pH-dependent 11° F1FO ATP synthase sub-steps reveal insight into the FO torque generating mechanism
Figures
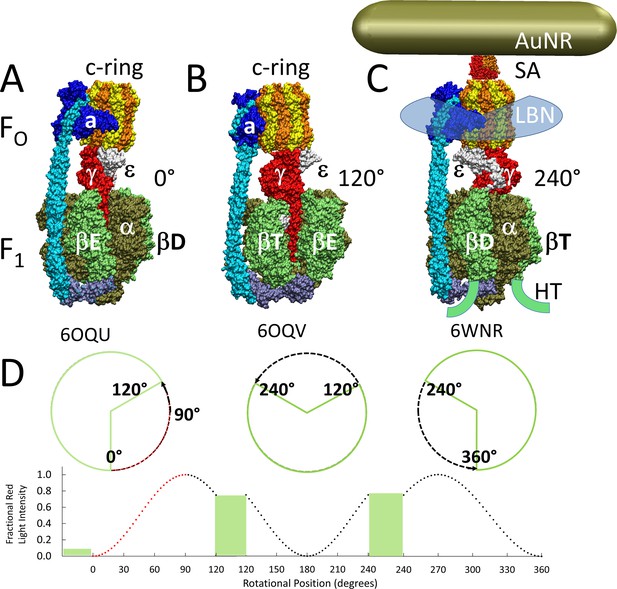
Cryo-EM structures of F1FO ATP synthase inhibited by ADP in three rotary states, and measurement of changes in rotational position between catalytic dwells.
(A) Rotational state-1, pdb-ID 6OQU (Sobti et al., 2020). (B) State-2, pdb-ID 6OQV, with rotor 120° counterclockwise (CCW) from (A) where subunit-α is not shown to reveal subunit-γ. (C) State-3, pdb-ID 6WNR, with rotor 240° CCW from (A) showing microscope slide assembly of F1FO embedded in a lipid bilayer nanodisc (LBN) for rotation measurements. His6-tags (HT) on β-subunit C-termini enabled attachment to slide, while the gold nanorod (AuNR) coated with streptavidin (SA) bound to the biotinylated subunit c-ring. (D) Rotational position of single F1FO molecules versus time was monitored by intensity changes of polarized red light scattered from the AuNR in the presence of 1 mM Mg2+ATP, which enabled F1-ATPase-dependent 120° CCW power strokes between catalytic dwells (green bars). Prior to data collection at 200 kHz, a polarizer in the scattered light path was rotated to minimize intensity during one of the three catalytic dwells. Light intensity increased to a maximum upon rotation by 90° during the subsequent CCW 120° power stroke. For each molecule the angular dependence of these power strokes versus time was analyzed.
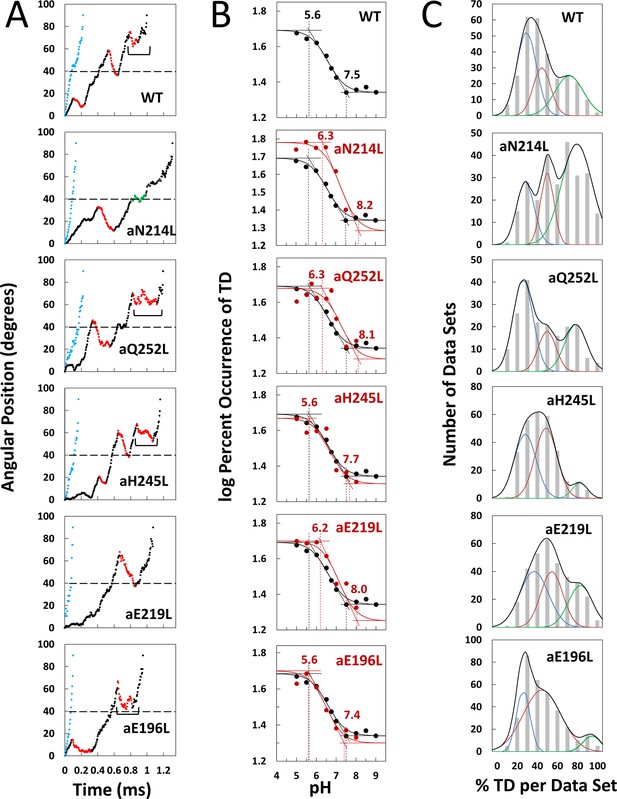
Effects of subunit-a mutations on transient dwells (TDs).
(A) Examples of power strokes without TDs (blue), and of power strokes with TDs that lacked (green), or contained clockwise (CW) synthase-direction c-ring rotation relative to subunit-a (red) plotted as degrees of rotation after the catalytic dwell versus time where 40° (dotted line) is the optimal position for binding of ATP or inhibitory ADP (Yasuda et al., 2001; Martin et al., 2014). Brackets indicate Brownian-type oscillations during a TD. (B) Average percent TDs per data set versus pH from which pKa values were derived via intercepts of the slope and plateaus (solid line) of each curve based on the fit of the data to Equation 1 for WT (black line) and subunit-a mutants (red line). (C) Distributions at pH 6.0 of the percent of TDs per data set of power strokes (gray bars) where multiple data sets that each contained ~300 power strokes were collected from each of the total number of the F1FO indicated, and data were binned in 10% increments. The data were fit to the sum of three Gaussians (black line) representing low (blue), medium (orange), and high (green) efficiencies of TD formation.
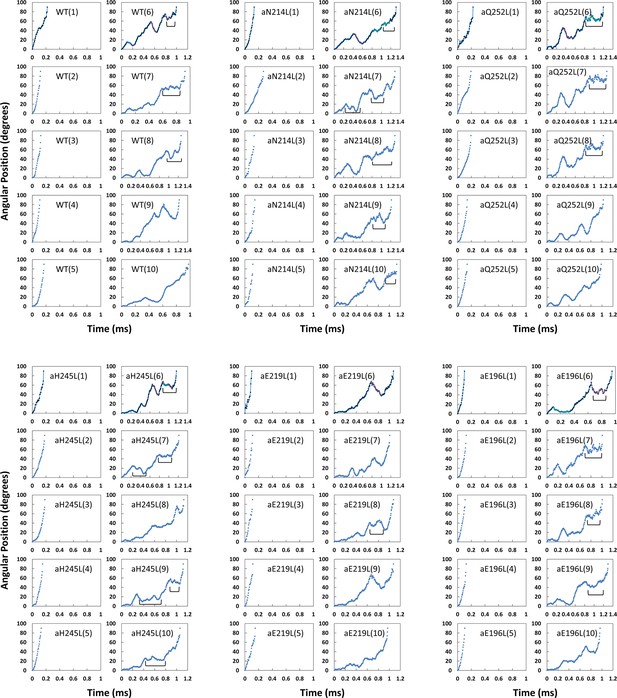
Examples each of the first 90° of ATP hydrolysis-driven power strokes observed using FOF1 nanodiscs.
In each mutant, examples 1–5 show power strokes without transient dwells (TDs). Examples 6–10 show power strokes with transient dwells where the FO motor either halts counterclockwise (CCW) rotation or caused clockwise (CW) rotation in the ATP synthase direction (synthase-direction steps). Brackets indicate Brownian-type oscillations during a TD.
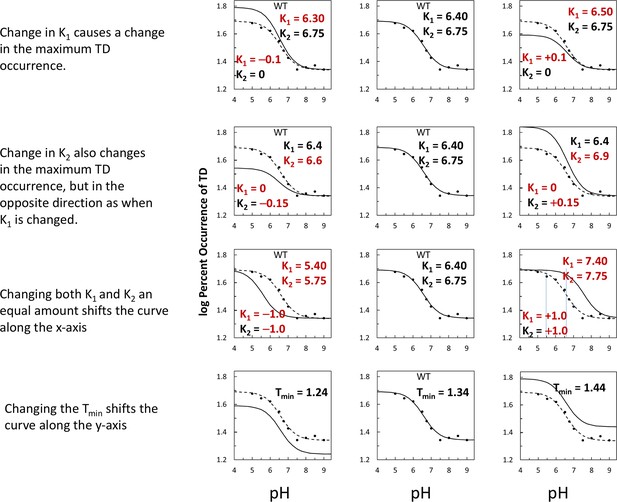
Examples of how changes in the variables in Equation 1 affect the log-log plots that describe the F1-ATPase inhibition kinetics of Figure 2C.
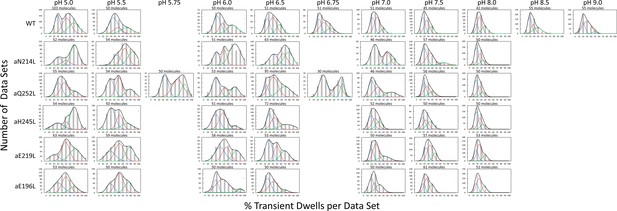
Distribution of power stroke data sets (each set containing ~300 power strokes) at each pH examined versus the percentage of the occurrence of transient dwells (TDs) per data set binned to each 10% (gray bars).
The data were fit to the sum of three Gaussians (—) representing low (—), medium (—), and high (—) efficiencies of TD formation. Ten data sets were acquired from each molecule, and the number of molecules examined is shown for each condition.
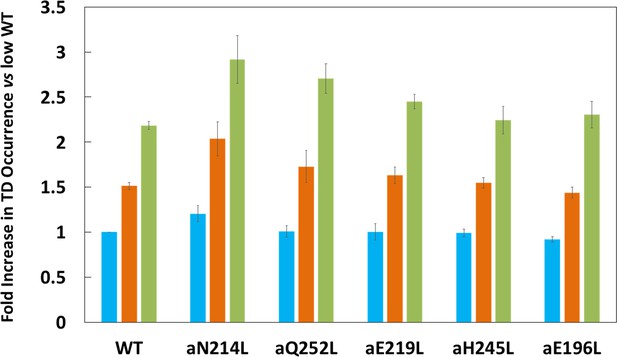
Proportion of low (blue bar), medium (orange bar), and high (green bar) transient dwell (TD) formation efficiencies relative to WT low efficiency TD formation.
Each was the average of all pH values examined (Figure 2—figure supplement 3). All of the low, medium, and high efficiences of TD formation are shown in that supplementary figure from which the averages were taken to calculate Figure 3. Vertical bars represent standard error.
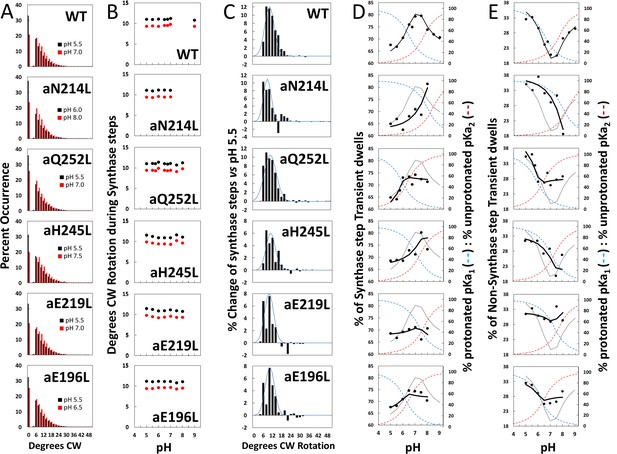
Effects of subunit-a mutations on the pH dependence of the extent of clockwise (CW) synthase-direction step rotation and fraction of transient dwells (TDs) containing synthase-direction steps.
(A) Distributions of the extent of CW rotation in the ATP synthesis direction during transient dwells for WT and subunit-a mutants at the low (black) and high (red) pH values indicated. (B) Mean (black) and median (red) extents of CW rotation during a synthase-direction step versus pH. (C) Distributions of the difference in extent of CW synthase-direction step rotation between pH values in Figure 2D when the percent of synthase-direction steps was maximum versus minimum where the blue line is the Gaussian fit. (D) Percent of TDs containing CW synthase-direction steps versus pH, where the data were fit to Equation 3 (black line). The fit for WT is shown as a gray line in the mutant plots. The fraction of protonated groups with pKa1 (blue line), and unprotonated groups with pKa2 (red line) versus pH was calculated from the pKa values of Table 2. (E) Percent of TDs that lack synthase-direction steps versus pH where the probability of forming a TD without a synthase-direction step (black line) was determined by Equation 2 from the fraction of protonated groups with pKa1 (blue line), and unprotonated groups with pKa2 (red line) versus pH calculated using pKa values from Table 2. The fit for WT is shown as a gray line in the mutant plots.
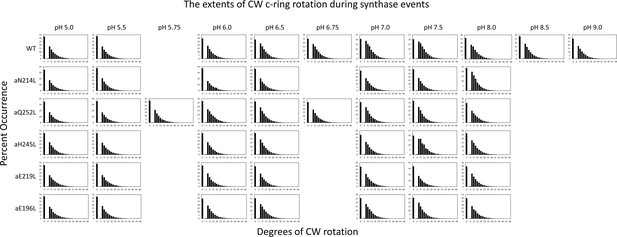
Distributions of the extent of clockwise (CW) rotation in the ATP synthesis direction during transient dwells for WT and subunit-a mutants versus pH.
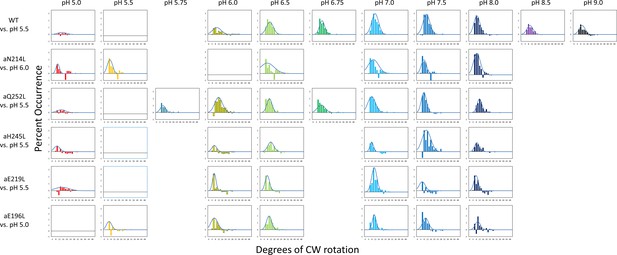
Distributions of the difference in extent of clockwise (CW) synthase-direction step rotation between pH values when the percent of synthase-direction steps was maximum versus minimum, where (—) is the Gaussian fit.
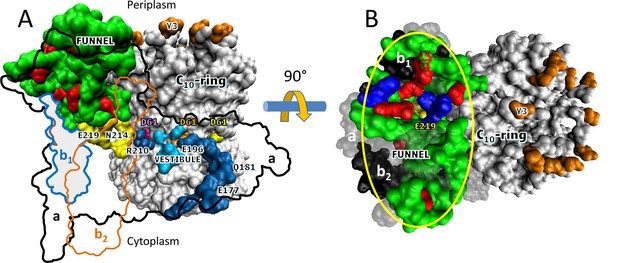
Aqueous funnel of charged and polar groups can serve as an antenna to supply protons to the input channel.
(A) Transmembrane view of Escherichia coli FO (pdb-ID 6OQR) showing the path of charged and polar residues across the membrane. Outlines indicate space occupied by hydrophobic residues in subunit-a (black line), subunit-b1 (blue line), and subunit-b2 (orange line). The inner surface of the funnel, which is lined with polar residues and loop regions (green), acidic groups (red), and histidines (blue) from subunit-a and the subunit-b1 N-terminus, is exposed to the periplasm at its wide end that narrows to aE219 (yellow) at the bottom. The input channel (yellow) extends from aE219 to aN214 and aQ252, which are proximal to aR210. Between aR210 and the output channel (dark blue) the leading (pink) and lagging (orange) cD61 groups rotate through a vestibule lined above and below the cD61 rotation plane by polar sidechains (light blue) that decrease the dielectric constant of the vestibule from that of the lipid bilayer. A protonated cD61 exposed to the lipid bilayer (yellow) is also visible. (B) Periplasmic surface of FO showing the interior surface of the funnel (orange oval) lined with charged and polar groups from subunit-a and subunit-b1 as in A that narrows to aE219 (yellow) at the bottom where the input channel begins. Hydrophobic residues are shown of subunit-a (gray), subunits-b1 and -b2 (black), and the c-ring (white). The cY3 sidechains (orange) are shown to indicate the orientation of the periplasmic surface of the c-ring.
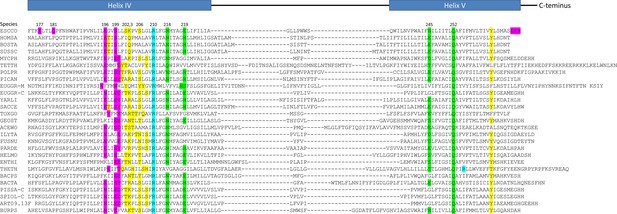
Sequence comparisons of the subunit-a helices that contact the c-ring.
Sequences were aligned relative to essential Escherichia coli residues aR210 (blue) in the penultimate helix (helix IV), and with highly conserved aQ252 in the ultimate helix (helix V). Residues associated with the input channel (green), the output channel (pink), and the vestibule (yellow). Sequences are from the following species with their names indicated on the left: ESCCO, Escherichia coli; HOMSA, Homo sapiens; BOSTA, Bos taurus; SUSSC, Sus scrofa; MYCPH, Mycobacterium phlei; TETTH, Tetrahymena thermophila mitochondrial; POLPR, Polytomella sp. Pringsheim 198.80 mitochondrial; PICAN, Pichia angusta mitochondrial; EUGGR-M, Euglena gracilis mitochondrial; EUGGR-C, Euglena gracilis chloroplast; YARLI, Yarrowia lipolytica mitochondrial; SACCE, Saccharomyces cerevisiae mitochondrial; TOXGO, Toxoplasma gondii; GEOST, Geobacillus stearothermophilus; ACEWO, Acetobacterium woodii (Na+); ILYTA, Ilyobacter tartaricus (Na+); FUSNU, Fusobacterium nucleatum; PARDE, Paracoccus denitrificans; HELMO, Heliobacter modesticaldum unicellular photosynthetic; ENTHI, Enterococcus hirae (V/A-type Na+); THETH, Thermus thermophilus (V/A-type); BACPS, Bacillus pseudofirmus OF4 (Alkalihalobacillus pseudofirmus); BACTA, Bacillus sp. Strain TA2.A1 (thermoalkalophilic); PISSA-C, Pisum sativum chloroplast; SPIOL-C, spinacia oleracea chloroplast, ART09.13F, Arthrospira sp. 09.13 F; BURPS, Burkholderia pseudomallei.
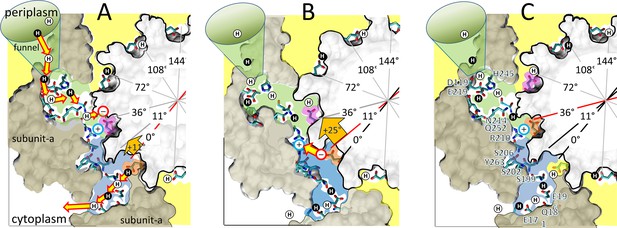
Alternating 11° and 25° sub-steps that power FO c-ring ATP synthase-direction rotation.
(A) The pH-dependent 11° sub-step occurs when H+ transfer from aN214/aQ25-bound water to the unprotonated leading cD61-carboxyl (pink), and from the protonated lagging cD61-carboxyl (orange) to aS199/aE196-bound water. Upon displacement from aR210 by protonation, leading cD61 adopts the closed conformation to enable rotation into the lipid bilayer (yellow). Due to coherent H+ movement in the Grotthuss column, each H+ entering the input channel (green) from the funnel causes a H+ to exit the output channel (blue) to the cytoplasm. Rotation occurs when lagging cD61 is deprotonated because the negatively charged carboxyl moves in response to the decrease in hydrophobicity from the lipid bilayer to the water-containing vestibule (blue), and from the electrostatic attraction to aR210. This decreases the distance between the lagging cD61 carboxyl and the aR210-guanidinium from ~11.5 to ~7.5 Å. (B) The 25° sub-step occurs primarily from the electrostatic interaction between the lagging cD61 carboxy (orange) and the aR210-guanidinium. (C) Electrostatic attraction decreases the distance between orange cD61 and aR210 from ~7.5 to ~3.5 Å to complete a 36° stepwise c-ring rotation, which positions the orange cD61 to become the leading carboxyl for the next pH-dependent 11° sub-step. Escherichia coli F1FO cryo-EM structures of rotary sub-states pdb-IDs 5OQS (A and C), and 5OQR (B) are shown as cross-sections of FO with hydrophobic resides of subunit-a (brown) and the c-ring (gray) along the plane defined by cD61 groups as viewed from the periplasm. Protons are alternately colored black and white to show the progression of proton transfer events.
Tables
pKa values and inhibition constants for WT and subunit-a mutants.
Values were derived from the fits to Equation 1 of the average percent of TDs per data set versus pH in Figure 2C.
K1 | K2 | Tmin (%) | pKa1 | pKa2 | |
---|---|---|---|---|---|
WT | 6.4 | 6.75 | 22.0 | 5.6 | 7.5 |
aN214L | 7.0 | 7.50 | 19.1 | 6.3 | 8.2 |
aQ252L | 7.0 | 7.40 | 19.5 | 6.3 | 8.1 |
aE219L | 6.9 | 7.35 | 17.8 | 6.2 | 8.0 |
aH245L | 6.5 | 6.87 | 20.0 | 5.6 | 7.7 |
aE196L | 6.3 | 6.70 | 20.0 | 5.6 | 7.4 |
pKa values and probabilities of forming transient dwells (TDs) without synthase-direction steps for WT and subunit-a mutants.
Values were derived from the fits of the data of Figure 4C to Equation 2.
pKa1 | P1 (%) | pKa2 | P2 | |
---|---|---|---|---|
WT | 6.5 | 38 | 7.7 | 33 |
aN214L | 8.0 | 37 | 8.4 | 5 |
aQ252L | 5.9 | 42 | 6.4 | 28 |
aE219L | 7.1 | 32 | 7.4 | 35 |
aH245L | 7.3 | 33 | 7.7 | 22 |
aE196L | 6.2 | 34 | 6.5 | 28 |