A general approach for stabilizing nanobodies for intracellular expression
Figures
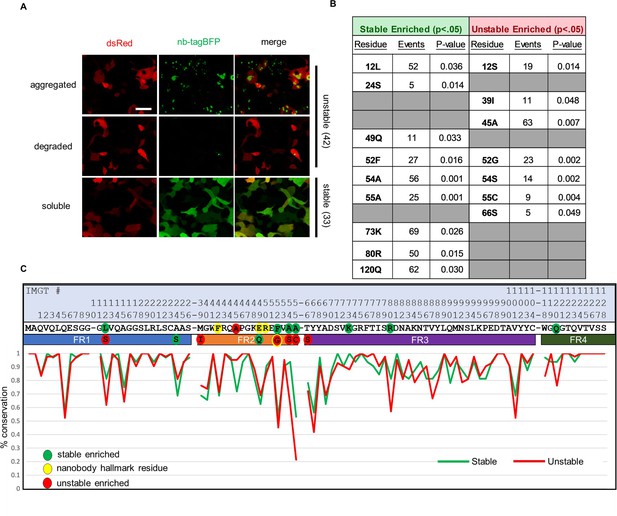
Sequence differences between intracellularly stable and unstable nanobodies.
(A) Representative images of intracellularly stable and unstable nanobody-TagBFP fusions in transiently transfected 293T cells. Red signal is from co-transfected CAG-dsRed plasmid. (B) Significantly enriched positional residues between stable and unstable nanobodies (Fisher’s exact test). Events denote the total number of instances of each positional residue across all nanobodies (75 total). Gray cells denote positional insignificance. (C) Positional amino acid conservation across framework sequences of stable and unstable nanobodies. Scale bar in Panel A is 20 µm.
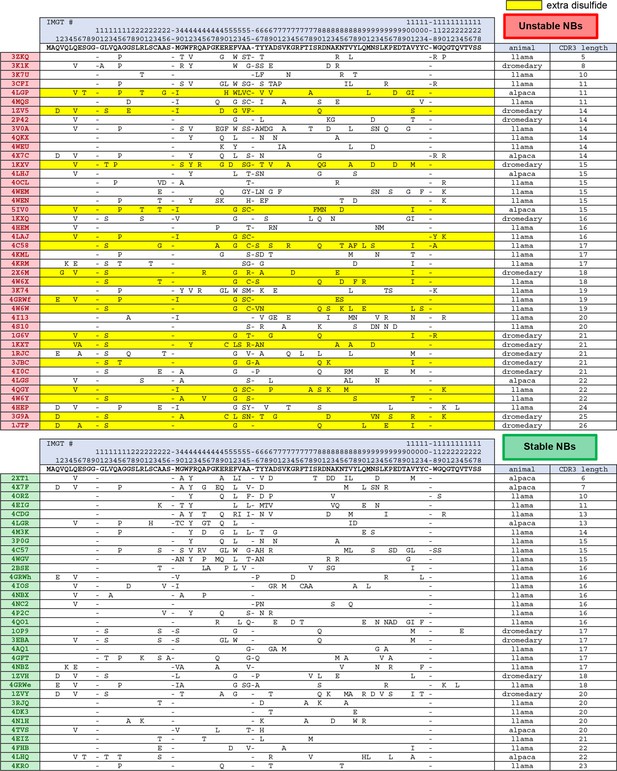
Framework sequence variability (compared to consensus sequence) across stable and unstable nanobodies.
Note: All nanobodies contain a conserved set of cysteines that normally forms a disulfide bond through the hydrophobic core of the nanobody (23Cys and 104Cys). Highlighted in yellow, ‘extra disulfide bond’ refers to nanobodies with an additional pair of cysteines, one of which is always located in CDR3, that normally forms a disulfide bond that impacts CDR3 conformation.
-
Figure 2—source data 1
Editable version of Figure 2.
- https://cdn.elifesciences.org/articles/68253/elife-68253-fig2-data1-v1.docx
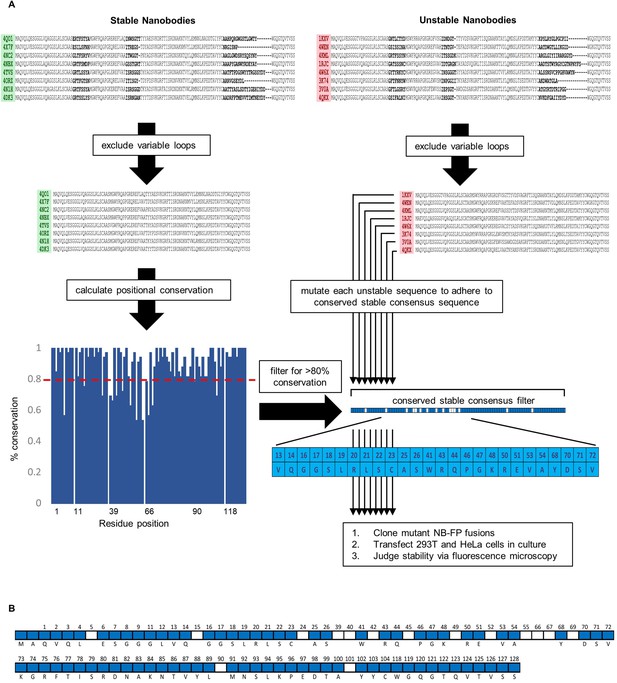
Schematic overview of conservation-based stabilizing mutagenesis strategy.
(A) Nanobody-TagBFP fusions were classified as ‘stable’ or ‘unstable’ based on intracellular expression via transient transfection in 293T cells. Amino acid sequences were binned according to stability group, and variable domain sequences (CDRs) were excluded from downstream consideration. Positional sequence conservation was calculated across stable nanobody sequences, and positional amino acids of high conservation (>80%) were compiled to form a partial consensus filter. Each individual unstable nanobody framework was then compared to this filter, and any positional amino acid disagreement was resolved to adhere to the filter. Mutated nanobodies were then cloned, transfected into 293T and HeLa cells, and judged for stability via fluorescence microscopy. (B) All positional amino acids captured in the partial consensus framework (blue cells). White cells denote framework positions excluded from the partial consensus framework.
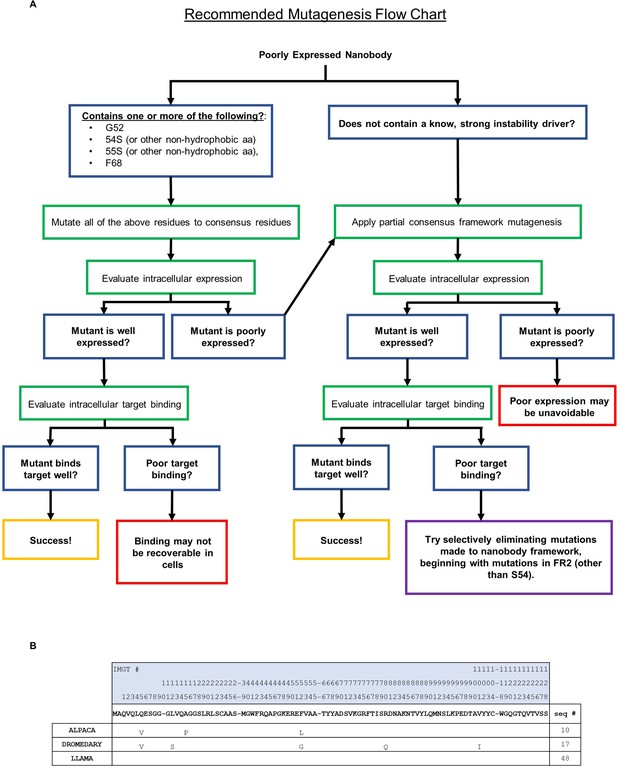
Nanobody mutagenesis flow chart and species consensus comparison.
(A) Flow chart detailing our recommended courses of action for mutationally stabilizing poorly expressed nanobodies.Our partial consensus filter is depicted in Figure 3, for reference. (B) Comparison between the framework consensus sequence of stable nanobodies profiled in this study, and the framework consensus sequences of all nanobodies from each species of origin profiled. The majority of nanobodies profiled originate from llama, and our stable framework consensus sequence is identical to the framework consensus sequence derived from all llama sequences profiled. We did not formally investigate the implication on expression of specific framework differences observed between alpaca, dromedary, and llama sequences.
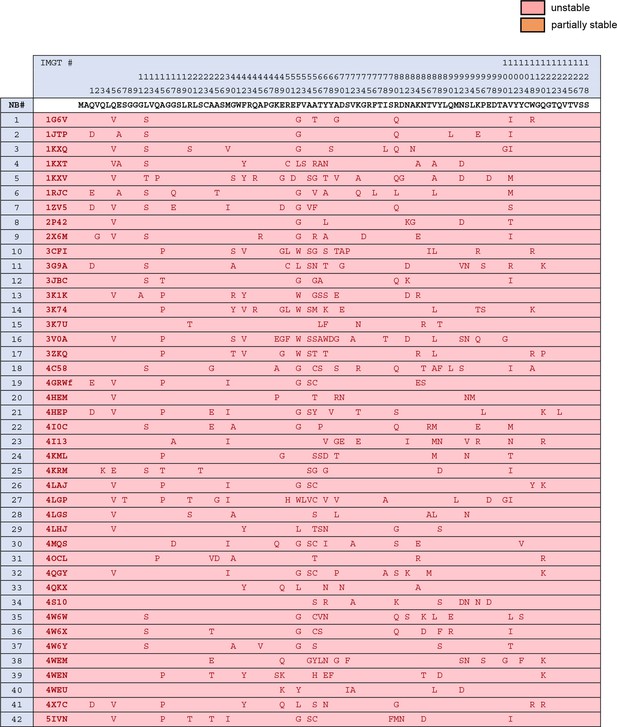
A pre-mutagenesis framework sequence variability across unstable nanobodies.
-
Figure 4—source data 1
Editable version of Figure 4.
- https://cdn.elifesciences.org/articles/68253/elife-68253-fig4-data1-v1.docx
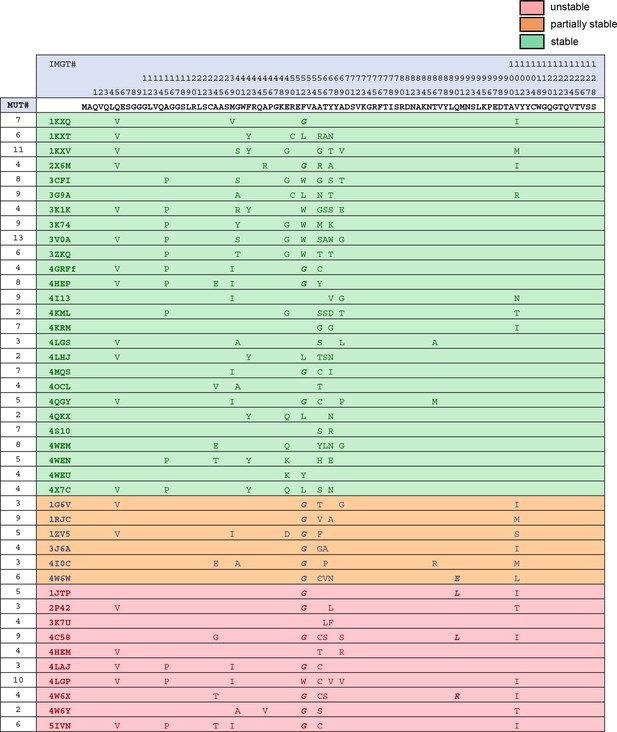
B sequence and stability variability across nanobodies following stage 1 mutagenesis *52Gly and 90X are bolded and italicized.
The number of mutations made to each nanobody is reported in the left-most column.
-
Figure 5—source data 1
Editable version of Figure 5.
- https://cdn.elifesciences.org/articles/68253/elife-68253-fig5-data1-v1.docx
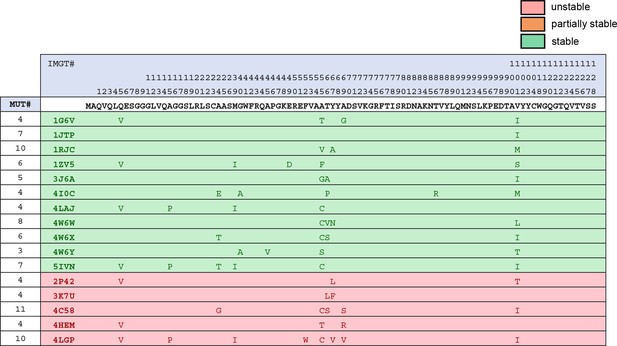
C sequence and stability variability across nanobodies following stage 2 mutagenesis (+G52F+X90Q).
-
Figure 6—source data 1
Editable version of Figure 6.
- https://cdn.elifesciences.org/articles/68253/elife-68253-fig6-data1-v1.docx
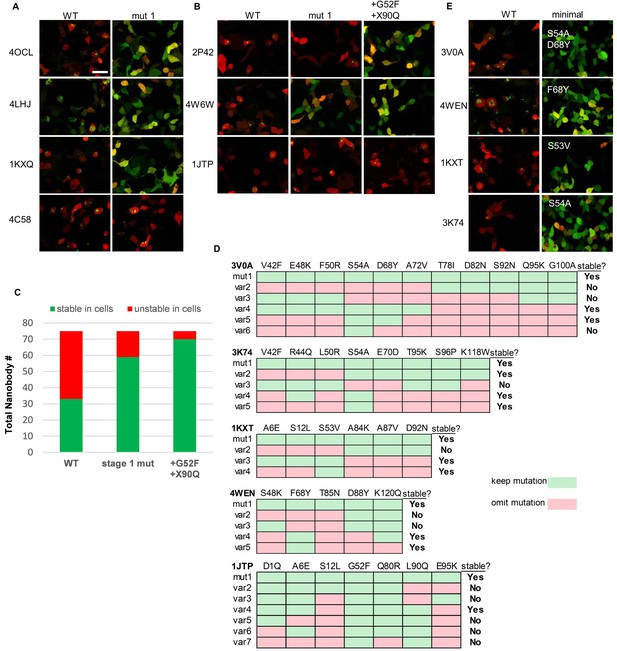
Mutationally stabilized nanobodies and specific stability drivers.
(A) Examples of nanobody-TagBFP expression in 293T cells following transient transfection before (WT) and after (mut1) stage 1, conservation-based mutagenesis. Red signal is from co-transfected CAG-dsRed plasmid. (B) Examples of nanobody-TagBFP expression in 293T cells following transient transfection for nanobodies with Gly52. Wild-type, first-pass conservation-based mutants (mut1), and mutants with up to two additional mutations, Gly52Phe and X90Gln, are depicted. (C) Numbers of stable versus unstable nanobodies (75 total) following first-pass conservation-based mutagenesis (stage 1 mut) and final mutagenesis (+G52F+X90Q). (D) Example mutant variants tested for specific mutationally stabilized nanobodies in order to identify necessary stability mutations. (E) Examples of nanobody-TagBFP expression in 293T cells following transient transfection for nanobodies effectively stabilized by one to two mutations. Scale bar is 20 µm.
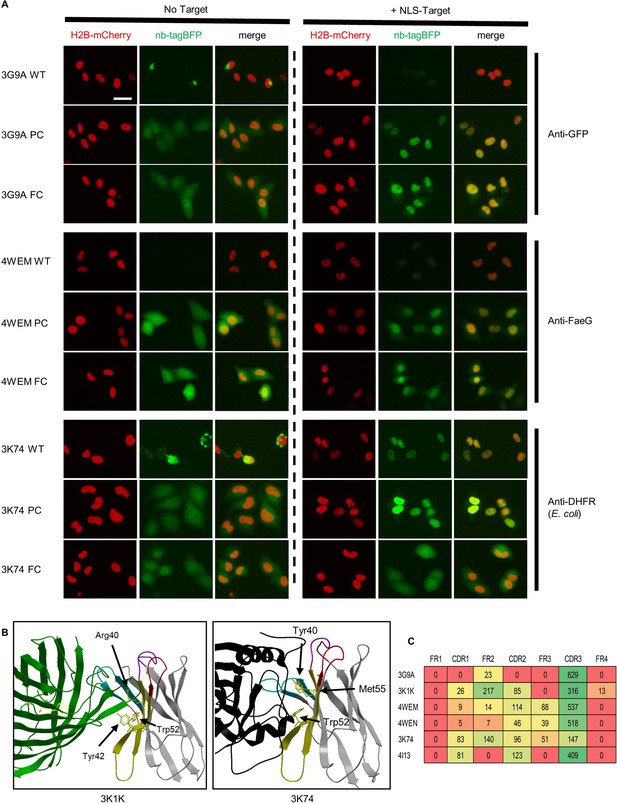
Target binding of parent and mutationally stabilized nanobodies.
(A) Representative images of nanobody-TagBFP expression in HeLa cells in the presence and absence of nuclearly localized target. Wild-type (WT) nanobodies, partial consensus (PC) mutants, and full-consensus (FC) mutants are depicted. Red nuclear signal is from co-transfected CAG-H2B-mCherry plasmid. Transfected DNA and nuclear protein amounts were standardized by addition of off-target nuclear localization sequence (NLS) plasmid to transfection mix for the ‘no target’ condition. Scale bar is 25 µm. (B) Crystal structures of two nanobodies that lose target binding when mutated to adhere to a full-consensus framework are shown. Non-consensus framework residues directly contributing to target interface are depicted. (C) Target-interfacing surface area values in square angstroms (rounded to whole numbers) across distinct regions for nanobodies tested for target binding are shown. Values are taken from buried surface area interface values made available through PDBE-PISA.
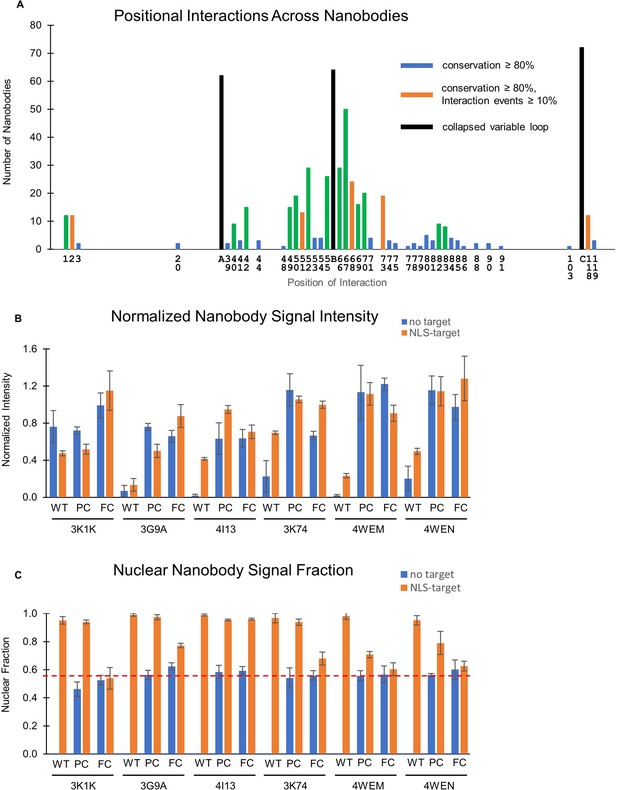
Positional interactions and intensity measurements.
(A) Total number of target-interfacing positions across 75 nanobodies. Positions of interaction were determined based on buried surface area for crystal structure interfaces made available through PDBE-PISA. Black bars labeled ‘A, B, and C’ denote positions across CDRs 1, 2, and 3, respectively. Colored bars describe sequence-level conservation across target-interfacing framework positions. (B) Nanobody-TagBFP signal intensities normalized to co-transfected H2B-mCherry signal intensities from captured fluorescence images (n = 3 replicate experiments for each condition, error bars denote standard deviation). (C) Nanobody-TagBFP signal fraction colocalized with H2B-mCherry signal in co-transfected cells from captured fluorescence images (n = 3 replicate experiments for each condition, error bars denote standard deviation). Red-dashed line represents average nuclear colocalization between nanobody-TagBFP signals and H2B-mCherry signals in the absence of nuclear target. Cells without both nanobody-TagBFP and H2B-mCherry signal were excluded from analysis.
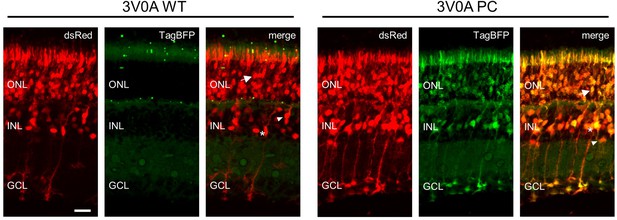
Nanobody expression in the murine retina representative Images of murine retina co-electroporated with CAG-dsRed and either wild-type or mutant CAG-3V0A-TagBFP plasmid.
Retinas were electroporated on postnatal day 2 and harvested on postnatal day 12. Multiple cell types show expression following electroporation: photoreceptors (arrow), bipolar interneurons (triangle), and Mueller glia (asterisk) are noted on merged images. Scale bar is 20 µm.
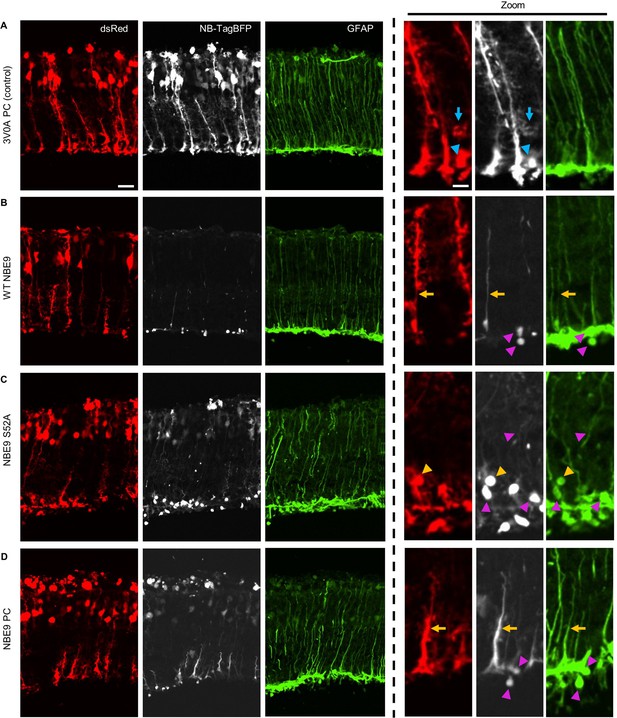
Nanobody targeting endogenous factor in the murine retina.
(A–D) Representative Images of rd1 murine retina co-electroporated with CAG-dsRed and CAG-nanobody-TagBFP plasmids. NBE9 is a nanobody that targets glial fibrillary acidic protein (GFAP), a protein whose expression in Mueller glia is upregulated in the rd1 retinal degeneration model. Retinas were electroporated on postnatal day 2 and harvested on postnatal day 20. Triangles denote features of accumulated protein, and arrows denote radial processes. Blue indicators denote features observed in both the dsRed and nanobody-TagBFP channels, pink indicators denote features observed only in the nanobody-TagBFP and GFAP channels, and orange indicators denote features observable across all three channels. Scale bars for main panels are 20 µm and for higher magnification panels are 4 µm.
Additional files
-
Transparent reporting form
- https://cdn.elifesciences.org/articles/68253/elife-68253-transrepform1-v1.docx
-
Supplementary file 1
Full nanobody sequences and targets.
- https://cdn.elifesciences.org/articles/68253/elife-68253-supp1-v1.docx
-
Supplementary file 2
Nanobody interaction interfaces.
- https://cdn.elifesciences.org/articles/68253/elife-68253-supp2-v1.xlsx