Analyzing the brainstem circuits for respiratory chemosensitivity in freely moving mice
Figures
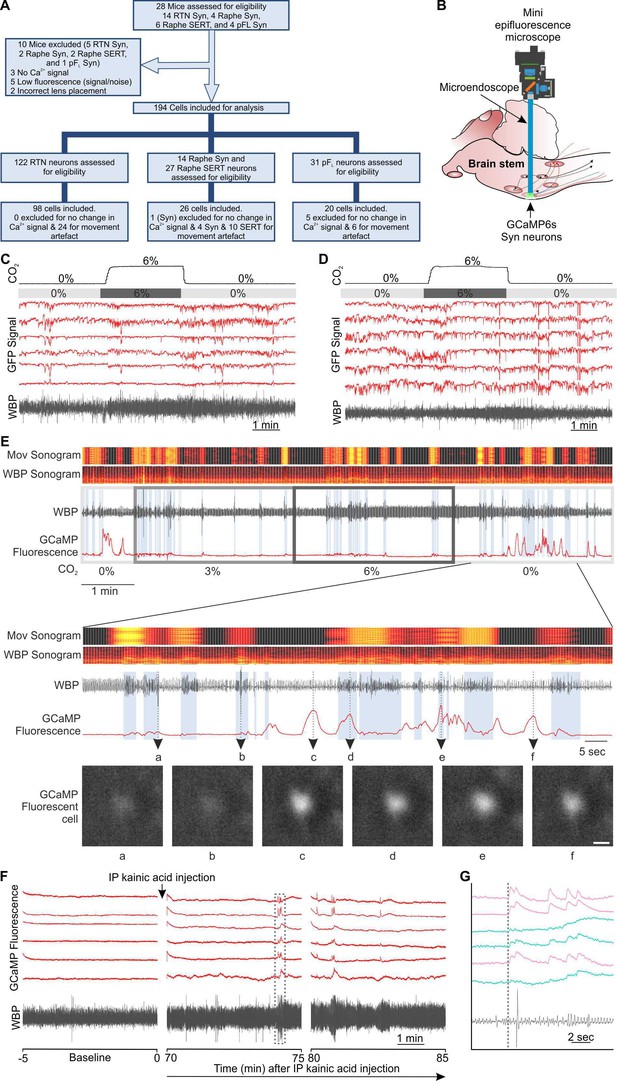
Experimental approach and movement artefact.
(A) A CONSORT flow diagram for inclusion/exclusion of experiments and cells from the study. (B) Representation of GRIN lens (microendoscope), baseplate, and mini epifluorescence camera placement for recording of brainstem nuclei. (C–D) GFP control. RTN neurons were transduced with AAV-Syn-GFP (not Ca2+ sensitive) and GRIN lens implanted. Fluorescence was recorded going from 0 to 6% inspired CO2 (gray bar). WBP: whole body plethysmography trace showing breathing movements. No signals are seen that resemble the Ca2+ transients observed with GCaMP6. (E) Comparison of Ca2+ fluorescence signals observed with GCaMP6 versus movement from WBP trace (WBP sonogram) and head movement as observed from video recording (Mov sonogram). In expanded traces- a,b, Examples of lack of fluorescence signal during movement of head/body; c,f, examples of fluorescence signal in the absence of movement; d,e, examples of fluorescence signals during movement. (Scalebar- 20 μm) (F) Seizures caused increase in activity of RTN neurons that was corelated with changes in breathing in anaesthetized mouse. Changes in mouse WBP before and after induction of non-behavioural seizures with intraperitoneal injection of kainic acid time matched with the activity of RTN neurons (red). The dotted square is changes in activity of neurons (ROIs) time matched with WBP and expanded in panel G. (G) Increase in the activity of RTN neurons correlated with changes in breathing. The start of the GCaMP6 transient is shown by the dotted line. Ca2+ transients with a fast rise and exponential fall (pink traces); and slower sustained changes (turquoise traces).
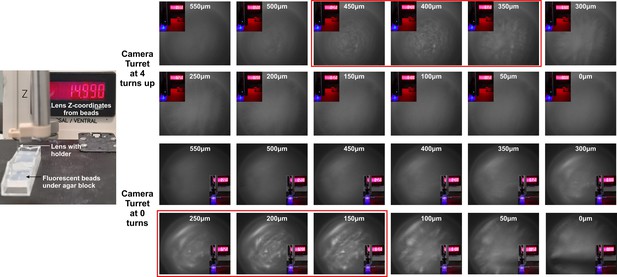
The depth of focus of the GRIN lens.
The focal plane and depth of GRIN lens from the fluorescent beads when camera turret was at the maximum (4 turns) high and at maximum low (0 turns) positions.
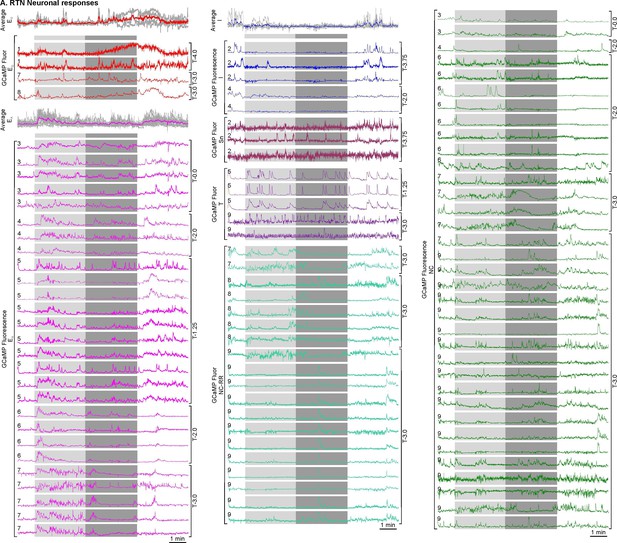
All RTN neurons responses to the hypercapnic challenge.
RTN excited graded (EG), excited adapting (EA), inhibited (I), sniff-coding (Sn), non-coding (NC), tonically active (T), and non-coding respiratory related (NC-RR) neuronal responses time-matched with hypercapnia (light grey-3% CO2, medium grey-6% CO2) and average waveforms of EG, EA, and I. Animal number is mentioned on the left hand side of the neuronal trace and camera turret position on the right hand side of the neuronal traces. All recordings of neuronal activity from a particular mouse are taken from the same imaging session for that mouse.
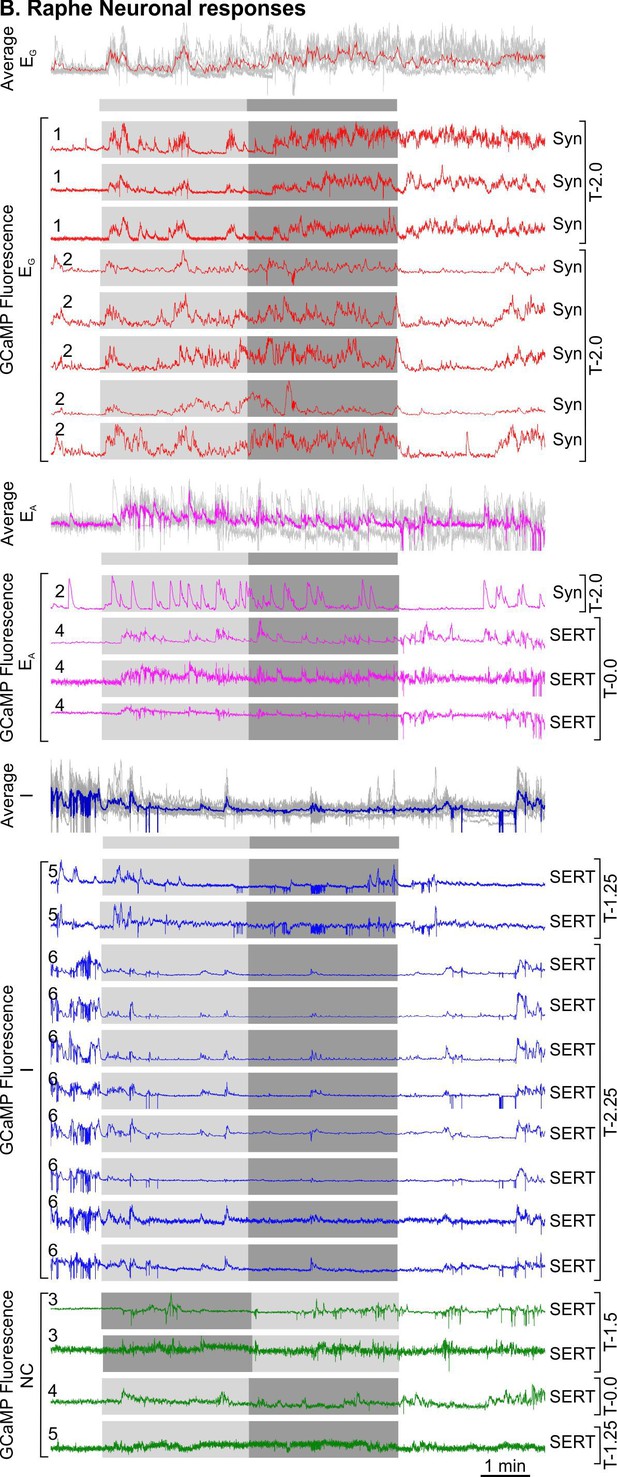
All raphe neurons responses to the hypercapnic challenge.
Raphe excited graded (EG), excited adapting (EA), inhibited (I), and non-coding (NC) neuronal responses time-matched with hypercapnia (light grey-3% CO2, medium grey-6% CO2), and average waveforms of EG, EA, and I. Animal number is indicated on the left hand side of the neuronal trace, and neuronal promoter and camera turret position on the right hand side of the neuronal traces. All recordings of neuronal activity from a particular mouse are taken from the same imaging session for that mouse.
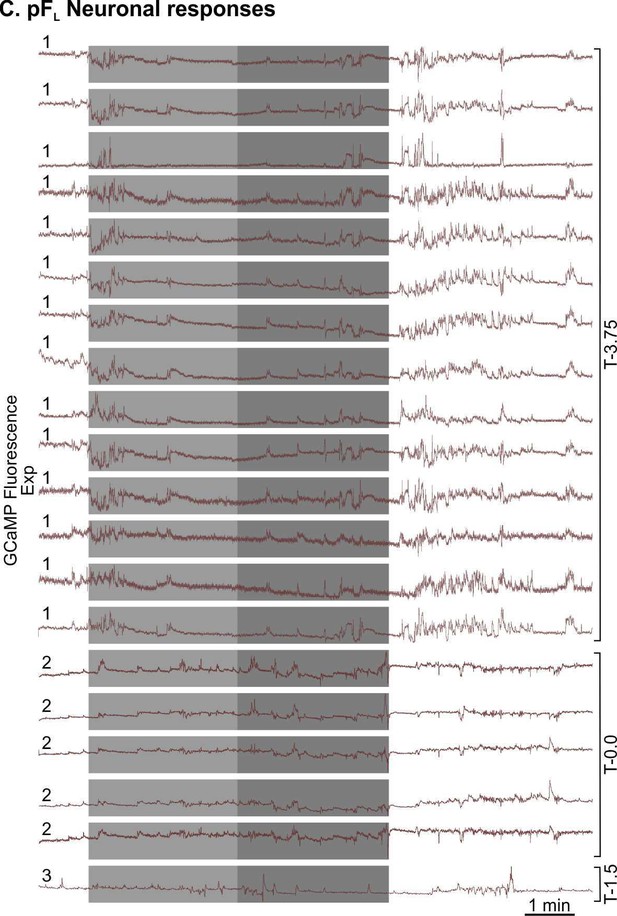
All pFL neuronal responses to the hypercapnic challenge (medium grey-6% CO2, dark grey-9% CO2).
Animal number is indicated on the left hand side of the neuronal trace and camera turret position on the right hand side of the neuronal traces. All recordings of neuronal activity from a particular mouse are taken from the same imaging session for that mouse.
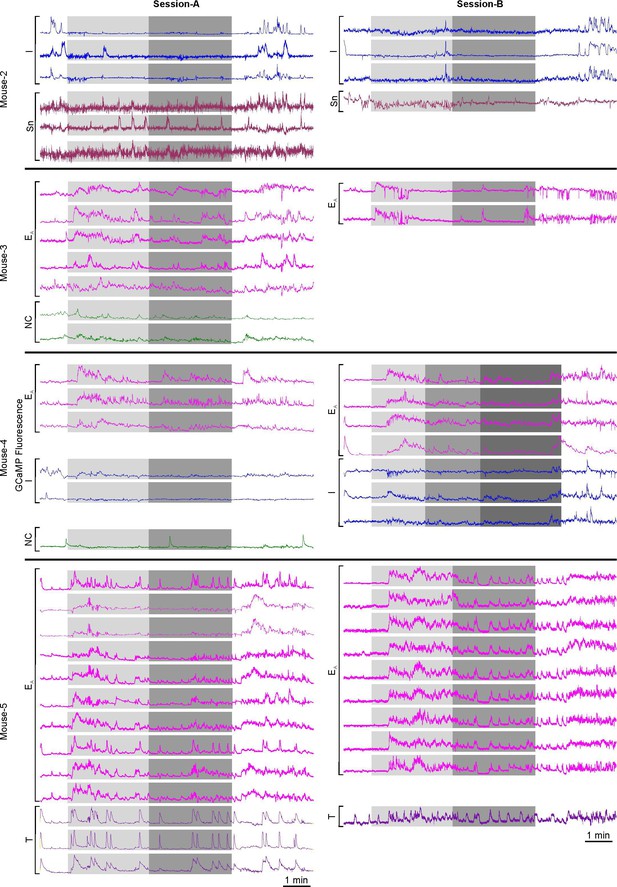
Consistent responses to hypercapnia in RTN neurons were recorded between sessions from the same mouse.
Session-A represents the recordings that contribute to the RTN neuronal dataset in Figure 1—figure supplement 2. Session-B represents recordings from a different session from the same mice. These recordings are not included in the dataset to avoid pseudoreplication but show that the same types of neuronal responses were consistently observed between sessions.
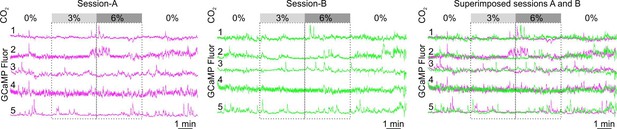
Activity patterns of individual RTN neurons remains very similar across recording sessions.
Left and right panels are different recordings sessions of the same neurons identified between different recording sessions from the same mouse on different days. The numbers represent neuron identification.
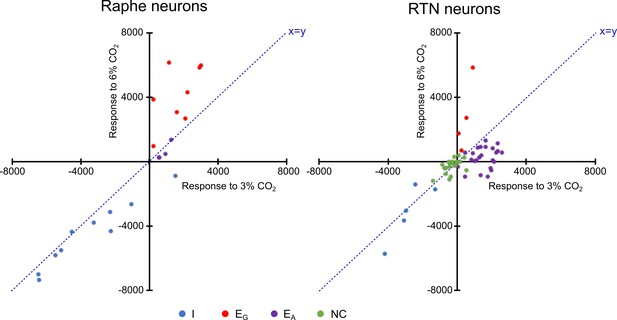
Two component analysis of neuronal categorisation in Raphe and RTN nuclei.
The change in Ca2+ signal (measured as area under the curve) from baseline (room air) elicited by 6% CO2 plotted against the Ca2+ response elicited by 3% CO2. The line of identity (x=y) is shown. EA neurons fall below this line, whereas EG neurons fall above this line. NC neurons are clustered around the origin (shown only for the RTN nucleus) and I neurons are predominantly in the negative quadrant of the graph.
-
Figure 1—figure supplement 7—source data 1
Data for Figure 1—figure supplement 7.
- https://cdn.elifesciences.org/articles/70671/elife-70671-fig1-figsupp7-data1-v2.xlsx
RTN neuronal responses to hypercapnia in mice transfected with synapsin GFP control (without GCaMP).
The dimensions of the fluorescence video are 596 x 531 µm (width x height).
Mouse undergoing kainate induced electrographic seizures in absence of physical seizures.
The dimensions of the fluorescence video are 900 x 650 µm (width x height).
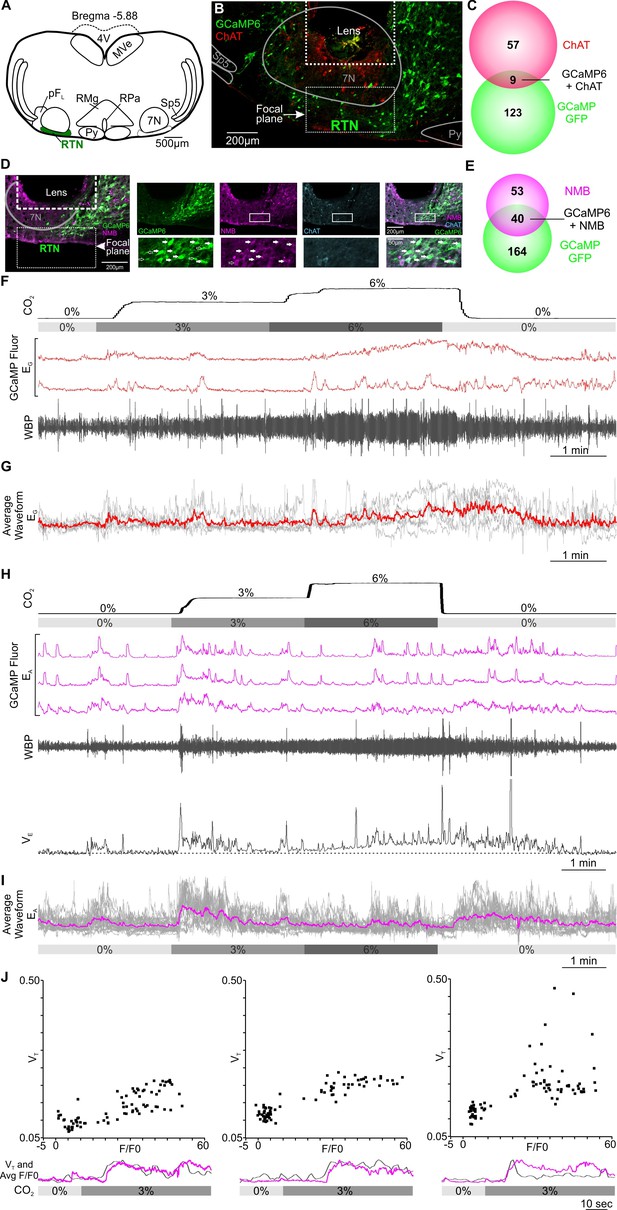
Excitatory chemosensory responses of RTN neurons in awake mice.
(A) AAV9-Syn-GCaMP6s injection into the RTN. (B) Micrograph of lens placement and viral transduction of neurons (green) relative to the facial nucleus (ChAT +neurons red). (C) Venn diagram of cell counts of GCaMP6s transduced (green), and ChAT+ (red), neurons under the GRIN lens (1 representative section from each of 4 mice). (D) Neurons transduced with GCaMP6s also contain NMB confirming their identity as RTN neurons. (E) Venn diagram of cell counts of GCaMP6s transduced (green), and NMB+ (magenta), neurons under the GRIN lens (1 representative section from each of 3 mice). (F) Recording of mouse whole body plethysmography (WBP) in response to hypercapnia time-matched with Inscopix recorded GCaMP6 signals. These show neurons that gave a graded response to CO2 (EG). (G) Average of the graded neuronal responses aligned to the WBP trace in (F). (H) Examples of neurons that showed an adapting response to a change in inspired CO2 (EA). WBP trace aligned to the GCaMP6 fluorescence and instantaneous VE (minute ventilation) shown to demonstrate how the signals in the EA neurons closely correspond to VE. (I) Average waveform of all EA neurons in the RTN aligned to 0, 3 and 6% inspired CO2 (gray bar). (J) A plot of VT versus F/F0 for the Ca2+ signal during the transition from 0 to 3% CO2 in three individual mice showing positive correlation. Underneath each VT vs F/F0 graph is a plot of change in VT (grey) and average of EA neuronal Ca2+ activity (magenta) from respective mouse during transition from baseline to 3% CO2. Note that there is a transient increase in breathing at the beginning of hypercapnia that corresponds to the activation of the EA neurons. Abbreviations: 7 N, facial motor nucleus; Py, pyramidal tract; MVe, medial vestibular nucleus; sp5, spinal trigeminal nucleus: RTN, retrotrapezoid nucleus; RMg, raphe magnus; RPa, raphe pallidus; pFL, parafacial lateral region.
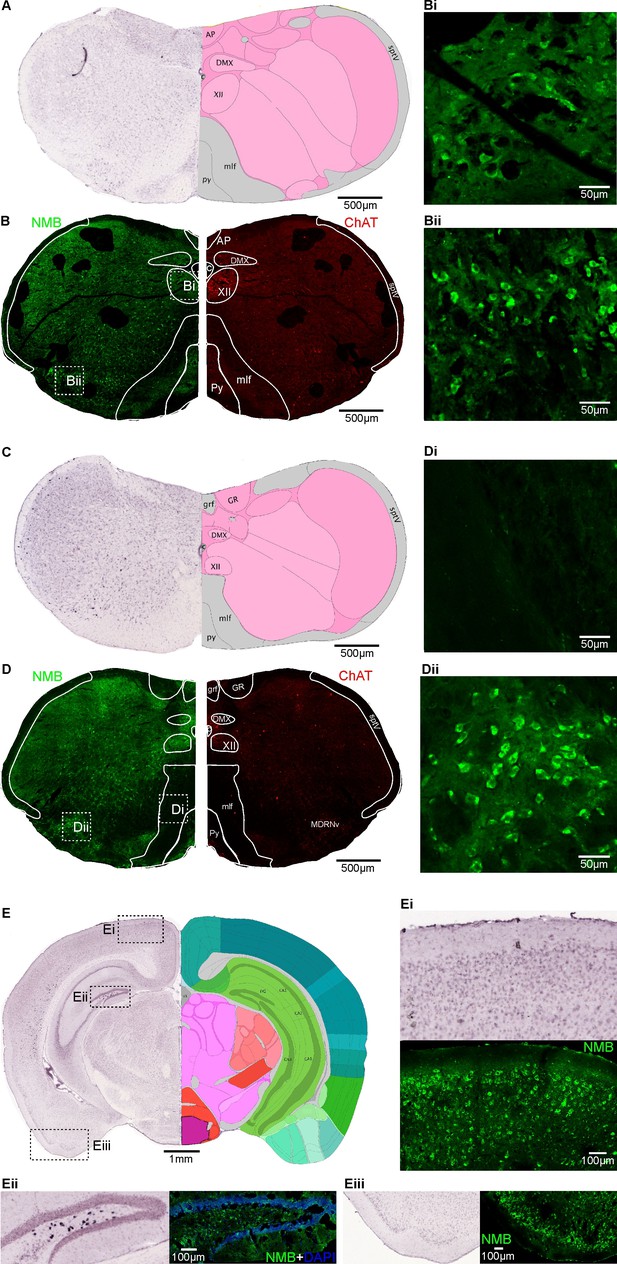
Validation of NMB immunostaining patterns.
(A) Section of caudal medulla oblongata, showing in situ hybridization (ISH) for NMB from the Allen Mouse Brain Atlas (http://mouse.brain-map.org/experiment/show/71836874) and equivalent section from the Allen Reference Atlas (atlas.brain-map.org): AP, area postrema; c, central canal; XII Hypoglossal nucleus; mlf, Medial longitudinal fascicle; DMX, Dorsal motor nucleus of the vagus nerve; sptV, Spinal tract of the trigeminal nerve. (B) NMB immunostaining of equivalent section to A and ChAT staining in the same section showing localisation of XII. Bi and Bii high-resolution images of the boxes in B showing presence of NMB immunoreactivity corresponding to the ISH above. (C) Section of caudal medulla oblongata, showing in situ hybridization (ISH) for NMB from the Allen Brain Atlas (mouse.brain-map.org/experiment/show/ 71836874) alongside the equivalent section from the Allen Reference Atlas (atlas.brain-map.org): abbreviations as in A; GR gracile nucleus; grf gracile fascicle; MDRNvp Medullary reticular nucleus ventral part. (D) NMB immunostaining showing equivalent section to the adjacent Allen Brain ISH section and ChAT in the same section showing localisation of XII. Di and Dii high-resolution images of the boxes in the image to the left showing absence of NMB immunoreactivity in the mlf, corresponding to the ISH above. Note the absence of NMB staining in the mlf, and presence of NMB staining in the MDRNvp. (E) Section of forebrain, showing in situ hybridization (ISH) for NMB from the Allen Brain Atlas (mouse.brain-map.org/experiment/show/ 71836874) alongside the equivalent section from the Allen Reference Atlas (atlas.brain-map.org). Ei, Comparison of NMB staining in cortex with ISH from the Allen Brain Atlas at a corresponding location. Note the absence of NMB staining in cortical layer 1, and sporadic staining for NMB in deeper layers, which matches the ISH pattern. Eii, NMB staining in the dentate gyrus corresponds with ISH from the Allen Brain Atlas. Eiii, NMB staining in the piriform and amygdalar cortex, note its absence in the superficial layers corresponds to the absence of ISH in the equivalent Allen Brain image.
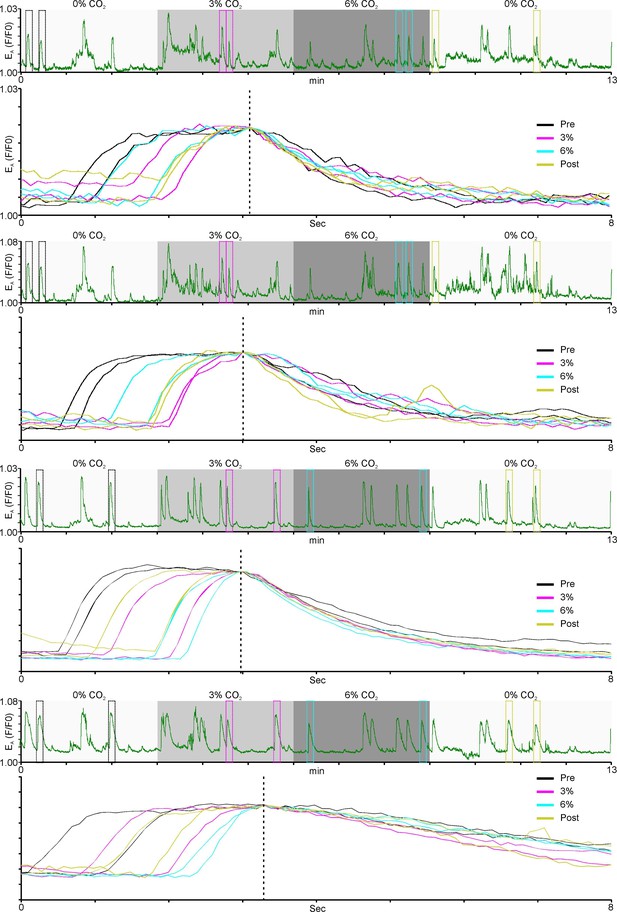
The dynamics of individual Ca2+ transients from two EA cells and two T cells recorded in the RTN before, during and after hypercapnia.
Individual transients (denoted by boxes in continuous trace) have been scaled, aligned to the decay phase and superimposed to show that the decay phase (reflecting extrusion/sequestration) remains constant and longer in duration than the rising phase (reflecting influx) during the entire experiment. Note that neither the decay phase nor the rising phase show any notable changes that could account for the adapting pattern of Ca2+ activity observed in the EA cells.
Excited graded (EG) RTN neuronal response to hypercapnia in synapsin GCaMP6 transfected mice.
The dimensions of the fluorescence video are 733 x 574 µm (width x height).
Excited adapting (EA) RTN neuronal response to hypercapnia in synapsin GCaMP6 transfected mice.
The dimensions of the fluorescence video are 691 x 524 µm (width x height).
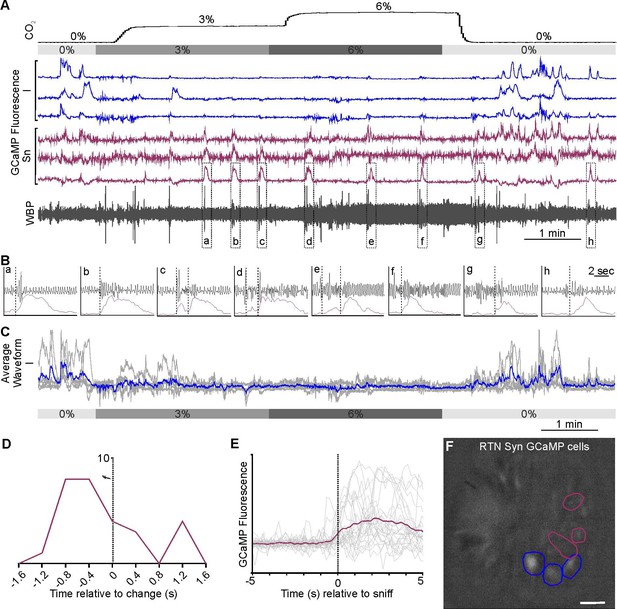
Sniff-coding, and CO2-inhibited RTN neuronal responses in awake mice.
(A) Recording of mouse WBP in response to hypercapnia time-matched with Inscopix recorded GCaMP6 signals. CO2-Inhibited (blue) and sniff-coding (eggplant purple) calcium traces correspond to the neurons shown in panel F. (B) Expanded recordings from (A), the start of the Ca2+ transient is shown by the dotted line. (C) Average waveform of RTN inhibited neurons in response to hypercapnia. (D) Sniff-correlation histogram and (E) Spike triggered average (eggplant purple line) of all Ca2+ events (grey lines) temporally correlated to the beginning of sniff activity (dotted verticle line). (F) GCaMP6s fluorescence of transduced RTN neurons in freely behaving mice. Individual regions of interest (ROIs) drawn around CO2-inhibited (blue) and sniff coactivated (eggplant purple) neurons. (Scalebar- 20 μm).
-
Figure 3—source data 1
Data for Figure 3 panels D and E.
- https://cdn.elifesciences.org/articles/70671/elife-70671-fig3-data1-v2.xlsx
RTN sniff-coding (Sn) and inhibited (I) neuronal responses in mice transduced with synapsin GCaMP6.
The dimensions of the fluorescence video are 733 x 574 µm (width x height).
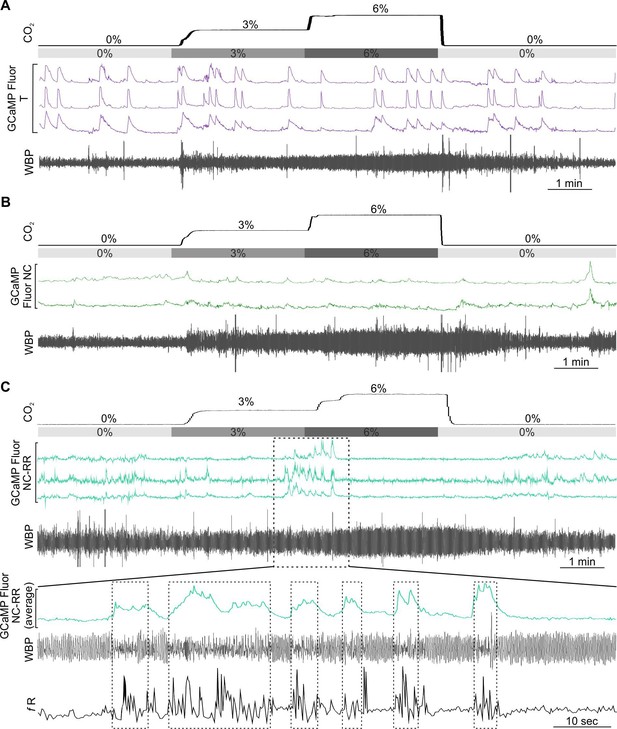
Tonically active, non-coding (NC) and NC-respiratory related (NC-RR) RTN neuronal responses in awake mice.
Recording of mouse WBP in response to hypercapnia time-matched with Inscopix recorded GCaMP6 signals (A, B, C). GCaMP fluorescence of RTN (A) tonically active (T; violet blue) (B) non-coding (NC; green) and (C) non-coding respiratory related (NC-RR; shamrock green) neurons. Average of NC-RR neuronal activity (dotted box) expanded under it displayed Ca2+ signals that were correlated to changes in respiratory frequency (fR) and were generally silent when tidal volume (WBP) was at its greatest.
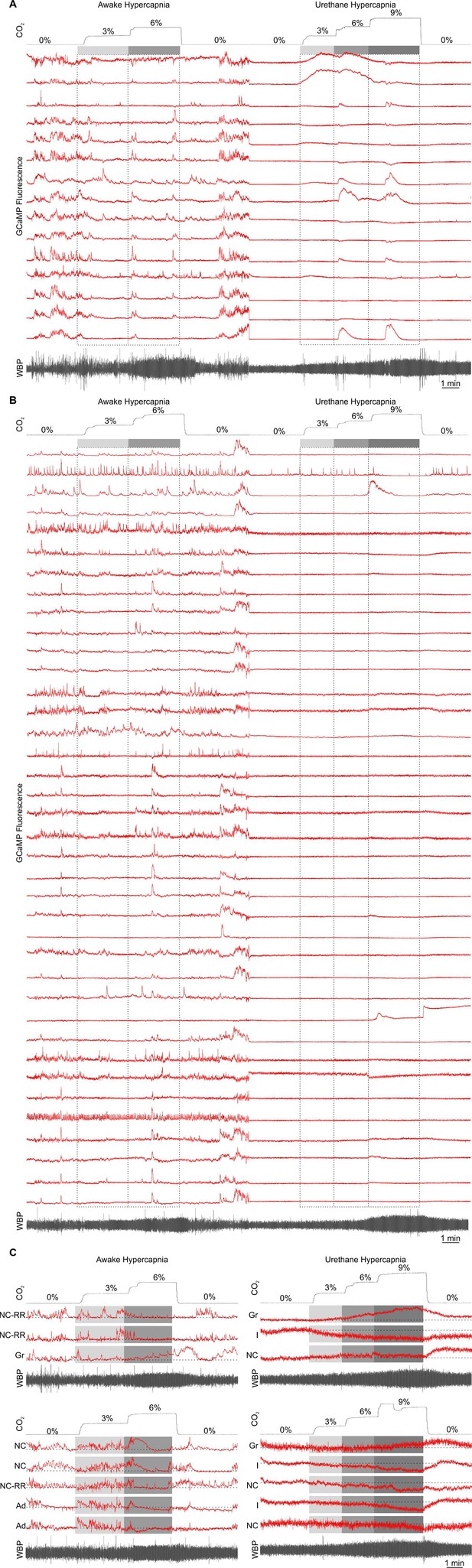
Hypercapnia induced RTN neuronal responses in awake and anaesthesized mice.
(A–B) Successive recordings from the same cells under the awake and anaesthesia states. Note how anaesthesia reduces the spontaneous activity of the neurons relative to the awake state. Under anaesthesia neurons are completely silent (A–B). (C) In two different mice (top and bottom) recorded under awake and anaesthesia at different sessions, the same cells showed completely different responses under these awake and anaesthesia states. Note how cells displaying a graded response to hypercapnia under anaesthesia were classified as non-coding respiratory related (NC-RR) or non-coding (NC) in the awake state.
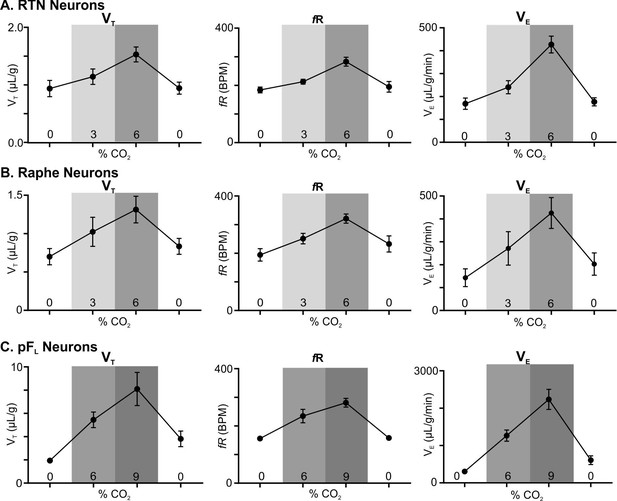
Change in breathing frequency (fR), tidal volume (VT) and minute ventilation (VE) in response hypercapnia from mice recorded in the study.
Change in tidal volume (VT; left) breathing frequency (fR, bpm; centre), and minute ventilation (VE; right) in response to changes in CO2 concentration in mice from which (A) RTN neurons, (B) Raphe neurons, (C) pFL neurons were recorded. Data presented as mean ± s.e.m (n=6 mice for RTN and Raphe; n=3 mice for pFL).
-
Figure 4—figure supplement 2—source data 1
Data for Figure 4—figure supplement 2.
- https://cdn.elifesciences.org/articles/70671/elife-70671-fig4-figsupp2-data1-v2.xlsx
Tonically active (T) RTN neurons (irrespective of hypercapnia) in synapsin GCaMP6 transfected mice.
The dimensions of the fluorescence video are 824 x 650 µm (width x height).
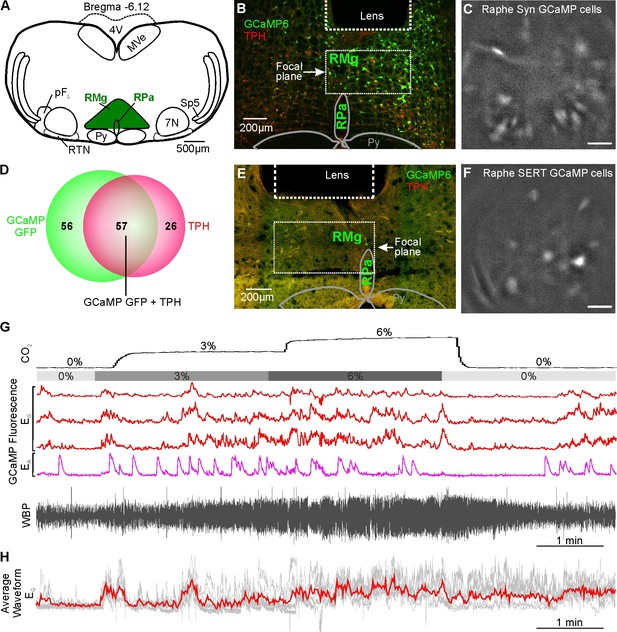
CO2-excitated medullary raphe neurons in awake mice.
(A) AAV injection into the Raphe. (B) Micrograph of lens placement and AAV9-Syn-GCaMP6s viral transduction of neurons (green) relative to the Raphe (TPH +neurons red). (C) GCaMP6s fluorescence signal from AAV9-Syn-GCaMP6s transduced raphe neurons in freely behaving mice (Scale bar- 50 μm). (D) Venn diagram of cell counts of AAV9-Syn-GCaMP6s transduced neurons (green) under the GRIN lens with TPH +neurons (red) in the raphe (1 representative section from each of 4 mice). (E) Micrograph of lens placement and AAV9-SERT-GCaMP6s viral transduction of neurons (green) relative to the Raphe (TPH +neurons red). (F) GCaMP6s fluorescence signal from AAV9-SERT-GCaMP6s transduced raphe neurons in freely behaving mice (Scale bar- 50 μm). (G) WBP recording in response to hypercapnia time-matched with Inscopix recorded GCaMP6 signals. GCaMP fluorescence of raphe excitatory graded (EG), and excitatory adapting (EA) neurons in response to hypercapnia. (H–I) Average waveform of raphe EG neuronal responses to hypercapnia aligned to the WBP trace in G. Abbreviations defined in Figure 2.
Excited graded (EG) and adapting (EA) Raphe neuronal responses to hypercapnia in synapsin GCaMP6 transfected mice.
The dimensions of the fluorescence video are 824 x 650 µm (width x height).
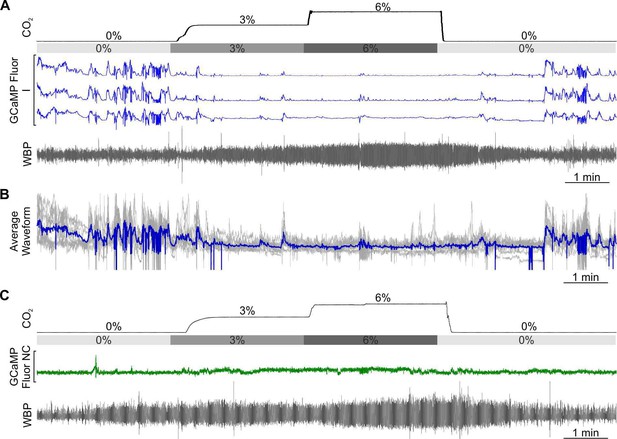
CO2-inhibited and non-coding raphe neuronal responses in awake mice.
Recording of mouse WBP in response to hypercapnia time-matched with Inscopix recorded GCaMP6 signals. GCaMP fluorescence of raphe CO2 inhibited (I; blue) (A) and non-coding (NC; green) (C) neurons. (B) Average waveform of raphe inhibited neurons in response to hypercapnia aligned to the WBP trace in A.
Inhibited Raphe neurons due to hypercapnia in SERT GCaMP6 transfected mice.
The dimensions of the fluorescence video are 696 x 650 µm (width x height).
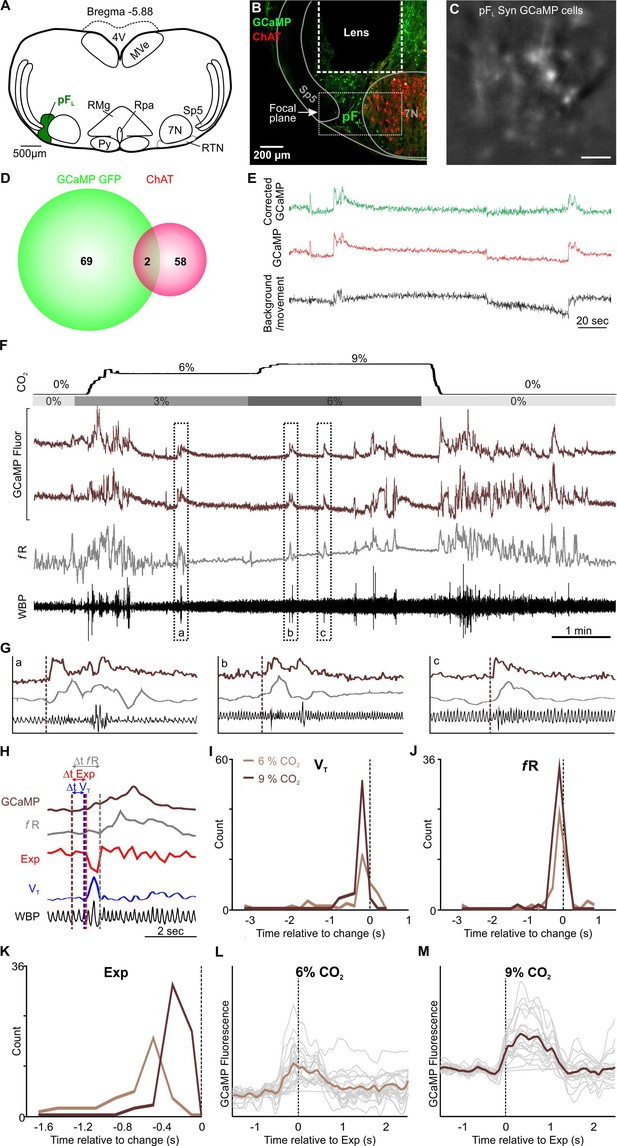
pFL neurons drive active expiration.
(A) AAV9-Syn-GCaMP6f injection into the pFL. (B) Micrograph of lens placement and viral transduction of pFL neurons (green) relative to the facial nucleus (ChAT +neurons red). (C) GCaMP6f fluorescence signal from transduced pFL neurons in freely behaving mice (Scale bar- 50 μm). (D) Venn diagram of cell counts of GCaMP6f transduced (green) and ChAT+ (red) neurons under the GRIN lens (1 representative section from each of 3 mice). (E) Movement artefact subtracted from GCaMP signal extracts a clear Ca2+ transient from pFL neurons. An ROI was placed over the border of a blood vessel (background/movement; black) and the subsequent fluoresence recording was subtracted from the GCaMP signal (uncorrected GCaMP; red) giving rise to neuronal Ca2+ transients of pFL neurons (corrected GCaMP; green) in awake mice that are free from the movement artefact. (F) WBP recording in response to hypercapnia time-matched with Inscopix recorded GCaMP signals. GCaMP6f fluorescence of transient expiratory (Exp; dark brown) pFL neurons. (G) Expanded traces from (F), the start of the Ca2+ transient is shown by the brown dotted line. (H) Measurements of GCaMP6 fluorescence (dark brown) relative to tidal volume (VT, blue), respiratory frequency (fR, grey), and expiration (Exp, red). Verticle dotted lines represent the start of the changes on their respective channels. (I–K) Frequency histograms of timing of changes in GCaMP fluorescence of pFL neurons relative to tidal volume (VT, left), respiratory frequency (fR, centre), and expiration (Exp, right). (L–M) Spike triggered average (brown line) of all Ca2+ events (grey lines) temporally correlated to the beginning of expiratory activity (dotted verticle line) at (L) 6% CO2 and (M) 9% CO2. Abbreviations defined in Figure 2.
-
Figure 7—source data 1
Data for Figure 7 panels I-K.
- https://cdn.elifesciences.org/articles/70671/elife-70671-fig7-data1-v2.xlsx
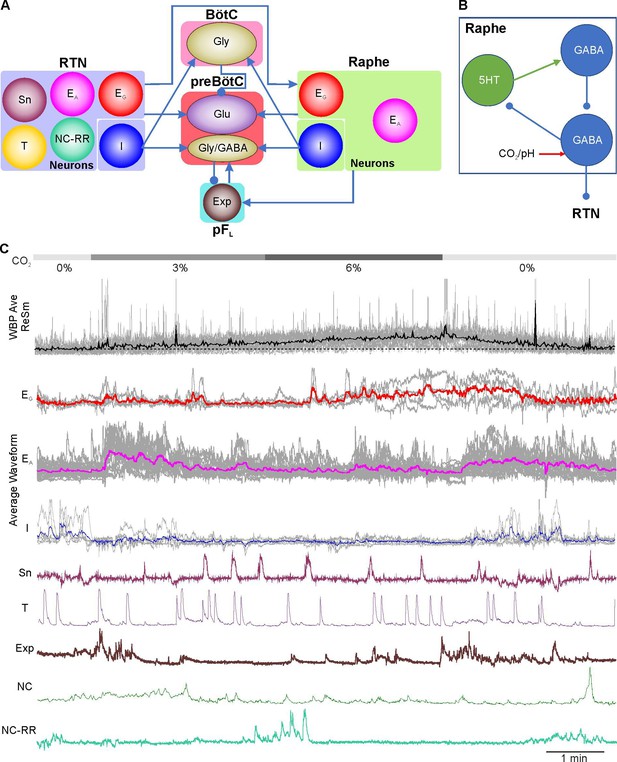
Summary diagram of findings in the paper showing contribution of the RTN, Raphe and pFL neurons to respiratory chemosensitivity and active expiration.
(A) Block diagram showing the types of activity pattern observed in neurons of the RTN, pFL and Raphe. Proposed potential interconnections to the preBötzinger and Bötzinger complexes are speculative and based on regional interactions reported in the literature. (B) Hypothesis for generating CO2 dependent inhibition of serotonergic neurons. CO2 is proposed to activate GABAergic neurons that inhibit tonically active serotonergic neurons which are not directly CO2 sensitive. If these were to activate a separate population of non-CO2 sensitive GABAergic neurons, these could normally silence the CO2 sensitive neurons until an episode of hypercapnia. This mechanism could explain an indirect inhibitory action of CO2 on both serotonergic and GABAergic neurons. (C) Averages of the different types of neuronal activity seen in the RTN, Raphe and pFL during hypercapnia aligned to an average of the WBP trace.
Tables
Reagent type (species) or resource | Designation | Source or reference | Identifiers | Additional information |
---|---|---|---|---|
Gene (Mus musculus) | Promoter of mouse Slc6a4, Gene Accession: NM_010484 | Genecopoiea | MPRM41232-PG02 | |
Strain, strain background (Mus musculus, male) | wild-type | Jackson Laboratories | C57BL/6 J background | |
Cell line (Homo sapiens) | 293AAV Cell Line | CellBioLabs | AAV-100 | HEK293-T |
Antibody | (Goat polyclonal) anti-cholineacetyl transferase antibody | Millipore | RRID:AB_262156 | (1:100 dilution) |
Antibody | (Rabbit polyclonal) anti-Tryptophan hydroxylase 2 antibody | Millipore Sigma | RRID:AB_10806898 | (1:500 dilution) |
Antibody | (Rabbit polyclonal) anti- NMB (CENTER) antibody | Sigma-Aldrich | RRID:AB_2619620 | (1:1000 dilution) |
Antibody | (donkey polyclonal) anti- rabbit IgG (H+L) Alexa Fluor 680 | Jackson Immunoresearch | RRID:AB_2340627 | (1:250 dilution) |
Antibody | (donkey polyclonal) anti- rabbit IgG (H+L) Alexa Fluor 594 | Jackson Immunoresearch | RRID:AB_2340621 | (1:250 dilution) |
Antibody | (donkey polyclonal) anti- goat IgG (H+L) Alexa Fluor 568 | Abcam | RRID:AB_2636995 | (1:250 dilution) |
Antibody | (donkey polyclonal) anti- goat IgG (H+L) Alexa Fluor 680 | Jackson Immunoresearch | RRID:AB_2340432 | (1:250 dilution) |
Recombinant DNA reagent | AAV-9: pGP-AAV-syn-GCaMP6s-WPRE.4.641 | Addgene, Watertown, MA, USA | BS1-NOSAAV9 | Dilution 1:10 |
Recombinant DNA reagent | AAV-DJ: pAAV-SERT-GCaMP6s | This paper | Raphe neuronal specific virus with calcium indicator | Dilution 1:10 |
Recombinant DNA reagent | AAV-9: pGP-AAV-syn-GCaMP6f-WPRE.24.693 | Addgene, Watertown, MA, USA | BS3-NXFAAV9 | Dilution 1:10 |
Recombinant DNA reagent | pAAV.Syn.GCaMP6s.WPRE.SV40 | Addgene, Watertown, MA, USA | Plasmid#100843 | |
Recombinant DNA reagent | AAV packaging plasmid pAAV-DJ, AAV-DJ Helper Free Expression System | Cellbiolabs | VPK-410-DJ | |
Recombinant DNA reagent | AAV packaging plasmid pHelper, AAV-DJ Helper Free Expression System | Cellbiolabs | VPK-410-DJ | |
Sequence-based reagent | VC327-4 6 s HindIII for | This paper | PCR primers | 5´- TTGACTGCCTAAGCTTgccaccatgcatcatcatcatcatcatg 300 nM |
Sequence-based reagent | VC327-4 6 s Afe rev | This paper | PCR primers | 5’-GATCTCTCGAGCAGCGCTtcacttcgctgtcatcatttgtacaaact 300 nM |
Peptide, recombinant protein | PrimeSTAR GXL DNA Polymerase | Takara Bio Europe SAS | R050A | 1 U·µg-1 |
Commercial assay, kit | In-Fusion HD Cloning Kit | Takara Bio Europe SAS | 639642 | |
Chemical compound, drug | OptiPrep | PROGEN Biotechnik GmbH | 1114542 | Iodixanol |
Commercial assay, kit | 2 x qPCRBIO SyGreen Mix Hi-ROX | PCRBiosystems | PB20.12 | |
Chemical compound, drug | kainic acid | Fisher Scientific | 15467999 | 8 mg·kg–1 IP |
Chemical compound, drug | atropine | Westward Pharmaceutical Co. | 0641-6006-10 | 120 µg·kg–1 |
Chemical compound, drug | meloxicam | Norbrook Inc. | 2 mg·kg–1 | |
Chemical compound, drug | buprenorphine | Reckitt Benckiser | 100 µg·kg-1 | |
Chemical compound, drug | PEI MAX Hydrochloride Transfection Grade Linear 40,000 mwco | Polysciences Europe GmbH | 24765–1 | 1 µg·µL-1 |
Software, algorithm | Inscopix Data Processing Software (IDPS) | Inscopix | Python Tools 2.2.6 for Visual Studio 2015 | Version: 1.6.0.3225 |
Software, algorithm | Spike2 | Cambridge Electronic Design | RRID:SCR_000903 | Version: 8.23 |
Software, algorithm | Prism 9 | GraphPad | RRID:SCR_002798 | Version 9.1.0 |
Software, algorithm | ZEN | Carl Zeiss | RRID:SCR_013672 | Version 11.0.0.190 |
Additional files
-
Supplementary file 1
A user-modifiable worksheet that uses simple mathematical functions to explore the predicted correlation between VT and F/F0 for EA neurons under two assumptions.
(i) no adapting component in the VT response to hypercapnia; and (ii) an adapting VT component in the response to hypercapnia is present. This should be compared to the data shown in Figure 2J.
- https://cdn.elifesciences.org/articles/70671/elife-70671-supp1-v2.xlsx
-
Transparent reporting form
- https://cdn.elifesciences.org/articles/70671/elife-70671-transrepform1-v2.docx