Autonomous clocks that regulate organelle biogenesis, cytoskeletal organization, and intracellular dynamics
Figures
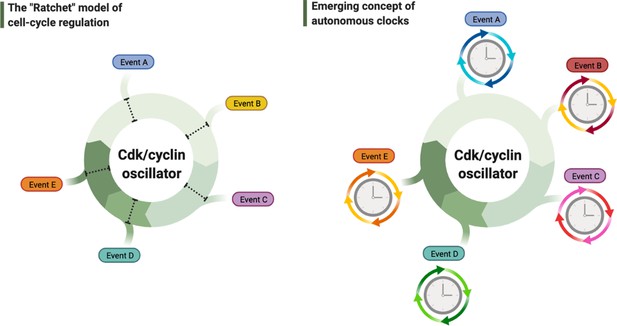
Debated models of the cell cycle.
Left diagram depicts the long-standing model of cell cycle regulation where cellular events are triggered directly by the principal cyclin-dependent kinase (Cdk)/cyclin cell division oscillator (CCO). Right diagram describes the emerging concept of autonomous clocks, where the CCO phase-locks the rhythms of otherwise intrinsic mechanisms responsible for timing different cellular events.
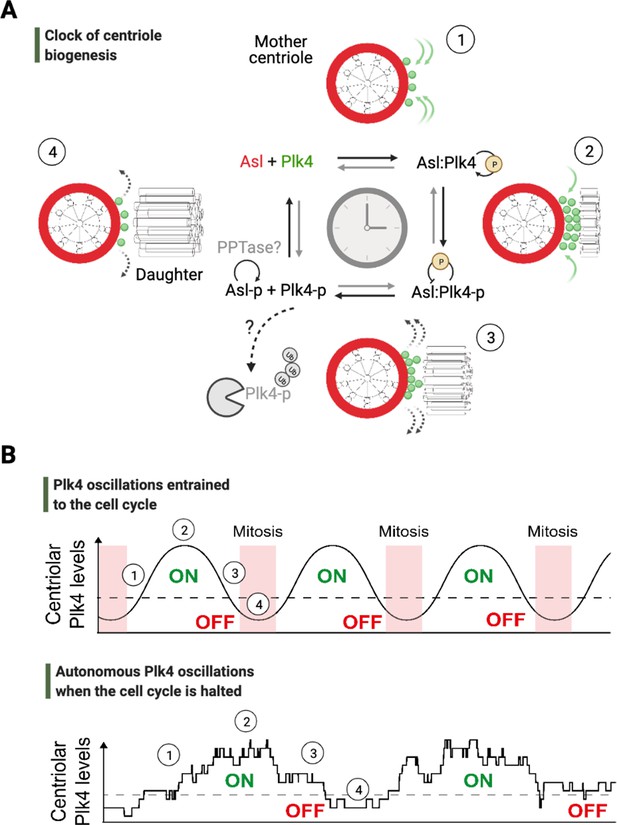
An autonomous clock of centriole biogenesis.
(A) Cartoon schematic describes proposed steps of the clock of centriole formation (Aydogan et al., 2020). Plk4 (green) is recruited to centrioles by its centriolar receptor Asl/Cep152 (red) (step 1). Plk4 begins to activate itself by its phosphorylation in trans and trigger daughter centriole formation (step 2). Next, active Plk4 may phosphorylate its receptor to inhibit the binding, leading to Plk4’s departure and/or potential degradation simultaneously to cease daughter centriole assembly (step 3). In order to reset the clock, phosphorylated receptor may be dephosphorylated by a phosphatase to start the process over (step 4). (B) Top graph illustrates Plk4 oscillations in entrainment with the cell cycle under wild-type conditions. Here, the Plk4 oscillation data on centrioles can be extracted via taking an average from the entire population of centrioles in fly embryos as the centriole duplication cycle is fully coupled to nuclear cycles. This leads to smoother curves for Plk4 oscillations. Bottom graph depicts Plk4 oscillations in cell-cycle-arrested conditions, where they continue to run and trigger centriole biogenesis autonomously. The duplications of individual centrioles are no longer coupled at the population level, so the individual centrioles duplicate periodically without any obvious synchronization. This leads to noisier Plk4 oscillation curves (as there is much less sampling of the Plk4 signal). Dotted lines indicate the threshold amount of centriolar Plk4 needed to start/stop centriole biogenesis (emphasized by the colored ON/OFF labels). Numbers on both the graphs superimpose relevant steps of the centriole clock model described in (A) onto the oscillations in (B).
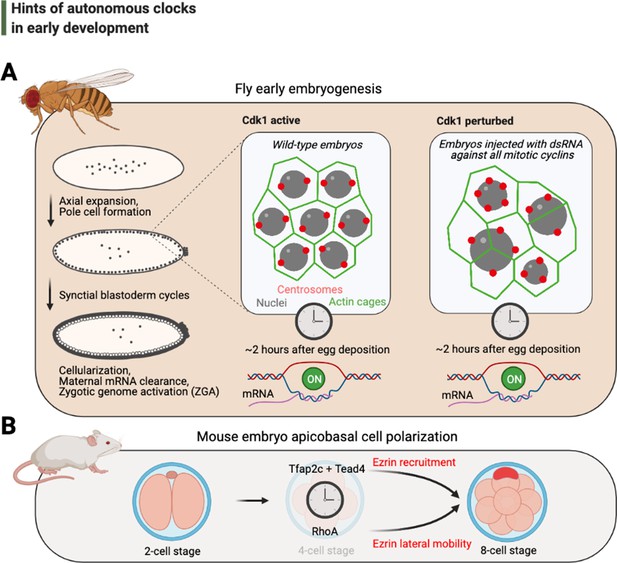
Hints of autonomous clocks in early development.
(A) Illustrations on the left describe Drosophila early embryo development within 2 hr of egg deposition. The two panels on the right describe the cytoskeletal architecture during the syncytial blastoderm cycles in embryos that develop normally (Cdk1 active) or in arrested embryos with 2–3× fewer nuclei (Cdk1 perturbed), which nevertheless cellularize and activate their genome after ~2 hr after egg deposition. (B) Cartoons depict recently identified elements of the autonomous clock that is thought to trigger, at least in part, apicobasal cell polarization during mouse embryogenesis. Cdk: cyclin-dependent kinase.
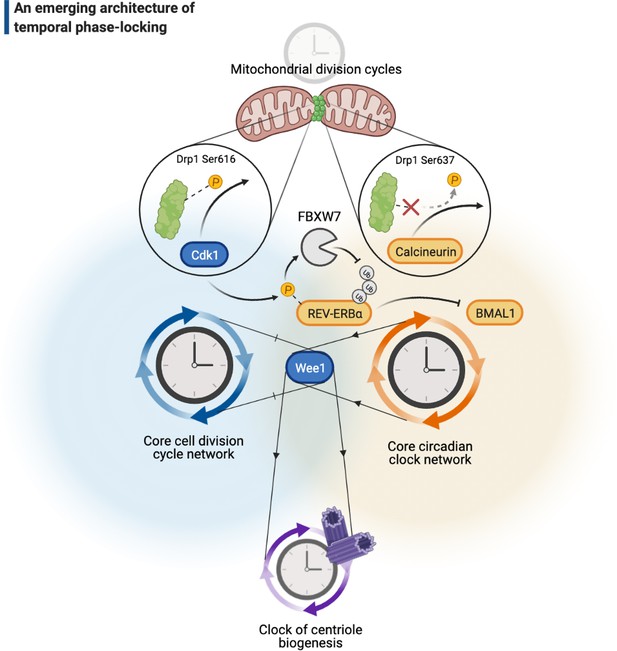
A cellular architecture of phase-locking in biological time control.
The simplistic cartoon, guided by recent advances, describes a logic for how intrinsically cyclic biological events (e.g., centriole biogenesis or mitochondrial fission-fusion cycles) can be phase-locked by core components of the cell cycle (e.g., Wee1 or Cdk1) or the circadian clock (e.g., REV-ERBα, BMAL1, or calcineurin) to run at similar paces. Cdk: cyclin-dependent kinase.
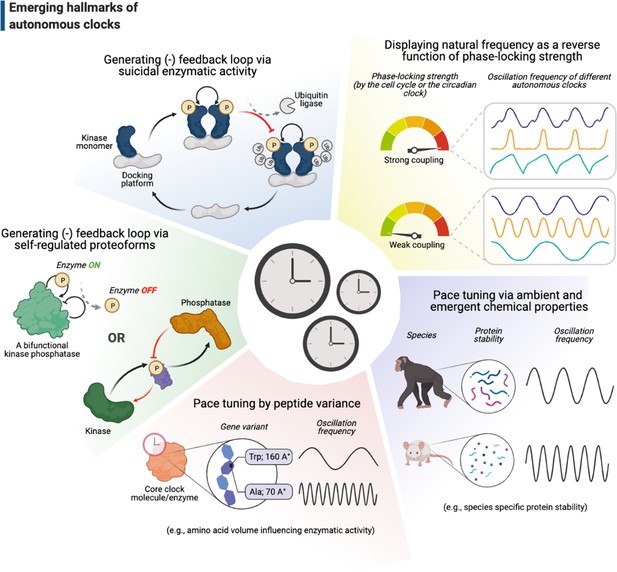
Emerging hallmarks of autonomous clocks.
Autonomous clocks may operate via engineering principles that in part display one or more of the five molecular features described in this gallery. Inspired mainly by research in the past decade of biological time control, we suggest these features as emerging hallmarks of autonomous clocks.
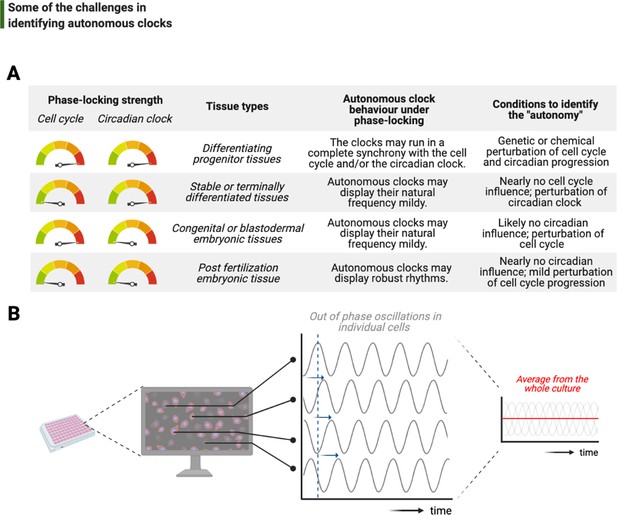
Challenges associated with the study of autonomous clocks.
(A) In most metazoans, biological events are usually phase-locked to run at the pace of the cell cycle and/or the circadian clock. The table provides examples for varying combinations of phase-locking strengths (when the strength is high, the gauge indicator points to red, or vice versa for green) and how these combinations are employed by different types of tissues during the development (first and second columns, respectively). Depending on the tissue type, autonomous clocks may display their natural frequency more easily in some (e.g., embryonic tissues) than others (e.g., proliferating progenitor tissues) (third column). We suggest experimental conditions in which novel autonomous clocks could be identified with a higher degree of confidence in interpreting their ‘autonomy’ (fourth column). (B) The flow chart illustrates a hypothetical high-throughput screen to identify novel autonomous clocks. If a prospective clock is not synchronized between cells and displays phase shift from one cell to the other (indicated by sliding blue arrows on the graph) due to the lack of an entrainment cue, then simply taking an average of rhythms in all cells may lead to a flat line. This will inevitably result in false conclusions and missed opportunities.