How the ligand Bnl diffuses from its source to activate Btl in neighboring cells remains a controversial issue. Studies performed on Drosophila larval Air-Sac-Primordium (ASP), using endogenous tagged versions of Bnl and Btl, brought to light a key role of long range direct cellular contacts mediated by long thin cellular extensions called cytonemes (Roy et al., 2011; Sato and Kornberg, 2002). In this process, rather than diffusing passively, Bnl produced by wing disc cells is delivered directly to ASP cells by cytonemes to activate FGF signaling (Du et al., 2018). No cytoplasmic extensions from either cardiac cells or MZ progenitors were observed so far, ruling out the delivery of Bnl from cardiac cells though long cytoplasmic extensions. Instead, both cardiac cells and MZ progenitors are embedded in a dense network of extra-cellular matrix (ECM) (Grigorian et al., 2013; Krzemień et al., 2007; Volk et al., 2014). The role of ECM components and associated cell-surface proteins, such as heparan sulfate proteoglycans (HSPGs) (Muha and Müller, 2013), in lymph gland Btl-FGF activation deserves additional investigation.
Bnl::GFP secreted by cardiac cells is detected in MZ progenitors as cytoplasmic punctate dots positive for Rab11, a marker for recycling vesicles, for Rab7, a marker for late endosomes, and for the receptor Btl. These data suggest that Bnl secreted by cardiac cells is internalized by MZ progenitors most likely through receptor-mediated endocytosis. bnl is transcribed in MZ progenitors and Bnl produced by these cells also contributes to lymph gland homeostasis. Taking into account the FGF dose-dependent response shown to operate in vertebrates (Ameri et al., 2010; Iyengar et al., 2007), several lymph gland sources of Bnl could be necessary to reach the threshold needed to fully activate the Btl-FGF pathway in lymph gland progenitors.
Interestingly, the Htl-FGF pathway is also required in MZ progenitors with a loss-of-function phenotype (Dragojlovic-Munther and Martinez-Agosto, 2013) opposite to that of to Btl-FGF inactivation. While Htl-FGF signaling acts through Ras and MAPK activation, we show here that Btl-FGF signaling controls intracellular calcium concentration in hematopoietic progenitors probably through PLCγ activation. By performing epistasis experiments, we further establish the absence of hierarchy between Htl-FGF and Btl-FGF signaling pathways in the MZ and that both pathways are required simultaneously to control lymph gland hematopoiesis. To our knowledge, this is the first example in which Btl and Htl are both expressed and required in the same cell population. We postulate that simultaneous regulation by the two pathways and a Bnl contribution by two separate tissues confers robustness to lymph gland hematopoiesis under normal developmental conditions and flexibility in response to environmental fluctuation. Since Htl and Btl inactivation leads to opposite lymph gland phenotypes, this raises the question of their respective downstream targets.
In vertebrates, FGF signaling controls both primitive and definitive hematopoiesis (Dzierzak and Bigas, 2018; Muha and Müller, 2013; Ornitz and Itoh, 2015). Additional studies indicate that FGFR1 in adult HSPCs is activated during hematopoietic recovery following injury, in order to stimulate HSPC proliferation and mobilization (Zhao et al., 2012). Furthermore, FGF2 facilitates HSPC expansion by amplifying mesenchymal stem cells, a niche cell type (Itkin et al., 2013; Itkin et al., 2012). Thus, in vertebrate adult bone marrow, the FGF pathway plays a major role in the control of hematopoiesis both under steady state conditions and in response to an immune stress. However, deciphering how FGF controls hematopoiesis in bone marrow remains an arduous task since many FGF ligands and receptors are expressed in HSPC and/or niche cells and redundancy and compensation mechanisms between different FGF members can occur (Haas et al., 2018). Given the high conservation of signaling pathways between Drosophila and mammals, the low genetic redundancy in Drosophila and the striking similarities between mammalian bone marrow and fly lymph gland, there is promise that our newly identified regulation of FGF signaling in the lymph gland will shed light on the complex regulation of FGF signaling in mammalian bone marrow.
Materials and methods
Fly strains
Request a detailed protocolw1118 (wild type, WT), UAS-mCD8-GFP and PG125dome-gal4 (Krzemień et al., 2007), antp-gal4 (Mandal et al., 2007), handΔ-gal4 (Morin-Poulard et al., 2016) and NP1029-gal4 (Monier et al., 2005). handΔ-gal4 corresponds to the 3rd intron of hand deleted from the specific visceral mesoderm enhancer (Popichenko et al., 2007 and Laurent Perrin personal communication). Lymph gland mcd8-GFP expression patterns under handΔ-gal4 and NP1029-gal4 drivers in L1, L2, and L3 larvae are given in Figure 1—figure supplement 1. The handΔ-gal4 is expressed in all cardiac cells, whereas the NP1029-gal4 is expressed in all cardiac cells except those that are expressing seven-up (Monier et al., 2005). Strains used are BcGFP (Tokusumi et al., 2009), bnlP2 (Sutherland et al., 1996), hhF4-GFP (Tokusumi et al., 2010), domeMESO-LacZ (Krzemień et al., 2007), domeMESO-Gal4 (Louradour et al., 2017), UAS-Bnl::GFP (Lin, 2009), UAS-btlCA on II or III (Parés and Ricardo, 2016), UAS-Bnl (Jarecki et al., 1999). Ubiquitin-rab11cherryFP (Y. Bellaiche), bnl:GFPendo and btl:cherryendo knock-in alleles (Du et al., 2018). Other strains were provided by the Bloomington (BL) and the Vienna (VDRC) Drosophila RNAi stock centers: btldev1 (BL4912), Sar1-RNAi (BL 32364, Cook et al., 2017), UAS-CaMKII (BL 29662), UAS-GCaMP3 (BL32116), UAS-IP3R (BL30741), sl-RNAi (BL32385 and BL35604), sl2 (BL724). The list of RNAi lines used for the functional screen is given in Figure 1—figure supplement 1. For RNAi treatments, UAS-Dicer two was introduced and at least two RNAi lines per gene were tested. Controls correspond to Gal4 drivers crossed with w1118. In all experiments, crosses and subsequent raising of larvae until late L1/early L2 stage were performed at 22°C, before shifting larvae to 29°C until their dissection at the L3 stage. For gal80ts experiments, crosses were initially maintained at 18°C (permissive temperature) for 3 days after egg laying, and then shifted to 29°C until dissection.
RNAi screen
Request a detailed protocolAntenapedia (Antp) immunostaining was revealed with the ABC kit from Abcam. The images were collected with a Nikon epifluorescence microscope. PSC cell numbers were counted manually using Fiji multi-point tool software. The BcGFP and anterior lobe areas were measured. Crystal cell index corresponds to BcGFP area/anterior lobe area. 2 RNAi lines per gene were tested when available, and at least 15 lymph glands were analyzed per genotype.
Generation of DomeMESO-RFP transgenic lines
Request a detailed protocolThe domeMESO sequence from pCasHs43domeMESO-lacZ (Rivas et al., 2008) was sub-cloned into pENTR Directional, following the experimental procedure of the TOPOCloning Kit from Invitrogen. The resulting plasmid was used to generate domeMESO-RFP transgenic flies using attP/attB technology (Bischof et al., 2007). The Drosophila line was created by integration at attP-68A4 (III) sites.
Antibodies and immunostaining
Request a detailed protocolLymph glands were dissected and processed as previously described (Krzemień et al., 2007). Antibodies used were mouse anti-Col (1/100) (Krzemień et al., 2007), chicken anti-βgal (1/1000, Abcam), rabbit anti-RFP (1/40 000, Rockland Immunochemicals), chicken anti-GFP(1/500, Abcam), mouse anti-Antp (1/100, Hybridoma Bank), mouse anti-Hnt (1/100, Hybridoma Bank); mouse anti-P1 (1/30, I. Ando, Institute of Genetics, Biological Research Center of the Hungarian Academy of Science, Szeged, Hungary), mouse anti-proPO (1/100, T.Trenczel, Justus-Liebig-University Giessen, Giessen, Germany). Secondary antibodies were Alexa Fluor-488 and −555 conjugated antibodies (1:1000, Molecular Probes) and goat anti-Chicken Alexa Fluor-488 (1/800; Molecular Probes). Nuclei were labeled with TOPRO3 (Thermo Fisher Scientific). Immunostainings were performed as previously described (Louradour et al., 2017). For detecting bnl:GFPendo and btl:cherryendo immunostainings were performed with anti-GFP and anti-RFP, respectively.
In situ hybridization
Request a detailed protocolThe protocol was as described in Oyallon et al., 2016. For fluorescent in situ hybridization we used digoxigenin-labeled tep4 and bnl probes. For revelation, samples were incubated with sheep-anti-DIG (1/1000, Roche) followed by biotinylated donkey-anti-sheep (1/500, Roche). ABC kit from Vector Laboratory was used followed by fluorescent tyramide staining (Alexa fluor 555 or 488 conjugated tyramide from Molecular Probes). The bnl probe was transcribed in vitro using T7 RNA polymerase II, from PCR-amplified DNA sequences. Pairs of primers were used and the sequence in italics corresponds to the T7 RNA-Pol II fixation site. For bnl: primer 1: GCCATGGACAACAACTTGAC/ATGAATTCTAATACGACTCACT ATAGGGCGTCGTTACGGTCCAGATTG; primer 2: GCAAGGCCAACAAGAAGAAG/ATGAATTCTAATACGACTCACTATAGGGCCTGGTCGTTATCCTGATCC.
Quantification of PSC cell numbers
Request a detailed protocolIn all experiments, genotypes were analyzed in parallel and quantified. PSC cells were counted manually using Fiji multi-point tool software. Statistical analyses (Mann–Whitney nonparametric test) were performed using GraphPad Prism five software.
Blood cell and progenitor quantification
Request a detailed protocolCrystal cells were visualized by either BcGFP or immunostainings with antibodies against proPO or Hnt. Plasmatocytes were labeled by P1 immunostainings. DomeMESO-RFP and DomeMESO-GFP were expressed in MZ progenitors, whereas MZ core progenitors were labeled by either tep4 in situ hybridization or Col immunostainings. Optimized confocal sections were performed on Leica SPE or SP8 microscopes for 3D reconstruction. The numbers of crystal cells, plasmatocytes and progenitors stained and anterior lobe volume (in µm3) were measured using Volocity 3D Image Analysis software (PerkinElmer). Crystal cell index: (crystal cell number/anterior lobe volume)x100000; plasmatocyte and progenitor index: (plasmatocyte or progenitor volume/anterior lobe volume)x100. At least 15 anterior lobes were scored per genotype, and experiments were reproduced at least three times. Statistical analyses (Mann–Whitney nonparametric test) were performed using GraphPad Prism five software. Since the number of lymph gland differentiated blood cells fluctuates depending on the larval stage, and to limit discrepancies in all the experiments, genotypes were analyzed in parallel.
Quantification of hhF4-GFP and UAS-GCaMP3 intensity
Request a detailed protocolOptimized confocal sections were performed on Leica SPE or SP8 microscopes for 3D reconstruction. For hhF4-GFP, the sum intensities for GFP per PSC labeled by Col and each PSC volume (in μm3) were measured using Volocity 3D Image Analysis software (PerkinElmer). The intensity of hhF4-GFP corresponds to the sum intensity of hhF4-GFP/the PSC volume. For GCaMP3, the sum intensities for GFP per lymph gland primary lobe labeled by TOPRO and each primary lobe volume (in μm3) were measured using Volocity 3D Image Analysis software. The intensity of GCaMP3 corresponds to the sum intensity of GCaMP3/per lymph gland primary lobe volume. At least 15 anterior lobes were scored per genotype, and experiments were reproduced at least three times. Statistical analyses (Mann–Whitney nonparametric test) were performed using GraphPad Prism five software.
Quantification of the diffusion in the MZ of cytoplasmic Bnl::GFP dots, in hand >Bnl::GFP and hand >Bnl::GFP >sar1 RNAi genetic contexts
Request a detailed protocolOptimized lymph gland confocal sections were obtained with a Leica SP8 microscope for 3D reconstruction. The maximum projection of 10 slices chosen in the middle of the stack was performed. A parallelepiped with a larger corresponding to four nuclei diameter, a width of 10 confocal slices a length corresponding to the distance from the CT to the CZ was designed. Along the length, the parallelepiped was subdivided into 11 sub parallelepipeds of similar size. The number of Bnl::GFP granules per sub parallelepiped (called interval in Figure 4g legend) was counted. Spot detector plugin from ICY software (http://icy.bioimageanalysis.org/) was used to quantify the number of Bnl::GFP dots per sub parallelepiped.
Sample size
Request a detailed protocoln corresponds to the number of anterior lobes analyzed. Figure 1: In e, for handΔ> n = 61 and n = 26 for handΔ>ilp6 RNAi. In h, for handΔ> n = 61 and n = 24 for handD >ds RNAi. In k, handΔ> n = 61 and n = 24 for handΔ>pvf3 RNAi. In f, for handΔ> n = 19 and n = 27 for handΔ>ilp6 RNAi. In i, for handΔ> n = 26 and n = 21 for handΔ>ds RNAi. In l, handΔ> n = 26 and n = 26 for handΔ>pvf3 RNAi. In o, for handΔ> n = 14 and n = 10 for handΔ>pvf3 RNAi. Figure 2: in d, n = 12 for WT and n = 10 for bnlP2/+. In i, handΔ> n = 22, handΔ>bnl-RNAi n = 13, HandΔ>bnl n = 38 and handΔ>bnl;bnl-RNAi n = 10. In l, for handΔ> n = 24 and n = 15 for handΔ>bnl-RNAi. In o, for handΔ>n = 25 and n = 28 in handΔ>bnl-RNAi. For r, handΔ> n = 36 and n = 22 for handΔ>bnl-RNAi. In u, for handΔ>n = 16 and n = 18 in handΔ>bnl-RNAi.
Figure 3a-a" domeMESO-GFP crossed with btl:mcherryendo; 3b and k: PG125dome-gal4,UAS-dcr2 crossed with w1118; c btldev1/TM6B crossed with w1118; e and i: PG125dome-gal4,UAS-dcr2 crossed with UAS-btl-RNAi; f: PG125dome-gal4,UAS-dcr2 crossed with UAS-btlCA; UAS-btl-RNAi; h: PG125dome-gal4,UAS-dcr2; DomeMESO-LacZ crossed with w1118; i: PG125dome-gal4,UAS-dcr2; DomeMESO-LacZ crossed with UAS-btl-RNAi; n: PG125dome-gal4,UAS-dcr2 crossed with UAS-htlDN; o: PG125dome-gal4,UAS-dcr2 crossed with UAS-htlDN; UAS-btl-RNAi.
In d, n = 45 for WT and n = 30 for btl dev1/+. In g, dome >n = 93, dome >btl-RNAi n = 23, dome >btlCA n = 12 and dome >btlCA;btl-RNAi n = 20. In j, n = 27 for dome> and n = 21 for dome >btl-RNAi. In m, n = 20 for dome> and n = 24 for dome >btl-RNAi. In p, n = 16 for dome>, n = 13 for dome >htlDN. n = 18 for dome >btl-RNAi and n = 29 for dome >dome > htlDN>btl-RNAi.
Figure 4a handΔ,UAS-dcr2 crossed with UAS-bnl::GFP; 4b: handΔ,UAS-dcr2; btl:mcherryendo crossed with UAS-Bnl::GFP; 4 c: handΔ,UAS-dcr2; UAS-bnl::GFP crossed with ubi-rab11::mcherry; 4d: handΔ,UAS-dcr2 crossed with UAS-bnl::GFP; 4e: handΔ,UAS-dcr2; UAS-Bnl::GFP crossed with w1118; 4 f: handΔ,UAS-dcr2; Bnl::GFP crossed with UAS-sar1-RNAi; 4 hr: handΔ,UAS-dcr2; BcGFP crossed with w1118; 4i: handΔ,UAS-dcr2; BcGFP crossed with:UAS-sar1-RNAi; 4 j: handΔ,UAS-dcr2; BcGFP crossed with UAS-sar1-RNAi; UAS-bnl::GFP.
In k: handΔ> n = 27, handΔ>sar1 RNAi n = 14, and handΔ>sar1 RNAi; Bnl//GFP n = 17.
Figure 5a PG125dome-gal4,UAS-dcr2 crossed with UAS-GCaMP3 ; 5b : PG125dome-gal4,UAS-dcr2 crossed with UAS-GCaMP3 ; UAS-btl-RNAi ; 5d : PG125dome-gal4,UAS-dcr2 crossed with w1118; 5e :PG125dome-gal4,UAS-dcr2 crossed with UAS-btl-RNAi ; 5 f :PG125dome-gal4,UAS-dcr2 crossed with UAS-CaMKII ; 5 g :PG125dome-gal4,UAS-dcr2 crossed with UAS-CaMKII ; UAS-btl-RNAi ; 5i :PG125dome-gal4,UAS-dcr2 crossed with UAS-sl-RNAi ; 5 j : sl2 ; 5 k : sl2; dome-gal4 crossed with sl2; UAS-btl-CA .
In c, n = 41 for dome> and n = 29 for dome >btl-RNAi. In h, for dome> n = 35, n = 33 for dome >btl-RNAi, n = 35 for dome >CaMKII and n = 26 for dome >CaMKII; btl-RNAi. In l, dome> n = 27 and n = 30 in dome >sl-RNAi. In m, WT n = 20 and n = 17 in sl2. In n, sl2, dome >n = 20 and n = 21 in sl2; dome >btlCA.
Replicates
Request a detailed protocolFigure 2a-a" domeMESO-GFP crossed with w1118; Figure 2b: w1118; Figure 2c: bnlP2/TM6B crossed with w1118; Figure 2e: handΔ,UAS-dcr2; BcGFP crossed with w1118; 2 f: handΔ,UAS-dcr2; BcGFP crossed with UAS-bnl-RNAi; 2 g: handΔ,UAS-dcr2; BcGFP crossed with UAS-bnl; 2 hr: handΔ,UAS-dcr2; BcGFP crossed with UAS-bnl;UAS-bnl-RNAi; 2 j: handΔ,UAS-dcr2; domeMESO-RFP crossed with w1118; 2 k: handΔ,UAS-dcr2; domeMESO-RFP crossed with UAS-bnl-RNAi; 2 m and p: handΔ,UAS-dcr2 crossed with w1118; 2 n and q: handΔ,UAS-dcr2 crossed with UAS-bnl-RNAi. s: handΔ,UAS-dcr2; bnl:GFPendo crossed with w1118; t: handΔ,UAS-dcr2; bnl:GFPendo crossed with UAS-bnl-RNAi.
(d, i) three independent experiments were performed and quantified. One is shown. (l) two independent experiments were performed and quantified. One is shown. (o) three independent experiments were performed and quantified. One is shown. (r) two independent experiments were performed and quantified. One is shown. (u) two independent experiments were performed and quantified. One is shown. Figure 3: (d, g) three independent experiments were performed and quantified. One is shown. (j) two independent experiments were performed and quantified. One is shown. (m) three independent experiments were performed and quantified. One is shown. (p) two independent experiments were performed and quantified. One is shown Figure 4: (k) two independent experiments were performed and quantified. One is shown. (Figure 5c,h,l–n) two independent experiments were performed and quantified. One is shown.
Drosophila genetics
Request a detailed protocolFly crosses for each figure:
Figure 1c-c', m handΔ,UAS-dcr2; BcGFP crossed with w1118; Figure 1d,d' : handΔ,UAS-dcr2; BcGFP crossed with UAS-ilp6-RNAi; Figure 1g,g' : handΔ,UAS-dcr2; BcGFP crossed with UAS-ds-RNAi; Figure 1j,j', n : handΔ,UAS-dcr2; BcGFP crossed with UAS-pvf3-RNAi.
Figure 1—figure supplement 1a-c handΔ,UAS-dcr2 crossed with UAS-mcd8GFP; d-f:NP1029, UAS-dcr2 crossed with UAS-mcd8GFP.
Figure 2—figure supplement 2a PG125dome-gal4,UAS-dcr2 crossed with w1118; b: PG125dome-gal4,UAS-dcr2 crossed with UAS-bnl-RNAi; d: PG125dome-gal4,UAS-dcr2 crossed with w1118; e: PG125dome-gal4,UAS-dcr2 crossed with UAS-bnl-RNAi; g: handΔ,UAS-dcr2 crossed with w1118; h: handΔ,UAS-dcr2 crossed with UAS-bnl; UAS-bnl-RNAi; j: handΔ,UAS-dcr2 crossed with w1118; k: handΔ,UAS-dcr2 crossed with UAS-bnl-RNAi; m: hhF4-GFP; handΔ,UAS-dcr2 crossed with w1118; n: hhF4-GFP; handΔ,UAS-dcr2 crossed with UAS-bnl-RNAi.
Figure 3—figure supplement 1 a : BcGFP crossed with btl:cherryendo; b : hml-gal4, UAS-mcd8-GFP crossed with btl:cherryendo; c : pcol-gal4, UAS-mcd8-GFP crossed with btl:cherryendo; d : handΔ,UAS-dcr2; BcGFP crossed with w1118; e: handΔ,UAS-dcr2; BcGFP crossed with UAS-btl-RNAi ; g: handΔ,UAS-dcr2 crossed with w1118; h: handΔ,UAS-dcr2 crossed with UAS-btl-RNAi; j and m: PG125dome-gal4,UAS-dcr2 crossed with w1118; k and n: PG125dome-gal4,UAS-dcr2 crossed with UAS-btl-RNAi.
Figure 4—figure supplement 1a handΔ, UAS-dcr2 crossed with w1118; b: handΔ, UAS-dcr2 crossed with UAS-sar1-RNAi; d and g: NP1029, UAS-dcr2 crossed with w1118; e and h: handΔ, UAS-dcr2 crossed with UAS-sar1-RNAi.
Figure 5—figure supplement 1 : a : PG125dome-gal4,UAS-dcr2 crossed with UAS-GCaMP3 ; b: PG125dome-gal4,UAS-dcr2 crossed with UAS-GCaMP3 ;UAS-btl-RNAi ; d : tep4-gal4,UAS-dcr2 crossed with w1118 ; e : tep4-gal4,UAS-dcr2 crossed with UAS-btl-RNAi ; f : tep4-gal4,UAS-dcr2 crossed with UAS-IP3R; g : tep4-gal4,UAS-dcr2 crossed with UAS-IP3R ; UAS-btl-RNAi.
Sample size
Request a detailed protocolFigure 1—figure supplement 1 At least 10 anterior lobes for each condition were analyzed. Figure 2—figure supplement 1: (h) For handΔ n = 16 and n = 17 for handΔ>bnl-RNAi. (k) for handΔ>n = 24 and n = 23 for handΔ>bnl-RNAi. (n) for NP1029 >n = 46 and n = 29 for NP1029 >bnl-RNAi. (q) for handΔ>; tub80ts n = 20 and n = 14 for handΔ>bnl-RNAi; tub80ts. Figure 2—figure supplement 2: (c) for dome >n = 24 and n = 26 for dome >bnl-RNAi. (f) dome >n = 23 and n = 24 in dome >bnl-RNAi. (i) for handΔ>n = 13, handΔ>bnl-RNAi n = 11, handΔ>bnl n = 11 and handΔ>bnl; bnl-RNAi n = 10. (l) for handΔ>n = 49 and n = 22 for handΔ>bnl RNAi. (o) for handΔ>n = 40 and n = 22 for handΔ>bnl-RNAi. Figure 3—figure supplement 1: (f) for handΔ>n = 23 and n = 17 for handΔ>btl-RNAi. (i) for handΔ>n = 50 and n = 34 for handΔ>btl-RNAi. (l) For dome >n = 26 and n = 22 for dome >btl-RNAi. (o) for dome >n = 21 and n = 23 for dome >btl-RNAi. Figure 4—figure supplement 1: (c) for handΔ>n = 37 and n = 28 for handΔ>sar1 RNAi. (f) for NP1029 >n = 20 and n = 28 for NP1029 >sar1 RNAi. (i) for NP1029 >n = 15 and n = 28 for NP1029 >sar1 RNAi. Figure 5—figure supplement 1: (c) for dome >n = 14 and for dome >btl-RNAi n = 7. (h) for tep4 >n = 14, for tep4 >btl-RNAi n = 12, for tep4 >IP3R n = 27 and for tep4 >IP3R; btl-RNAi n = 27.
Replicates
Request a detailed protocolFigure 2—figure supplement 1a BcGFP crossed with w1118; b: hml-gal4, UAS-mcd8GFP crossed with w1118; c: pcol-gal4, UAS-mcd8-GFP crossed with w1118; d: handΔ,UAS-dcr2 crossed with UAS-mcd8-GFP; e: handΔ,UAS-dcr2 crossed UAS-mcd8-GFP; UAS-bnl-RNAi; f: handΔ,UAS-dcr2 crossed with w1118; g: handΔ,UAS-dcr2 crossed with UAS-bnl-RNAi; i: handΔ,UAS-dcr2 crossed with w1118; j: handΔ,UAS-dcr2 crossed with UAS-bnl-RNAi 34572; l: NP1029, UAS-dcr2 crossed with w1118; m: NP1029, UAS-dcr2 crossed with UAS-bnl-RNAi; o: handΔ,UAS-dcr2;tub- gal80ts crossed with w1118; p: handΔ,UAS-dcr2;tub- gal80ts crossed with UAS-bnl-RNAi.
(h)two independent experiments were performed and quantified. One is shown. (k and n) three independent experiments were performed and quantified. One is shown. (q) two independent experiments were performed and quantified. Figure 2—figure supplement 2: (c) three independent experiments were performed and quantified. One is shown. (f and i) two independent experiments were performed and quantified. One is shown. (l and o) three independent experiments were performed and quantified. One is shown. Figure 3—figure supplement 1: (f) three independent experiments were performed and quantified. One is shown. (i). two independent experiments were performed and quantified. One is shown. (l and o) three independent experiments were performed and quantified. One is shown. Figure 4—figure supplement 1: (c, f and i) two independent experiments were performed and quantified. One is shown. Figure 5—figure supplement 1: (c and h) two independent experiments were performed and quantified. One is shown.
Data availability
All data generated or analysed during this study are included in the manuscript and supporting files. Source data files have been provided for all figures.
References
-
Complexity of bone marrow hematopoietic stem cell nicheInternational Journal of Hematology 106:45–54.https://doi.org/10.1007/s12185-017-2262-9
-
Blood cells and blood cell development in the animal kingdomAnnual Review of Cell and Developmental Biology 22:677–712.https://doi.org/10.1146/annurev.cellbio.22.010605.093317
-
Bone marrow vascular niche: home for hematopoietic stem cellsBone Marrow Research 2014:1–8.https://doi.org/10.1155/2014/128436
-
Fibroblast growth factor signaling promotes physiological bone remodeling and stem cell self-renewalCurrent Opinion in Hematology 20:1–244.https://doi.org/10.1097/MOH.0b013e3283606162
-
Postembryonic hematopoiesis in DrosophilaDevelopmental Biology 230:243–257.https://doi.org/10.1006/dbio.2000.0123
-
The host defense of Drosophila melanogasterAnnual Review of Immunology 25:697–743.https://doi.org/10.1146/annurev.immunol.25.022106.141615
-
ThesisClonal Analysis of Growth Behaviors During Drosophila Larval Tracheal Development In Doctoral thesisUniversity of Basel.
-
Spatiotemporal gene expression targeting with the TARGET and gene-switch systems in DrosophilaScience's STKE : Signal Transduction Knowledge Environment 2004:pl6.https://doi.org/10.1126/stke.2202004pl6
-
Functions and mechanisms of fibroblast growth factor (FGF) Signalling in Drosophila melanogasterInternational Journal of Molecular Sciences 14:5920–5937.https://doi.org/10.3390/ijms14035920
-
A high signal-to-noise ca(2+) probe composed of a single green fluorescent proteinNature Biotechnology 19:137–141.https://doi.org/10.1038/84397
-
The fibroblast growth factor signaling pathwayWiley Interdisciplinary Reviews: Developmental Biology 4:215–266.https://doi.org/10.1002/wdev.176
-
FGF control of E-cadherin targeting in the Drosophila midgut impacts on primordial germ cell motilityJournal of Cell Science 129:354–366.https://doi.org/10.1242/jcs.174284
-
Regulation of the Sar1 GTPase cycle is necessary for large cargo secretion from the endoplasmic reticulumFrontiers in Cell and Developmental Biology 5:75.https://doi.org/10.3389/fcell.2017.00075
-
Small wing encodes a phospholipase C-(gamma) that acts as a negative regulator of R7 development in DrosophilaDevelopment 125:5033–5042.
-
Fibroblast growth factor signalling: from development to CancerNature Reviews Cancer 10:116–129.https://doi.org/10.1038/nrc2780
-
Molecular mechanisms of sar/Arf GTPases in vesicular trafficking in yeast and plantsFrontiers in Plant Science 5:411.https://doi.org/10.3389/fpls.2014.00411
-
The Drosophila lymph gland is an ideal model for studying hematopoiesisDevelopmental & Comparative Immunology 83:60–69.https://doi.org/10.1016/j.dci.2017.11.017
-
Regulation of stress-induced hematopoiesisCurrent Opinion in Hematology 22:286–292.https://doi.org/10.1097/MOH.0000000000000149
Article and author information
Author details
Funding
Ministère de l'Education Nationale, de l'Enseignement Superieur et de la Recherche ('ANR programme blanc')
- Michele Crozatier
Fondation ARC pour la Recherche sur le Cancer (ARC)
- Manon Destalminil-Letourneau
Fondation pour la Recherche Médicale (FRM)
- Michele Crozatier
Ligue Contre le Cancer
- Michele Crozatier
China Scholarship Council
- Yushun Tian
Société francaise d'hématologie
- Manon Destalminil-Letourneau
The funders had no role in study design, data collection and interpretation, or the decision to submit the work for publication.
Acknowledgements
We thank M Affolter, Y Bellaiche, J Casanova, J Colombani, L Du, M Freeman, M Grammont, M A Krasnow, A Paululat, L Perrin, S Ricardo, S Roy, T Tanaka, P Thérond, Bloomington and Vienna Stock Center and the TRiP at Harvard Medical School for fly strains; I Ando, A Moore and T Trenczek for antibodies; L Bataillé, A Davy, G Lebreton, M Meister, C Monod, B Monnier and A Vincent, for critical reading of the manuscript. We are grateful to B Ronsin and S Bosch for assistance with confocal microscopy (Plateforme TRI); J Favier, V Nicolas and A Destenable for fly culture. Research in the authors’ laboratory is supported by the CNRS, University Toulouse III, Ministère de la Recherche (ANR « programme blanc »), ARC (Association pour la Recherche sur le Cancer), La Ligue contre le Cancer 31, La Société Française d’Hématologie (SFH), FRM (Fondation pour la Recherche Médicale) and the China Scholarship Council.
Copyright
© 2021, Destalminil-Letourneau et al.
This article is distributed under the terms of the Creative Commons Attribution License, which permits unrestricted use and redistribution provided that the original author and source are credited.
Metrics
-
- 1,662
- views
-
- 234
- downloads
-
- 33
- citations
Views, downloads and citations are aggregated across all versions of this paper published by eLife.
Citations by DOI
-
- 33
- citations for umbrella DOI https://doi.org/10.7554/eLife.64672