Analysis of the PcrA-RNA polymerase complex reveals a helicase interaction motif and a role for PcrA/UvrD helicase in the suppression of R-loops
Figures
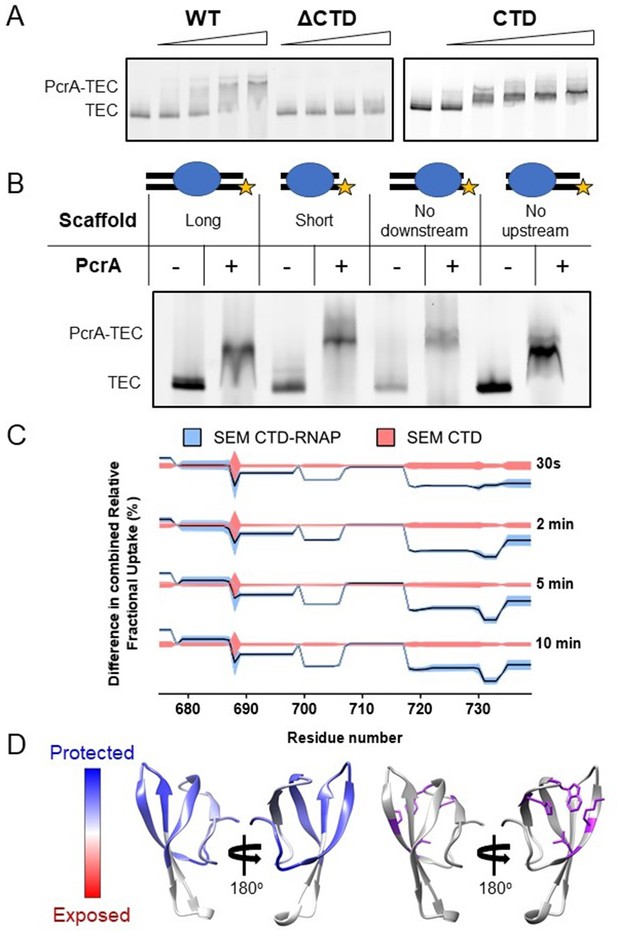
Interactions between PcrA and a transcription elongation complex are mediated by protein-protein interactions involving the PcrA-CTD.
(A) EMSA supershift assays to monitor association of PcrA with a TEC. The PcrA-CTD is necessary and sufficient for stable formation of the PcrA-RNAP complex. WT PcrA and ΔCTD PcrA were titrated from 0.25 µM to 1.5 µM. The PcrA-CTD was titrated from 0.5 µM to 3 µM. (B) EMSA supershift assay showing that binding of PcrA is not dependent on the presence of upstream or downstream DNA in the TEC. The star indicates the position of the fluorescent label at the 5′ end of the template strand of the scaffold. PcrA was used at 1 µM. The oligonucleotides used to assemble the scaffolds are shown in Table 6. (C) Relative HDX measured for the PcrA-CTD in the CTD-RNAP complex compared to CTD alone. The black line shows the differential relative uptake and the pink and blue shadowing, the SEM of the CTD and CTD-RNAP conditions, respectively. The four offset traces show different exchange times. Negative uptake values over the CTD baseline with non-overlapping shadowing show protected amino acid regions on the CTD when it is in complex with RNAP. Note the key regions of the CTD (aa ~690–705 and aa ~720-Ct) are protected upon binding RNAP. (D) Left - homology model of the PcrA-CTD showing regions protected from HDX in the complex with RNAP (dark blue). Right - amino acids known to be important for interaction with RNAP (purple residues).
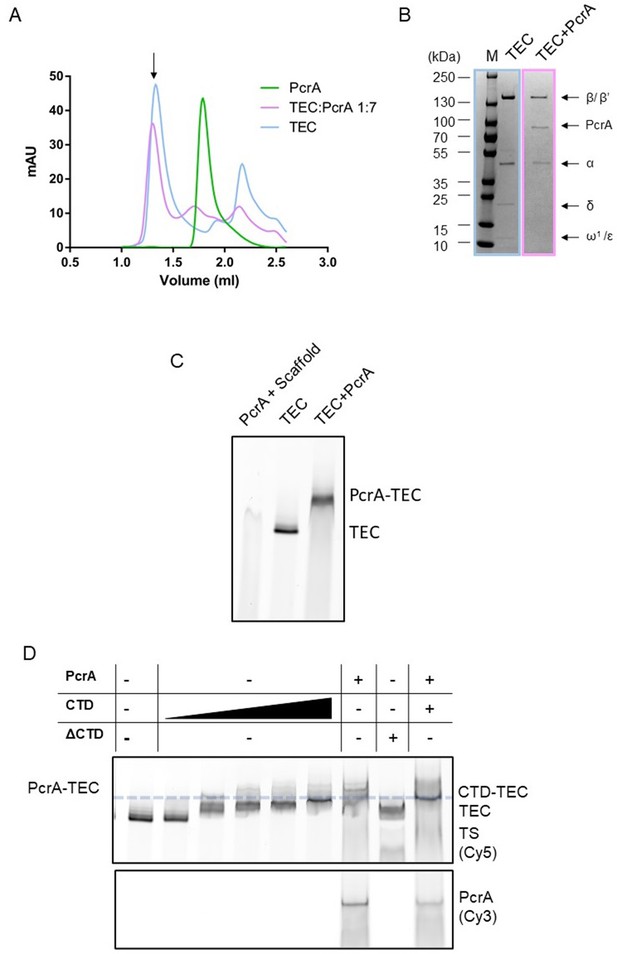
Interactions between PcrA and a transcription elongation complex are mediated by protein-protein interactions involving the PcrA-CTD.
(A) PcrA interacts physically with the TEC during size exclusion chromatography. Chromatographs are shown for free PcrA (green), the TEC alone (blue), and the TEC:PcrA mixture (magenta) at a 1:7 ratio. (B) SDS-PAGE gel showing samples taken from the size exclusion runs for TEC only (blue) and the TEC:PcrA mixture (magenta) at the position indicated by the arrow in (A). Note the presence of PcrA at an apparently very high molecular weight when the TEC is present, providing evidence for a physical interaction. (C) PcrA interacts physically with the TEC in native polyacrylamide gels. The gel shows a EMSA ‘supershift’ assay in which a fluorescently labelled transcription bubble scaffold is shifted by PcrA alone, RNAP alone (i.e. the TEC) and a mixture of RNAP and PcrA. PcrA was used at 1 µM concentration. Note that a unique supershifted band is formed in the final lane providing evidence for a PcrA-TEC complex. The free scaffold runs off the bottom of this gel due to the long electrophoresis time required to separate the shifted bands. (D) The CTD competes with FL PcrA for binding to the TEC in native polyacrylamide gels. The upper panel shows the TEC signal (the template strand is Cy5- labelled) and the lower panel, the FL PcrA (V448C mutant labelled with Cy3). The ΔCTD construct is not able to supershift the TEC. When CTD is added to the TEC-PcrA complex, the band shifts downwards to the expected position of the CTD-TEC complex. FL PcrA and CTD were used at 3 µM concentration. The CTD titration is the same titration gel shown in Figure 1A right panel. The dashed line shows the position of the CTD-TEC.
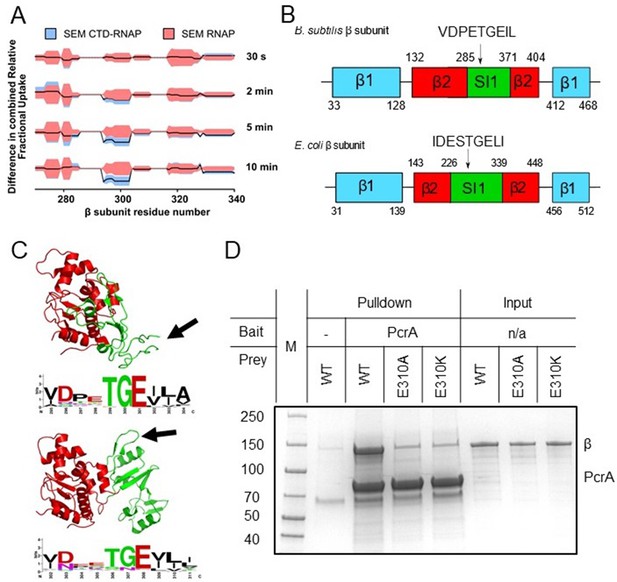
The PcrA-CTD binds to a conserved motif in the SI1 domain of RNAP.
(A) Relative HDX measured for a region of the RNAP β subunit (residue numbers on x axis) within the CTD-RNAP complex (blue) compared to RNAP alone (red). A small region of RpoB (at amino-acid positions around ~300) becomes significantly protected by interaction with the PcrA CTD as the exchange time becomes longer. (B) The protected region maps to a conserved motif in the SI1 domain of B. subtilis RpoB. This region is organised differently in E. coli RpoB, but the same conserved amino acid motif appears in a slightly different position in the structure (black arrow). (C) Structure of the B.subtilis (upper panel) and E. coli (lower panel) β2 (red) - SI1(green) domains indicating the beta-loop structure containing a putative interaction motif at the tip (black arrows). This sequence is well-conserved in bacterial RNA polymerases and the consensus sequence is shown in weblogo format beneath each structure. (D) In vitro pulldown of RpoB using PcrA as a bait (see Materials and methods for details). Mutation of the conserved glutamate (E301) in the putative helicase interaction motif dramatically reduces RpoB pulldown.
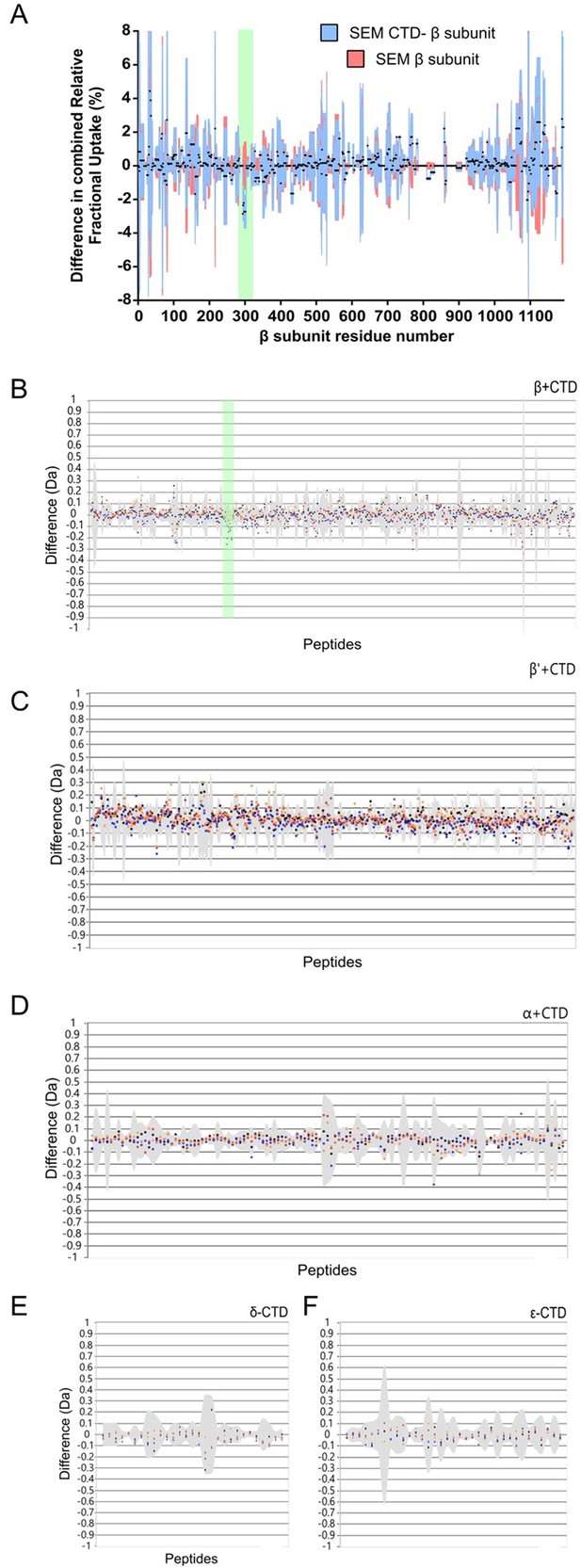
HDX protection plots for the remaining RNAP subunits in the CTD-RNAP experiment.
(A) Complete data for the β subunit shown in Paved format as used for Figure 2A. The green box indicates the protected area. (B–F) DYNAMX HDX butterfly plots for the β, β’, α, δ, and ε subunits of RNAP. Negative values represent protected regions and positive values represent regions that are exposed upon binding of the CTD. The green box in panel B indicates the region of β that is protected by the PcrA CTD. Note that there are no significant protection signals anywhere else in the entire RNAP complex. For difference plots, four replicates were performed for each of the four independent colour-coded time points (orange dots 0.5 min, red dots 2 min, blue dots 5 min, black dots 10 min). Grey shading indicates the standard deviation of all charge states and replicates per peptide.
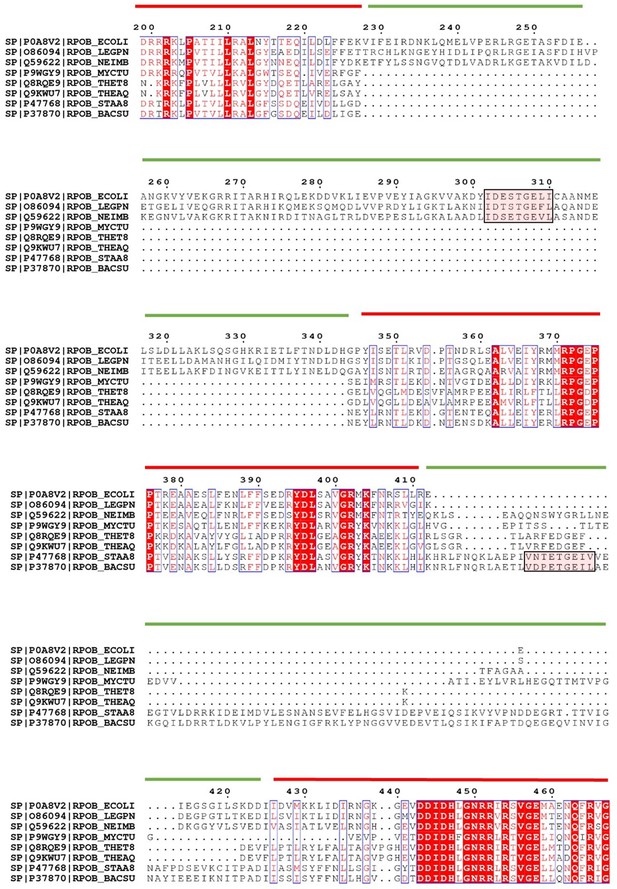
The CTD-interacting motif is located in different regions of the SI1 domain among landmark organisms.
A multiple sequence alignment for the β2 (red line) and SI1 (green line) domains of the RNAP β subunit for the organisms indicated. Pink shading indicates the putative helicase interaction motif which is positioned differently in E. coli-like compared to B. subtilis-like RNAP. Note that the motif was not clearly identified in all bacterial RNAPs.
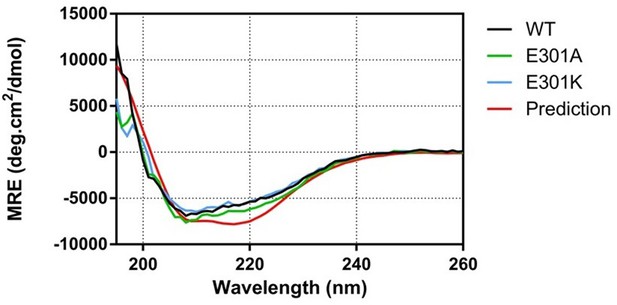
Mutations to E301 do not alter the overall structure of the β subunit of RNAP.
CD spectra for the proteins indicated were obtained at 0.25 mg/ml as described in the Materials and methods. The spectra are all similar and show a high α helical content as expected based on the cryo-EM structure (predicted spectrum shown as red line), suggesting that the wild type and mutant proteins are globally folded.
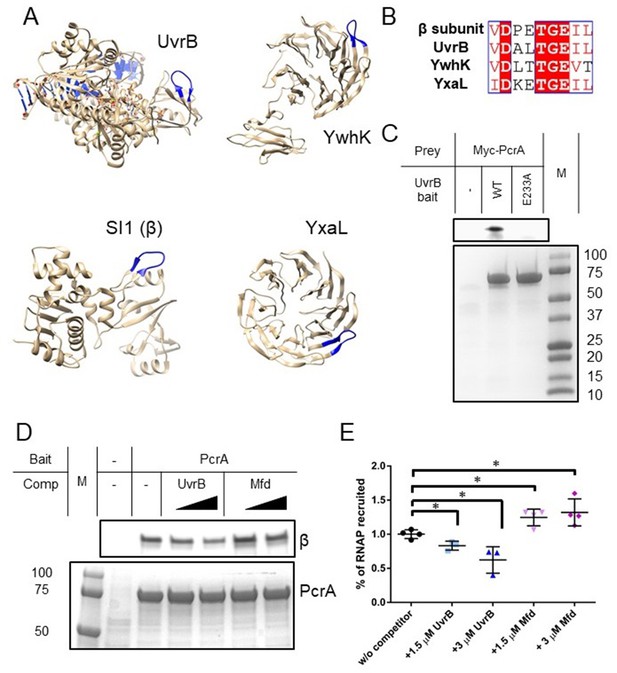
Many PcrA partner proteins contain the helicase interaction motif.
(A) Putative helicase interaction motif (blue) in known PcrA interaction partners from B. subtilis. A beta hairpin structure (blue) is formed by the interaction motif in each of the proteins. Two of the structures are homology models as indicated in the text. (B) Sequences of putative helicase interaction motifs in four known PcrA partner proteins. (C) Pulldown of Myc-tagged PcrA from B. subtilis cell extracts using UvrB as bait (for details see the Materials and methods). Mutation of the conserved glutamate (E233A) in the putative helicase interaction motif dramatically reduces PcrA pulldown. PcrA was detected using an anti-Myc antibody (upper gel). Equivalent loading of WT and mutant UvrB was confirmed by Coomassie staining (lower gel). (D) and (E) Pulldown of RNAP from B. subtilis cell extracts using biotinylated PcrA as bait. Where indicated the prey was supplemented with purified UvrB or Mfd. Free UvrB, but not Mfd, competes for the interaction site formed between PcrA and RNA polymerase. Error bars show the SEM of three independent experiments. Two-tailed Student’s t test determined statistical significance (*p value < 0.05).
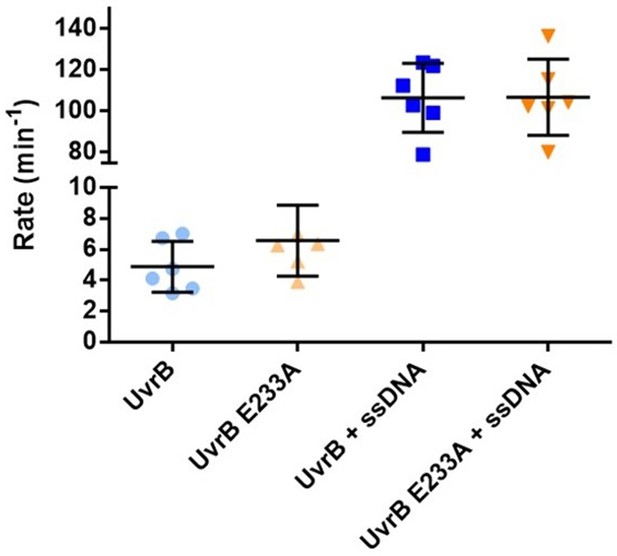
The E233A mutation does not affect the ATPase activity of UvrB.
ssDNA stimulated steady-state ATPase activity of WT and E233A UvrB was measured as described in the Materials and methods. The reported values are the mean turnover number and standard deviation for at least five independent experiments.
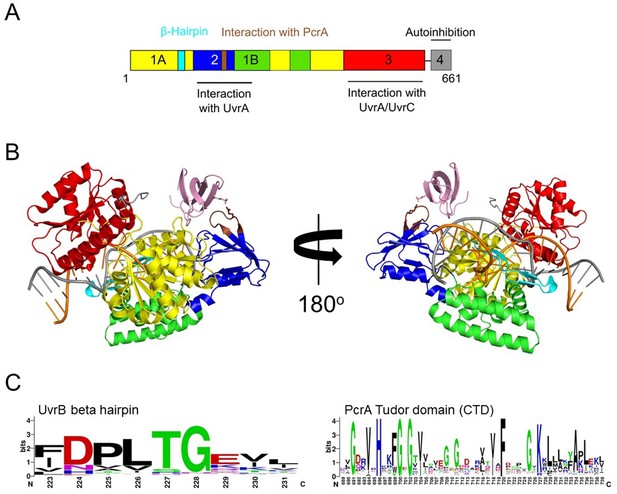
The CTD interacts with UvrB domain two and close to the damaged DNA site.
(A) Domain organisation of UvrB showing the interaction sites for UvrA, UvrC and the location of the PcrA/UvrD helicase interaction motif identified in this study. (B) Docking model generated by HADDOCK (van Zundert et al., 2016) showing DNA-bound UvrB (PDB: 6o8e; colours according to panel A) and the PcrA CTD (pink). The DNA strands are shown in orange and grey. (C) Sequence conservation logos for the helicase interaction motif in UvrB and the PcrA-CTD. Note that the glutamic acid is somewhat more variable than in the logo generated for the RNAP β subunit, although the interaction motifs are otherwise highly similar.
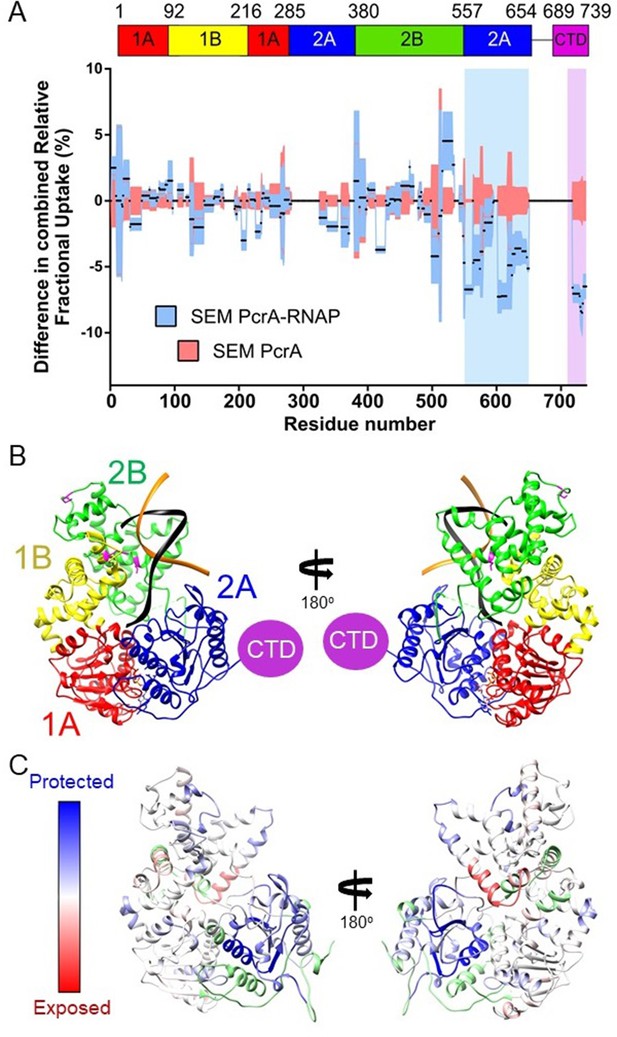
The PcrA helicase core is protected by interaction with RNA polymerase.
(A) Relative HDX measured for full length PcrA (residue numbers on x axis) in the PcrA-RNAP complex (blue) compared to PcrA alone (red). The magenta rectangle highlights strong protection of the CTD afforded by interaction with RNAP as expected based on results presented earlier in this manuscript. The blue rectangle highlights a second region of strong protection within the 2A domain of PcrA. (B) The PcrA helicase core (homology model from PDB: 3PJR) showing domain organisation. The DNA substrate is shown in black and orange and the CTD (which is disordered in this structure) is indicated as a purple circle. (C) The same structure showing the mapping of the HDX-protection data (bottom; blue indicates protection in the complex, red indicates exposure and green indicates a lack of data). Note that the HDX-protection data maps largely to one face of the helicase within domains 2A and, to a lesser extent, 2B.
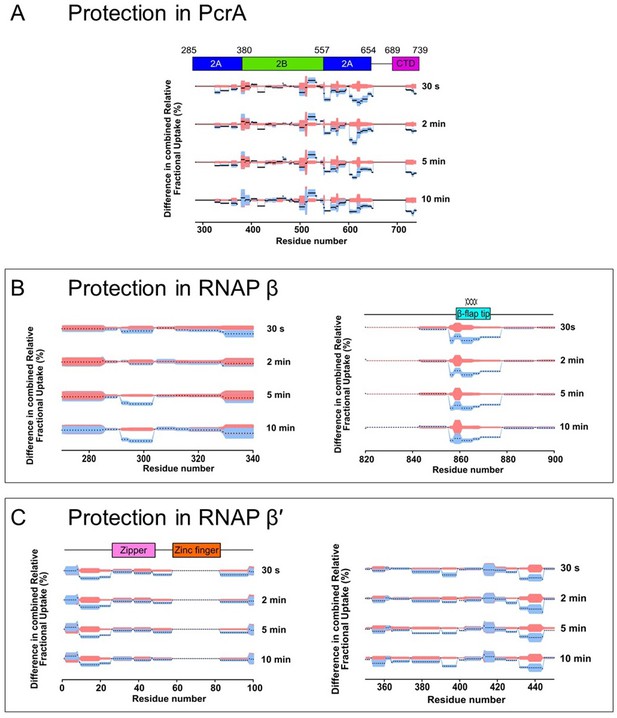
Deuterium uptake dynamics for regions of PcrA and RNAP that are significantly protected in the PcrA-RNAP complex.
The plots show all four timepoints for the protected regions in (A) PcrA, (B–C) the RNAP β subunit, with the spiral representing the β-flap tip region and (D–E) the RNAP β’ subunit.
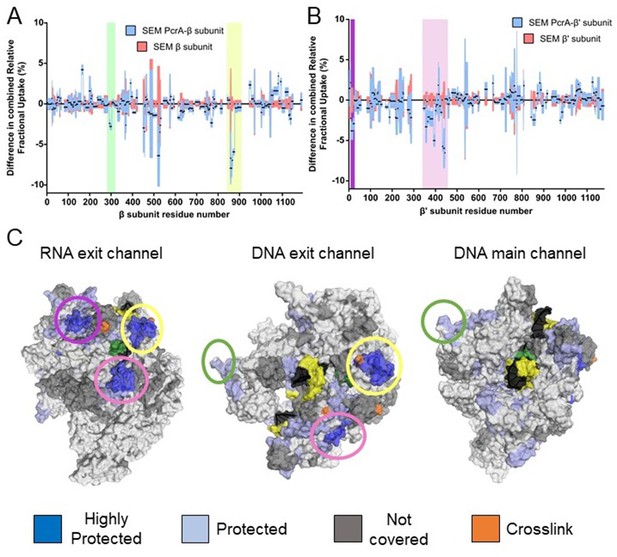
The PcrA helicase core binds to RNAP close to the RNA and DNA exit channel.
(A) Differential protection plot for the β subunit of RNAP showing the differential relative uptake at 10 min of exposure to deuterium for a PcrA-RNAP complex (blue) compared to RNAP alone (red). Green and yellow rectangles highlight regions of RpoB that are significantly protected in the complex state. (B) Differential protection plot for the β′ subunit of RNAP showing the differential relative uptake at 10 min of exposure to deuterium for a PcrA-RNAP complex compared to RNAP alone. Purple and pink rectangles highlight regions of RpoC that are significantly protected in the complex state. (C) Three views of the B. subtilis TEC (PDB: 6WVJ) showing regions protected by interaction with PcrA. DNA is shown in yellow and black. RNA is green. Coloured circles correspond to the protected regions highlighted in panels A and B. The green circle highlights the helicase interaction motif already identified as the site of binding of the PcrA-CTD. Note that the other major protection sites surround the DNA/RNA exit channels. The crosslinking sites (orange) are from reference (Epshtein et al., 2014).

HDX protection data for the remaining RNAP subunits in the PcrA-RNAP interaction experiment.
DYNAMX HDX butterfly plots for the (A) α and (B) ε subunits. Negative values represent protected regions and positive values, regions that are exposed upon binding of PcrA. For difference plots, four replicates were performed for each of the four independent colour-coded time points shown. Grey shading indicates the standard deviation of all charge states and replicates per peptide.
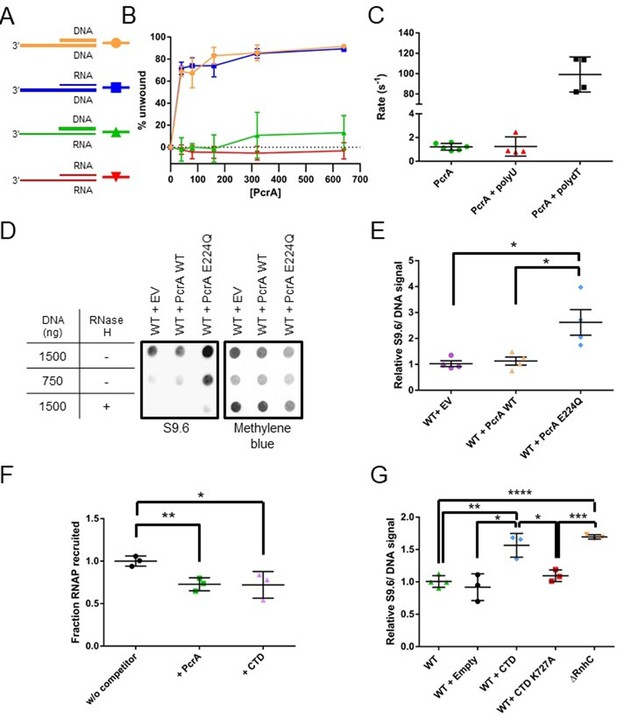
PcrA unwinds DNA-RNA hybrids in vitro and supresses R-loops in vivo (A) DNA and RNA substrates used for helicase assays.
Thick lines represent DNA strands and thin lines, RNA strands. The oligonucleotides used to form these substrates are shown in Table 7. (B) Quantification of unwinding as a function of PcrA concentration (in nM) for the 3′-tailed substrates shown in panel A. The substrate is only efficiently unwound if the longer of the two nucleic acids strands is DNA. Error bars show the standard deviation of at least three independent experiments. (C) The ATPase activity of PcrA is strongly stimulated by single-stranded DNA but not single-stranded RNA. Error bars show the standard deviation of at least three independent experiments. (D) Anti R-loop antibody (S9.6) dot blot for nucleic acid samples purified from three strains of B. subtilis. These strains contain an integrated expression cassette for either wild-type PcrA or a dominant negative form of PcrA (E224Q). The control strain (EV) contains an integrated but empty expression cassette. The S9.6 signal is normalised using methylene blue as a stain for all DNA. Note the high S9.6 signal for the strain expressing PcrA E224Q. (E) Quantification of four independent repeats of the experiment shown in (c). Error bars show the SEM. Expression of a dominant negative form of PcrA increases R-loop content (relative to DNA) in B. subtilis by ~2.5 fold. (F) Quantification of pulldown experiments of RNAP from B. subtilis cell extracts using biotinylated PcrA as bait and supplemented with purified PcrA WT or CTD. Addition of the CTD competes with WT PcrA to bind to RNAP. Error bars show the SEM of three independent repeats. (G) Relative R-loop levels in strains of B. subtilis expressing free CTD, a CTD mutant that interacts weakly with RNAP, or with a control expression cassette. A ΔrnhC strain is shown as a control for elevated R-loop levels. Error bars show the SEM of at least three independent experiments. In all panels, the statistical significance was determined using two-tailed Student’s t test (*p value < 0.05, **p value < 0.01, ***p value < 0.001, ****p value < 0.0001).
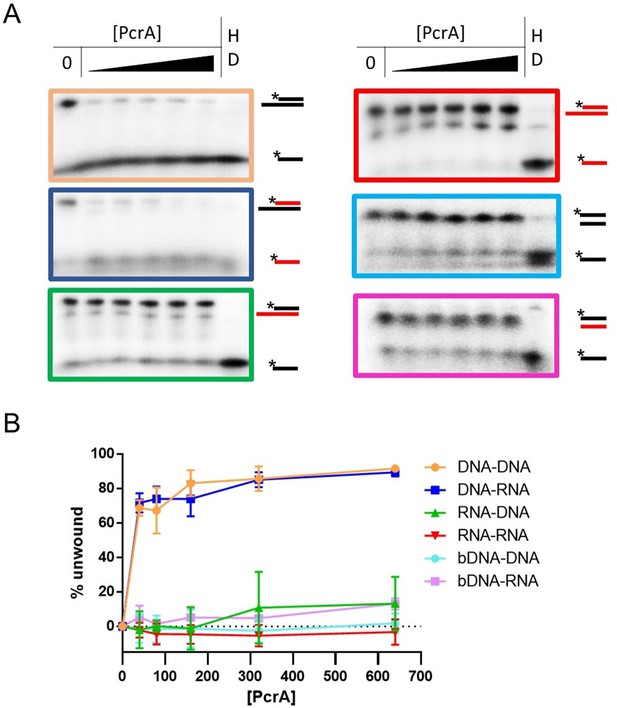
PcrA requires a 3′-ssDNA tail to unwind DNA duplexes.
(A) Representative helicase assays using 3′-tailed or duplex substrates consisting of annealed DNA (black) and RNA (red) oligonucleotides. The sequences of the oligonucleotides are in (Table 7). (B) Quantification of unwinding as a function of PcrA concentration (in nM) for 3′-tailed and fully duplex blunt-ended substrates (indicated by the prefix b). For the 3′-tailed substrates, the substrate is only efficiently unwound if the longer of the two nucleic acids strands is DNA. The fully duplex substrates are not unwound efficiently. Part of this data is reproduced from Figure 6 for comparison. Error bars show the standard deviation of at least three independent experiments.
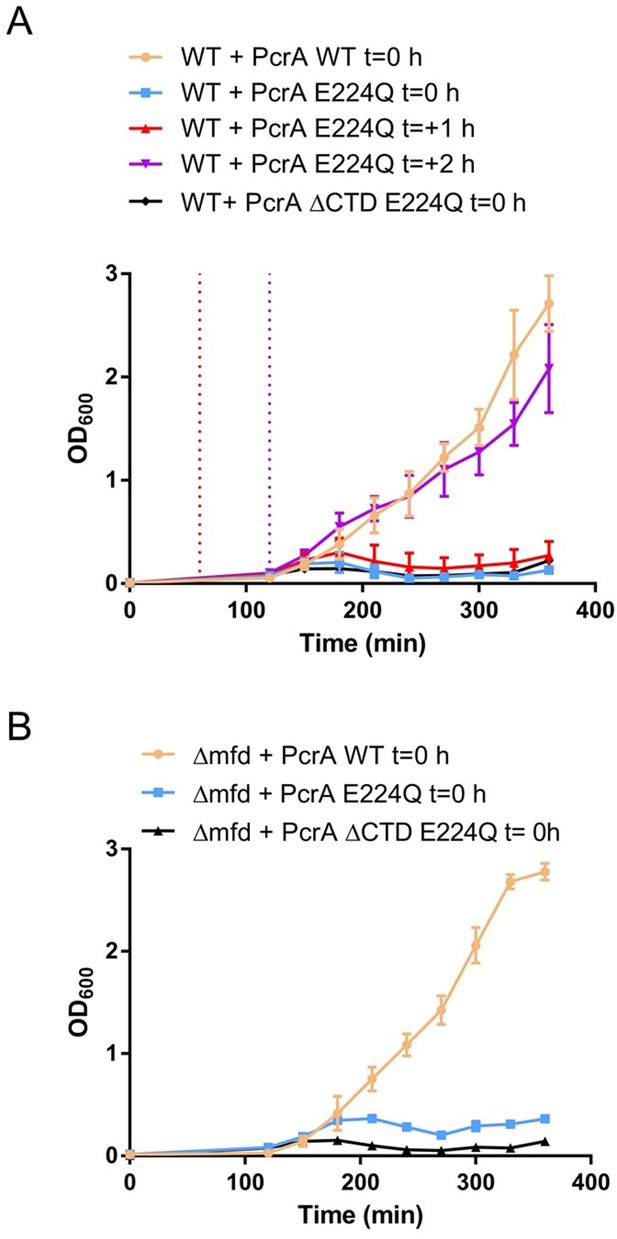
Overexpression of PcrA E224Q causes growth defects in WT and Δmfd B. subtilis.
Growth curves for (A) WT strains or (B) Δmfd strains overexpressing wild-type PcrA or E224Q at 37°C. The overexpression was induced with 1 mM IPTG at the indicated times. The overexpression cassette was integrated in the codirectional orientation. Three biological replicates were averaged at each time point and error bars represent the standard error of the mean.
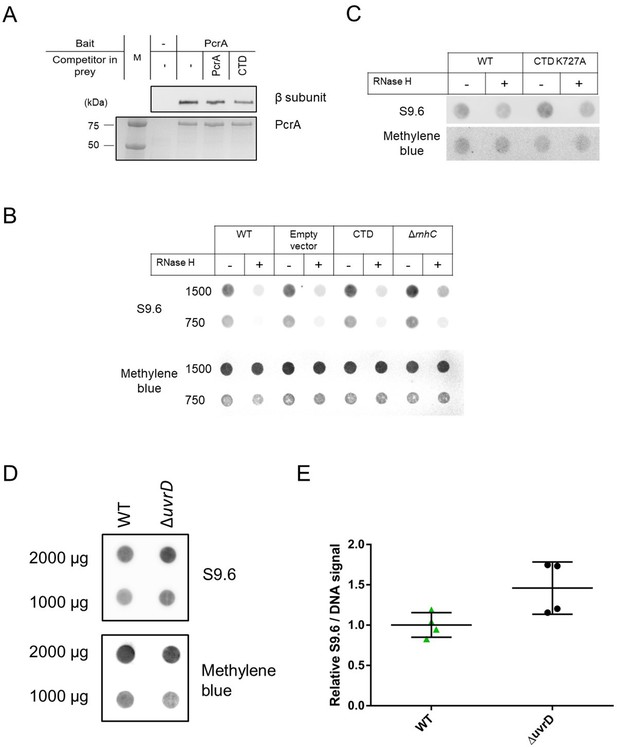
Overexpression of the PcrA CTD in B. subtilis and deletion of uvrD in E. coli increase R-loop levels in the cell.
(A) Representative anti-β subunit immunoblot (upper panel) and Coomassie-stained gel (lower panel) of a pulldown from B. subtilis extracts using biotinylated PcrA as bait. The gel shows that both free PcrA (full length) and free CTD can compete with the bait (tagged PcrA) to bind to RNAP. Where indicated, 1.5 µM untagged PcrA or CTD were added to the cell lysate. Quantification of these data is shown in Figure 6F. (B) and (C) Representative dot blots of B. subtilis CTD overexpression strains (as indicated) using the S9.6 antibody. Methylene blue was used as loading control. Quantification of these data is shown in Figure 6G. (D) and (E) Representative RNA/DNA hybrid dot blot of genomic DNA from the indicated E. coli strains and quantification. Error bars show the SEM of four independent experiments. In all panels, the statistical significance was determined using two-tailed Student’s t test (*p value < 0.05).
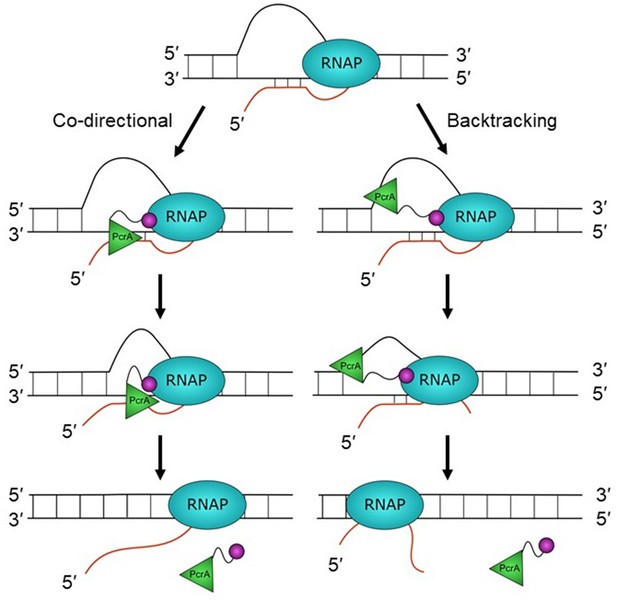
Hypothetical models for PcrA-dependent R-loop suppression during transcription.
R-loops form during transcription but lead to genomic instability and are therefore targeted for removal by PcrA. In the co-directional model, PcrA helicase (green) interacts with an R-loop-associated RNAP (blue) via the PcrA-CTD (magenta). It then engages the template DNA strand before translocating in the 3′>5′ direction and directly unwinding the DNA:RNA hybrid. In this model, R-loops may be unwound as they are formed if the helicase is in close contact with the TEC. In the backtracking model, PcrA binds to the displaced (non-template) strand behind RNAP and pulls it backwards, thereby unwinding the R-loop indirectly (see main text for further details and Discussion).
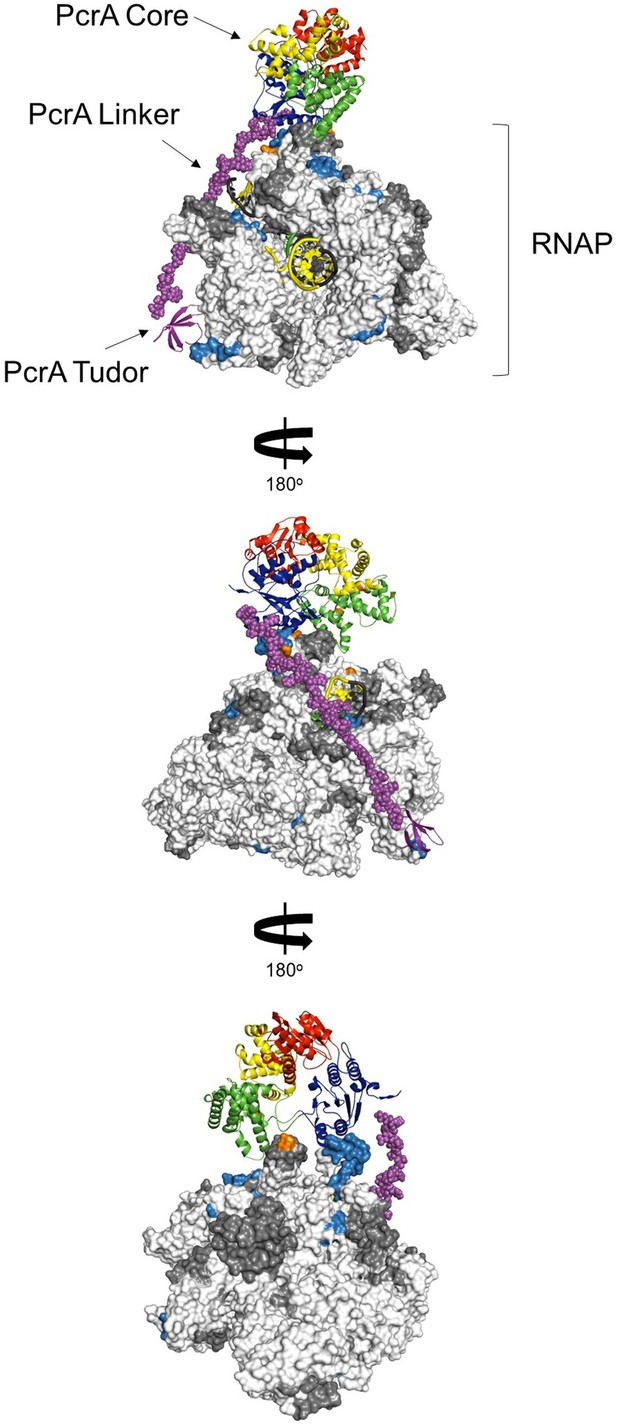
Working model for the interaction between PcrA and a TEC.
The figure shows the PcrA core (homology model from PDB: 3PJR) coloured by domain organisation (red, blue, green, and yellow) as in Figure 4B. This is joined to the PcrA-CTD (template PDB: 5DMA, purple ribbons) via an extended linker (manually modelled; purple spheres). The B. subtilis TEC (PDB: 6WVJ) with the addition of the β-flap tip (PDB: 6FLQ) and extended nucleic acids (PDB: 6ALF) is shown as a surface representation. Light blue and dark grey regions on the TEC show protected regions and regions for which there is no data, respectively, from the HDX-MS experiment. Orange residues show the crosslinks identified in the E. coli XL-MS experiment (Epshtein et al., 2014). The template strand is black, the non-template strand is yellow and the RNA is green. This simple model is intended to show that it is possible to satisfy the large majority of the protected regions we identify on RNAP by docking a single PcrA monomer. Importantly, note that the PcrA linker region is of an appropriate length to allow simultaneous binding of the PcrA helicase core to the DNA/RNA exit channels and the PcrA-CTD to the distant SI1 domain.
Tables
Sequence coverage and redundancy for the proteins analysed by HDX-MS.
Protein | CTD-RNAP complex | PcrA-RNAP complex | ||
---|---|---|---|---|
Sequence coverage (%) | Sequence redundancy | Sequence coverage (%) | Sequence redundancy | |
CTD/ PcrA | 87.2 | 3.61 | 78.5 | 2.38 |
α | 93.9 | 3.48 | 89.2 | 2.59 |
β | 83.7 | 2.84 | 84.9 | 2.24 |
β’ | 88.7 | 2.58 | 80.3 | 2.05 |
δ | 75.7 | 1.79 | 17.3 | 1.53 |
ε | 100 | 4.26 | 91.3 | 2.38 |
ω | - | - | - | - |
σA | 42.6 | 1.29 | - | - |
Mfd pulls down RNAP subunits from Bacillus subtilis cell extracts.
Proteins enriched in the biotinylated Mfd bait condition compared to the no-bait control pulldown (see Materials and methods for details). Subunits of RNAP that are enriched in the Mfd pulldown are indicated in bold text. Accession refers to the UniProt accession code, GN refers to gene name and FC, to fold change.
Accession | Description | LogFC |
---|---|---|
P37474 | Transcription-repair-coupling factor GN=mfd | 7.887 |
O34863 | UvrABC system protein A GN=uvrA | 6.127 |
O34628 | Uncharacterised protein YvlB GN=yvlB | 5.779 |
Q795Q5 | Uncharacterised membrane protein YttA GN=yttA | 3.735 |
O34942 | ATP-dependent DNA helicase RecG GN=recG | 3.304 |
Q06796 | 50S ribosomal protein L11 GN=rplK | 3.269 |
P20429 | DNA-directed RNA polymerase subunit alpha GN=rpoA | 2.899 |
O32006 | Resolvase homolog YokA GN=yokA | 2.898 |
O07542 | UPF0342 protein YheA GN=yheA | 2.800 |
O35011 | DNA-directed RNA polymerase subunit omega GN=rpoZ | 2.780 |
P39592 | Uncharacterised HTH-type transcriptional regulator YwbI GN=ywbI | 2.667 |
Q08792 | Uncharacterised HTH-type transcriptional regulator YcxD GN=ycxD | 2.654 |
O34949 | Uncharacterised HTH-type transcriptional regulator YkoM GN=ykoM | 2.600 |
O34381 | HTH-type transcriptional regulator PksA GN=pksA | 2.512 |
List of B. subtilis and E. coli strains used in this work.
Strain | Genotype | Reference | Plasmid to generate the strain | Parent strain |
---|---|---|---|---|
MH5636 | rpoC-His10::cat trpC2 | Qi and Hulett, 1998 | - | JH642 |
1a1 | trpC2 | Koo et al., 2017 | - | - |
IU79 | lacA::Pxyl-myc-PcrA::mls insb pMAP39 in pcrA | This work | pMAP39 (Petit et al., 1998) and pBS2EXylRPxylA(V2)- myc-PcrA | 1a1 |
IU6 | amyE::Phyperspank::spec HO trpC2 | This work | pDRIII (HO) | 1a1 |
IU35 | amyE::Phyperspank::spec CD trpC2 | This work | pDRIII (CD) | 1a1 |
IU41 | amyE::Phyperspank-mycPcrA::spec CD trpC2 | This work | pDRIII(CD)-mycPcrA | 1a1 |
IU56 | amyE::Phyperspank-mycPcrA-E224Q::spec CD trpC2 | This work | pDRIII(CD)-mycPcrA-E224Q | 1a1 |
BKK00550 | Δmfd:kan trpC2 | Koo et al., 2017 | - | 1a1 |
IU60 | amyE::Phyperspank::spec Δmfd:kan trpC2 | This work | pDRIII (CD) | BKK00550 |
IU61 | amyE::Phyperspank-myc-PcrA::spec Δmfd:kan trpC2 | This work | pDRIII(CD)-mycPcrA | BKK00550 |
IU62 | amyE::Phyperspank-myc-PcrA-E224Q::spec Δmfd:kan trpC2 | This work | pDRIII(CD)-mycPcrA-E224Q | BKK00550 |
IU65 | amyE::Phyperspank-myc-PcrAΔCTD-E224Q::spec trpC2 | This work | pDRIII(CD)-mycPcrAΔCTD-E224Q | 1a1 |
IU66 | amyE::Phyperspank-myc-PcrAΔCTD-E224Q::spec Δmfd:kan trpC2 | This work | pDRIII(CD)-mycPcrAΔCTD-E224Q | BKK00550 |
BKK28620 | trpC2 ΔrnhC::kan | Koo et al., 2017 | - | 1a1 |
IU3 | amyE::Phyperspank::spec(HO) trpC2 | This work | pDRIII(HO) | BKK28620 |
IU5 | amyE::Phyperspank-myc-CTD::spec (HO) trpC2 | This work | pDRIII(HO)-mycCTD | BKK28620 |
IU9 | amyE::Phyperspank-myc-CTD-K727A::spec (HO) trpC2 | This work | pDRIII(HO)-mycCTD-K727A | BKK28620 |
TB28 | E. coli ΔlacIZYA | Bernhardt and de Boer, 2004 | - | MG1655 |
N6632 | E. coli ΔuvrD::dhfr | Guy et al., 2009 | - | MG1655 |
Plasmids used in this work.
Plasmid name | Vector | Insert(s) | Reference |
---|---|---|---|
pET22b-PcrA | pET22b | PcrA | Gwynn et al., 2013 |
pET22b-bioPcrA | pET22b | BioPcrA | Gwynn et al., 2013 |
pET22b-PcrAV448C | pET22b | PcrA V448C | This work |
pET22b-PcrAΔCTD | pET22b | PcrAΔCTD | This work |
pET47b-CTD | pET47b | CTD | This work |
pET28a-rpoB | pET28a | RNAP β subunit | This work |
pET28a-rpoB-E310A | pET28a | RNAP β subunit E310A | This work |
pET28a-rpoB-E310K | pET28a | RNAP β subunit E310K | This work |
pET47b-UvrB | pET47b | UvrB | This work |
pET47b-UvrB-E233A | pET47b | UvrB E233A | This work |
pET22b-Mfd | pET22b | Mfd | This work |
pBS2EXyl-mycPcrA | pBS2EXylRPxylA (V2) pBS0EPliaI | mycPcrA | Popp et al., 2017 |
pDRIII(HO) | pDRIII(HO) | - | Fisher et al., 2017 |
pDRIII(CD) | pDRIII(CD) | - | This work |
pDRIII(CD)-mycPcrA | pDRIII(CD) | mycPcrA | This work |
pDRIII(HO)-mycPcrA | pDRIII(HO) | mycPcrA | This work |
pDRIII(CD)-mycPcrA-E224Q | pDRIII(CD) | PcrA-E224Q | This work |
pDRIII(CD)-mycPcrAΔCTD-E224Q | pDRIII(CD) | PcrAΔCTD-E224Q | This work |
pDRIII(HO)-mycCTD | pDRIII(HO) | mycCTD | This work |
pDRIII(HO)-mycCTD-K727A | pDRIII(HO) | mycCTD-K727A | This work |
pMAP39 | pBSspec+ | (nt 201 to 699 of pcrA) at HincII | Petit et al., 1998 |
Oligonucleotides used in this work for cloning (all sequences written 5′>3′).
Name | Sequence | Purpose |
---|---|---|
PcrA_V448C_F | AAGCGATTCAGCAGTGTGATTTTATCG | SDM |
PcrA_V448C_R | CGATAAAATCACACTGCTGAATCGCTT | SDM |
His-PcrA_CTD_XmaI_F | GACTCCCGGGAAAGAAACAAGAGCGACGTC | PcrA CTD (664-739) subcloning in pET47b |
BsuPcrA_BamHI_R | GATCGGATCCTTACTGCTTTTCAATAGGAGCAAATG | PcrA CTD (664-739) subcloning in pET47b |
PcrA_E224Q_F | CATCCACGTTGATCAGTATCAGGATACGAAC | SDM |
PcrA_E224Q_R | GTTCGTATCCTGATACTGATCAACGTGGATG | SDM |
BSuPcrA_deltaCTD_F | CCTAAATGAGAAATAAGAAACAAG | SDM |
BSuPcrA_deltaCTD_R | CTTGTTTCTTATTTCTCATTTAGG | SDM |
bsurpob_ndeI_F | CAGGTGCATATGTTGACAGGTCAACTAGTTCAGTATG | RpoB subcloning in pET28a |
bsurpob_xhoI_R | TTAATTCTCGAGTTATTCTTTTGTTACTACATCGCGTTC | RpoB subcloning in pET28a |
rpoB_E301A_F | GATCCTGAAACAGGAGCAATCCTTGCTGAAAAAG | SDM |
rpoB_E301A_R | CTTTTTCAGCAAGGATTGCTCCTGTTTCAGGATC | SDM |
rpoB_E310K_F | GATCCTGAAACAGGAAAAATCCTTGCTGAAAAAG | SDM |
rpoB_E310K_R | CTTTTTCAGCAAGGATTTTTCCTGTTTCAGGATC | SDM |
BSuUvrB_SmaI_F | AGCAGCCCGGGGTGAAAGATCGCTTTGAGTTAGTCTCGAAATATC | UvrB subcloning in pET47b |
BsuUvrB_XhoI_R | GCATCTCGAGTCATCCTTCCGCTTTTAGCTCTAAAAGTAAATC | UvrB subcloning in pET47b |
BsuUvrB_E233A_F | GCTGACAGGAGCAATTCTCGGCGAC | SDM |
BsuUvrB_E233A_R | GTCGCCGAGAATTGCTCCTGTCAGC | SDM |
bMfd_5′NdeI_F | TTAATCATATGGACAACATTCAAACCTTT | Mfd subcloning in pET22b |
bMfd_XhoI_3′_R | ATTAACTCGAGTTACGTTGATGAAATGGTTTG | Mfd subcloning in pET22b |
bMfd_mutA2586G_F | CCTGACGCGAAGGTAGCGTATGCGCATGGGAAAATG | SDM |
bMfd_mutA2586G_R | CATTTTCCCATGCGCATACGCTACCTTCGCGTCAGG | SDM |
bMfdmutT2361C_F | CGCGTACGCTGCACATGTCTATGCTTG | SDM |
bMfd_mutT2361C_R | CAAGCATAGACATGTGCAGCGTACGCG | SDM |
pDRIII-inver-upsR-BlpI | TACTTAGCTAAGCCTAACTCACATTAATTGCGTTGCG | Invert MCS in pDRIII |
pDRIII-inver-downsF-BamHI | TAATTTGGATCCCTAAGCAGAAGGCCATCCTG | Invert MCS in pDRIII |
CTD_HindIII_F | ATCGTAAGCTTAAAGAAACAAGAGCGACGTC | CTD subcloning in pDRIII |
CTD_SphI_R | GCTTTGCATGCTTACTGCTTTTCAATAGGAGCAAATG | CTD and PcrA subcloning in pDRIII |
myc-PcrA_5′SalI_RBS_F | CGTTGTCGACAGGAGGTATACATATGGAGCAAAAG | PcrA subcloning in pDRIII |
PcrA_K727A_F | CTGTCGGCGTGGCACGCCTGTTAGCAG | SDM |
PcrA_K727A_R | CTGCTAACAGGCGTGCCACGCCGACAG | SDM |
Oligonucleotides used in this work for assembling the TEC (all sequences 5′>3′).
Name | Strand | Modification | Sequence (5′−3′) |
---|---|---|---|
RNA I | - | - | AUCGAGAGG |
Standard scaffold | TS | 5′ Cy5 | TGTCACTTCGCCGTGTCCCTCTCGATGGCTGTAAG TATACT |
NTS | AGTATACTTACAGCCATCGAGAGGGACACGGCGAAGTG ACA | ||
Downstream gap in TS | TS | 5′ Cy5 | CGTGTCCCTCTCGATGGCTGTAAGTATACT |
NTS | AGTATACTTACAGCCATCGAGAGGGACACGGCGAAGTGACA | ||
Upstream gap in NTS | TS | 5′ Cy5 | TGTCACTTCGCCGTGTCCCTCTCGATGGCTGTAAGTATAC |
NTS | AGCCATCGAGAGGGACACGGCGAAGTGACA | ||
Short scaffold | TS | 5′ Cy5 | CGTGTCCCTCTCGATGGCT |
NTS | AGCCATCGAGAGGGACACG | ||
No duplex downstream | TS | 5′ Cy5 | CGTGTCCCTCTCGATGGCTGTAAGTATACT |
NTS | AGTATACTTACAGCCATCGAGAGGGACACG | ||
No duplex upstream | TS | 5′ Cy5 | TGTCACTTCGCCGTGTCCCTCTCGATGGCT |
NTS | AGCCATCGAGAGGGACACGGCGAAGTGACA |
Oligonucleotides used in this work for helicase assays (all sequences 5′>3′).
Oligo name | Type | Sequence (5′−3′) |
---|---|---|
IU_1 | DNA | GGGAGCCGGTCTGCGTCTGGTGTACTCTTCTGCTTTCTCG |
IU_1R | RNA | GGGAGCCGGUCUGCGUCUGGUGUACUCUUCUGCUUUCUCG |
IU_2 | DNA | CCAGACGCAGACCGGCTCCC |
IU_2R | RNA | CCAGACGCAGACCGGCUCCC |
IU_3 | DNA | GGGAGCCGGTCTGCGTCTGG |
IU_3R | RNA | GGGAGCCGGUCUGCGUCUGG |