Structure-guided glyco-engineering of ACE2 for improved potency as soluble SARS-CoV-2 decoy receptor
Figures
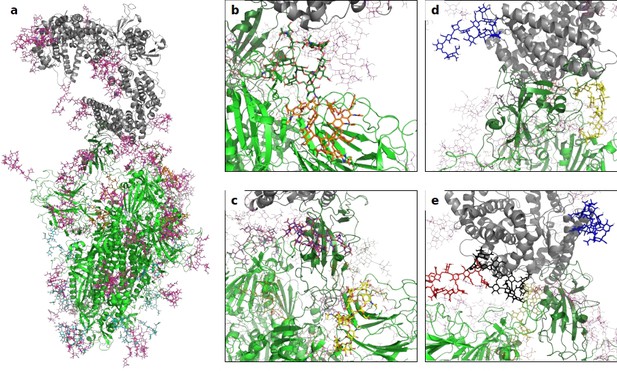
A 3D structural model of the glycosylated Spike-hACE2 complex.
(a) 3D model of the Spike trimer (in green, with RBD of monomer three in dark green) binding to ACE2 (in gray) with complex glycosylation in magenta, Man5 glycans in light blue and Man9 glycans in orange. (b) Close-up view of the glycans at N122 (orange sticks) and N165 (dark green sticks) on monomer 3 of Spike. (c) Close-up view of the glycans at N331 (yellow sticks) and N343 (purple sticks) on monomer 3 of Spike. (d) Close-up view of the glycans at N53 (blue sticks) and N90 (yellow sticks) on ACE2. (e) Close-up view of the glycans at N53 (blue sticks), N90 (yellow sticks), N322 (black sticks), and N546 (red sticks).

Site-specific glycosylation profiles of rshACE2.
Prior to analysis by LC-ESI-MS, reduced and S-alkylated rshACE2 was digested in-solution with chymotrypsin and trypsin. Glycan compositions are denoted as the sum of hexoses (H), N-acetyl-hexosamines (N), N-acetyl-neuraminic acids (i.e. sialic acids; S) and fucoses (F). Molecular ion-species detected as ammonium adducts (i.e. +17 amu) are indicated by asterisks.
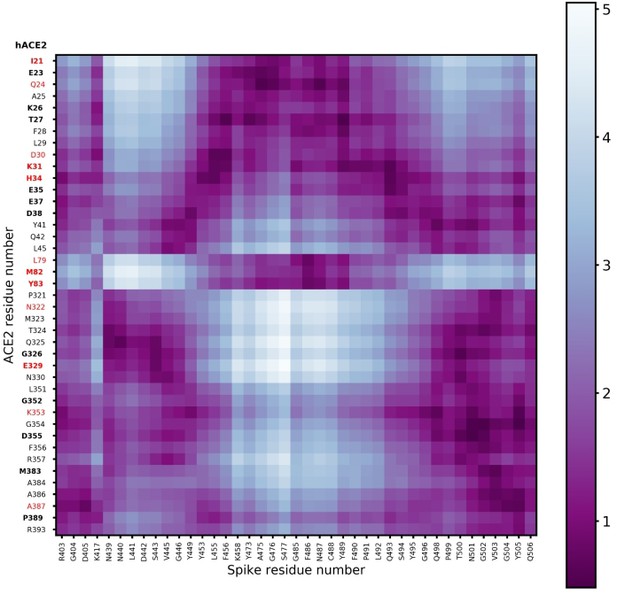
Distance heatmap of human ACE2 (hACE2) residues in contact with Spike RBD.
Black squares correspond to an average distance of 0 nm and white squares to an average distance of 6 nm. Residues which differ in mouse ACE2 are highlighted in red, residues known to be genetically polymorphic (Stawiski, E. W., Diwanji, D., Suryamohan, K., Gupta, R., Fellouse, F. A., Sathirapongsasuti, F., Liu, J., Jiang, Y., Ratan, A., Mis, M., Santhosh, D., Somasekar, S., Mohan, S., Phalke, S., Kuriakose, B., Antony, A., Junutula, J. R., Schuster, S. C., Jura, N., & Seshagiri, S. (2020). Human ACE2 receptor polymorphisms predict SARS-CoV-2 susceptibility. BioRxiv. doi:10.1101/2020.04.07.024752) are shown in bold.

Electrostatic potential of the binding interface region on ACE2.
Electrostatic potential surface of human ACE2. Red corresponds to a negative charge and blue to a positive charge. A bundle of glycan conformations is shown in sticks for the glycans at N53 (blue), N90 (yellow), N322 (black), and N546 (red).
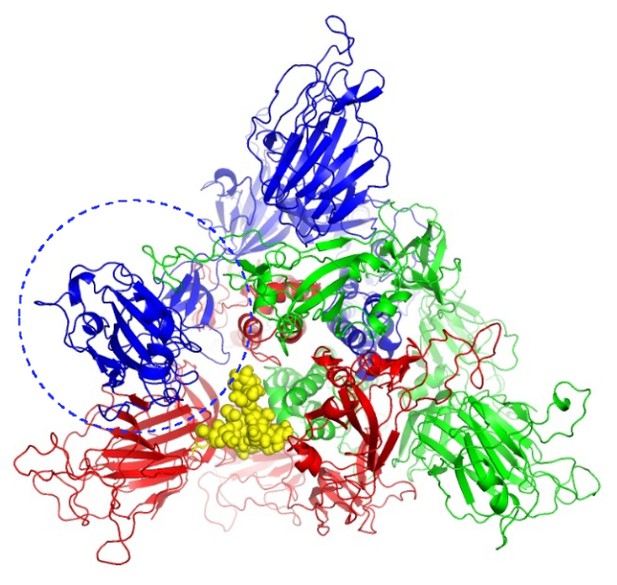
Positional modeling of glycan N234 in the Spike trimer.
The glycan at N234 (yellow spheres) of monomer 2 (red) is partially inserting into the core of the Spike trimer. The vacant space in the core is created by the receptor-binding domain of monomer 3 (blue) residing in the up conformation (in the dashed circle). Monomer 1 is shown in green.
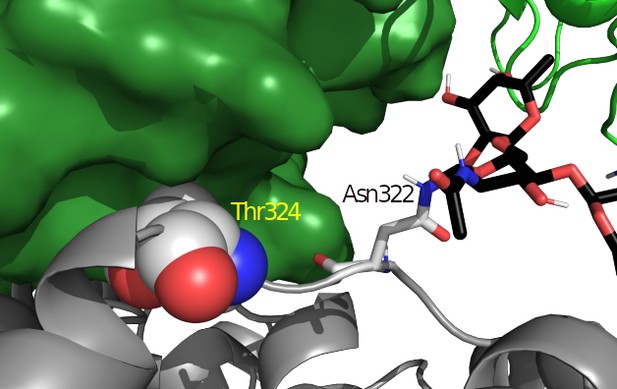
Residue T324 of ACE2 at the interface to Spike.
Residue T324 (in spheres) of ACE2 (gray) is directly interacting with the RBD of Spike (dark green surface). Ablation of the glycan (black sticks) at N322 (gray sticks) was therefore achieved through the mutation N322Q.
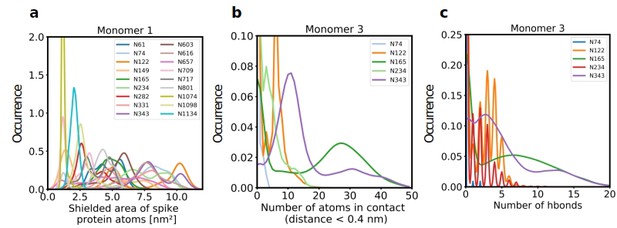
Role of Spike glycosylation in shielding the protein surface and interactions with hACE2.
(a) Normalized distribution of the area of Spike protein atoms that is shielded by each of its glycans on monomer 1 (see Figure 2—figure supplement 1 for monomers 2 and 3). (b) Normalized distribution of the number of atoms in contact with ACE2 and (c) the number of hydrogen bonds with ACE2 for glycans on monomer 3 of Spike (see Figure 2—figure supplement 2 for monomers 1 and 2).
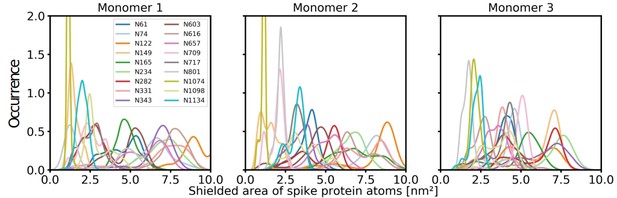
Normalized distribution of the area of Spike atoms shielded by each of its N-glycans.
The distributions were obtained from the molecular dynamics simulations for the individual monomers of Spike. For every Spike glycosite, the area of Spike protein atoms that is shielded by the glycan during the simulation is indicated. For instance, the glycan at N331 on monomer 1 shields between 7.5 and 10.0 nm2 of the Spike surface (see also Figure 1c of the main manuscript).
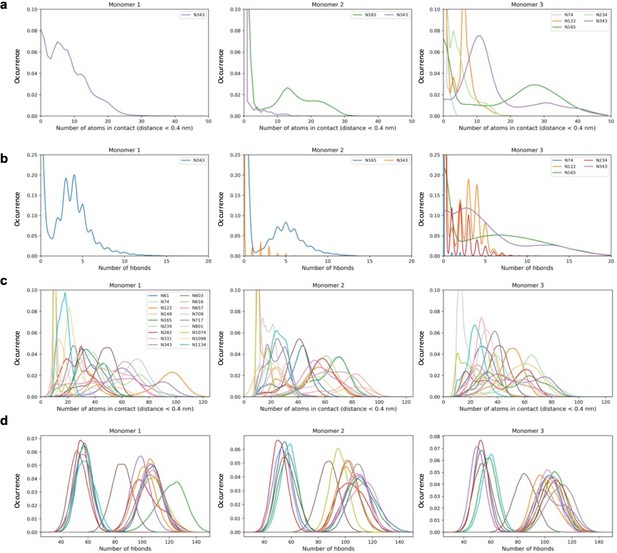
Interactions of Spike N-glycans with ACE2 and with Spike.
(a) Normalized distribution of the number of atoms of glycans of the indicated Spike monomers that are in contact with human ACE2. (b) Normalized distribution of the number of hydrogen bonds formed between Spike glycans and human ACE2, shown for 2 Spike monomers. Glycans of the second monomer did not form H-bonds with human ACE2. (c) Normalized distribution of the number of atoms of Spike glycans that are in contact with Spike protein atoms, shown for all three Spike monomers. (d) Normalized distribution of the number of hydrogen bonds formed between Spike glycans and Spike for all three Spike monomers. Monomer 3 is the interacting monomer containing the RBD. The number of interacting atoms and the number of hydrogen bonds observed during the MD simulations are represented as normalized distribution for the individual glycosites on Spike on the different monomers.
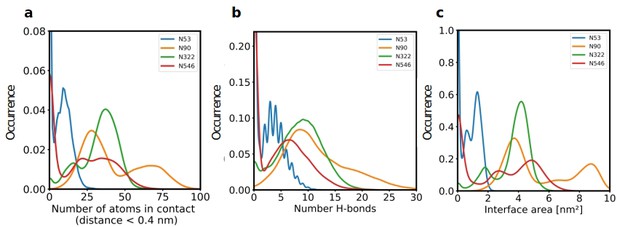
The role of hACE2 glycosylation in the interaction with Spike.
(a) Normalized distribution of the number of atoms of glycans at N53, N90, N322, and N546 of ACE2 that are in contact with Spike (distance <0.4 nm). (b) Normalized distribution of the number of hydrogen bonds between glycans at N53, N90, N322, N546 of ACE2 and Spike. (c) Normalized distribution of the interface area between Spike and glycans at N53, N90, N322, and N546 of ACE2.
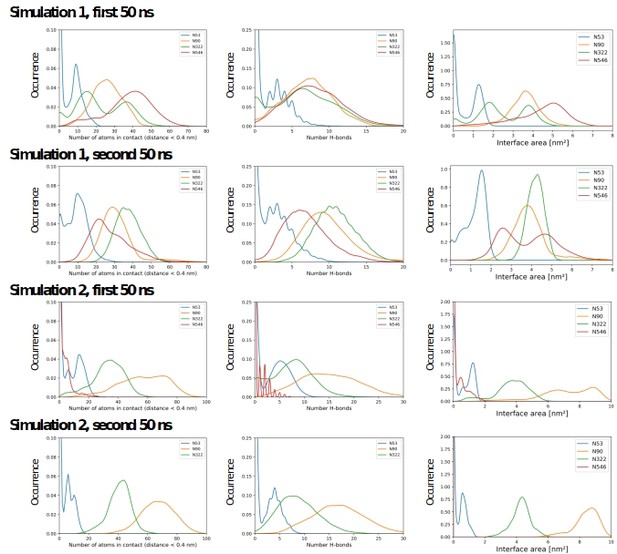
Analysis of the individual MD simulations with separate analysis of the first and second half of the simulations.
The first column shows the normalized distribution of the number of atoms of glycans at N53, N90, N322, and N546 of ACE2 that are in contact with Spike (distance <0.4 nm). The second column shows the normalized distribution of the number of hydrogen bonds between glycans at N53, N90, N322, N546 of ACE2 and Spike. The third column shows the normalized distribution of the interface area between Spike and glycans at N53, N90, N322, and N546 of ACE2.
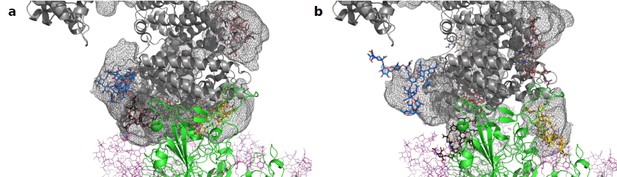
Average location density maps of glycans on ACE2.
(a) The density map (gray mesh) of the glycans at N53, N90, N322, and N546 as observed in the simulations of unbound ACE2 are superimposed onto the ACE2 – Spike complex. (b) The density map of the same glycans, as observed in the simulation of the ACE2 – Spike complex. ACE2 in gray, Spike in green. Single, randomly selected conformations of the glycans are shown in blue (N53), yellow (N90), black (N322), and red (N546).
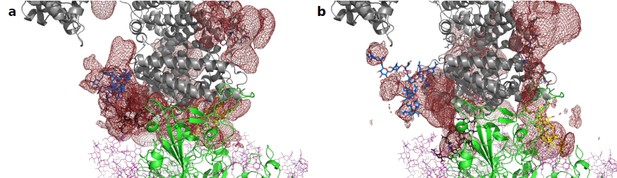
Average location density maps of sialic acids on ACE2 glycans.
(a) The density map (red mesh) of sialic acids at the termini of the glycans at N53, N90, N322, and N546 as observed in the simulations of unbound ACE2 are superposed onto the ACE2 – Spike complex. (b) The density map of the same glycans, as observed in the simulation of the ACE2 – Spike complex. ACE2 in gray, Spike in green. Single, randomly selected conformations of the glycans are shown in blue (N53), yellow (N90), black (N322), and red (N546).
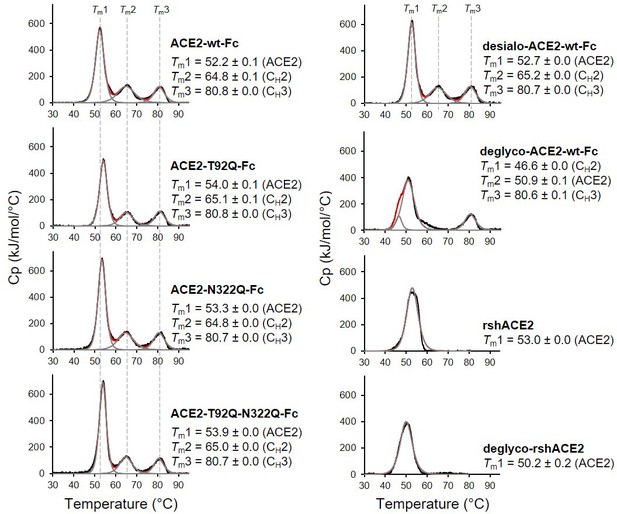
Analysis of ACE2 variants by differential scanning calorimetry (DSC).
Raw data (black) were smoothened (red) and then fitted using a non-two-state thermal unfolding model (gray). Data are presented as mean ± SEM of three independent experiments. Cp, heat capacitance; rshACE2, clinical-grade recombinant soluble human ACE2; deglyco-rshACE2, enzymatically deglycosylated rshACE2; deglyco-ACE2-wt-Fc, enzymatically deglycosylated wild-type ACE2-Fc; desialo-ACE2-wt-Fc, enzymatically desialylated wild-type ACE2-Fc.
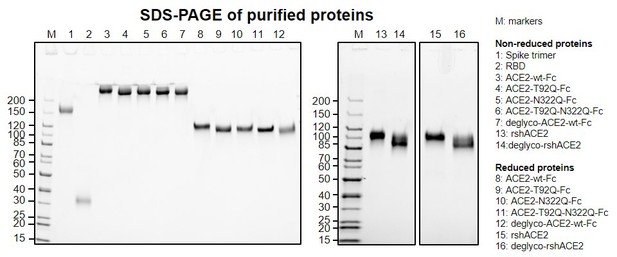
Analysis of ACE2 and Spike variants by SDS-PAGE.
Analysis of 1 µg of each purified protein by SDS-PAGE. M, molecular mass markers.
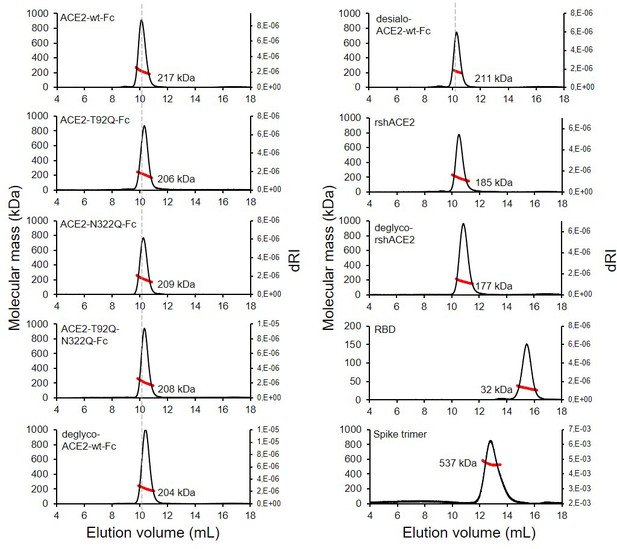
Analysis of ACE2 and Spike variants by SEC-MALS.
A total of 50 µg of Spike was loaded onto a Superose 6 Increase 10/300 GL column at a flow rate of 0.25 mL min–1. All other proteins were analyzed by injection of a total of 25 µg of the respective protein onto a Superdex 200 10/300 GL column at a flow rate of 0.75 mL min–1. The elution time of ACE2-wt-Fc is marked with a dashed line. Molecular masses (red traces) were determined by MALS. dRI, change in refractive index.
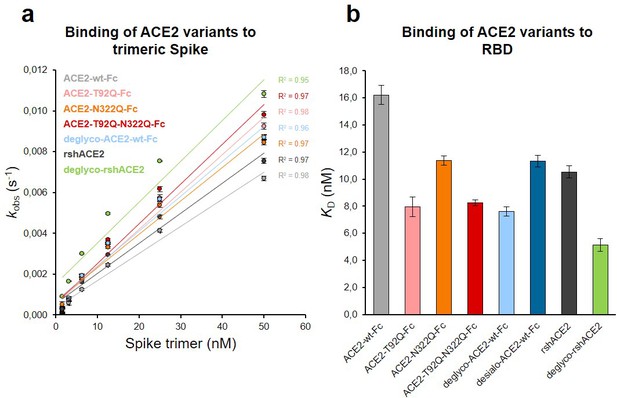
Binding of Spike and RBD to glyco-engineered ACE2 variants.
(a) Binding of Spike to glyco-engineered ACE2 variants as determined by biolayer interferometry (BLI). Plots of kobs (observed association rate) as a function of Spike concentration were generated by fitting the association data to a 1:1 binding model. Binding analysis was performed by dipping ACE2-loaded biosensors into twofold serial dilutions of purified Spike (1.6–50 nM). All measurements were performed in triplicates. rshACE2, clinical-grade recombinant soluble human ACE2; deglyco-rshACE2, enzymatically deglycosylated rshACE2; deglyco-ACE2-wt-Fc, enzymatically deglycosylated wild-type ACE2-Fc. (b) KD values for the interaction of the indicated glyco-engineered ACE2 variants with monomeric RBD. Data are presented as mean ± SEM of 3 independent experiments. Desialo-ACE2-wt-Fc, enzymatically desialylated wild-type ACE2-Fc.
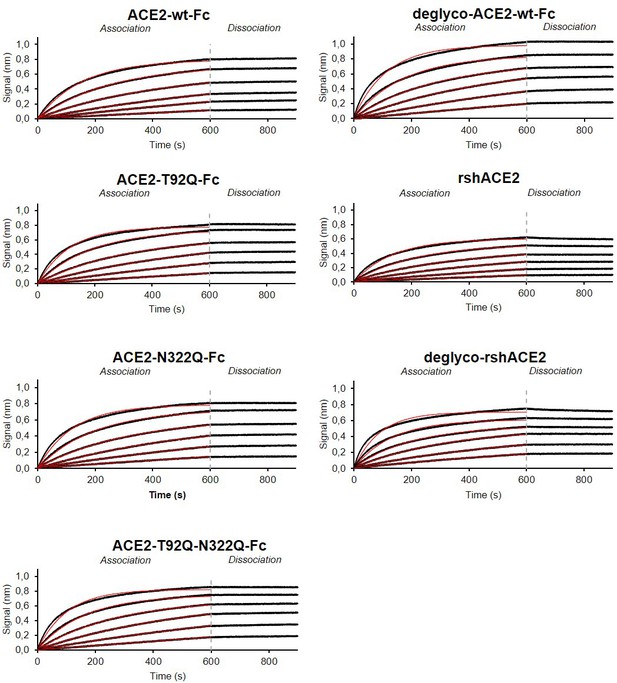
Binding of Spike to human ACE2 variants as determined by biolayer interferometry.
Binding analysis was performed by dipping biosensors loaded with different ACE2 variants into twofold serial dilutions of purified Spike (1.6–50 nM). Black lines represent the response curves of the association and dissociation phases. The data were fitted to a 1:1 binding model (red lines). For each ACE2 variant, one representative experiment out of three is shown.
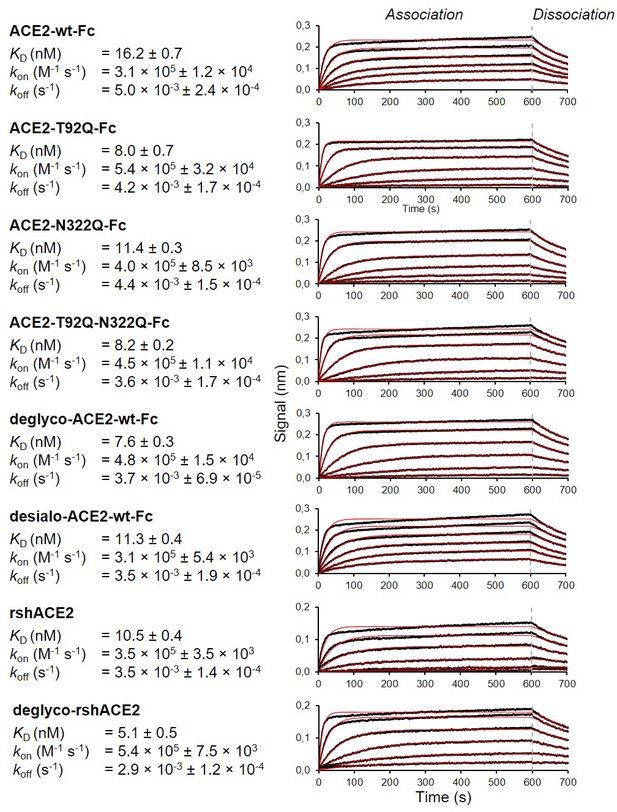
Binding kinetics of RBD to human ACE2 variants as determined by biolayer interferometry.
Black lines represent the response curves of the association and dissociation phases. The data were fitted to a 1:1 binding model (red lines). KD, kon, and koff values represent the mean ± SEM of three independent experiments. For each ACE2 variant, one representative experiment is shown.
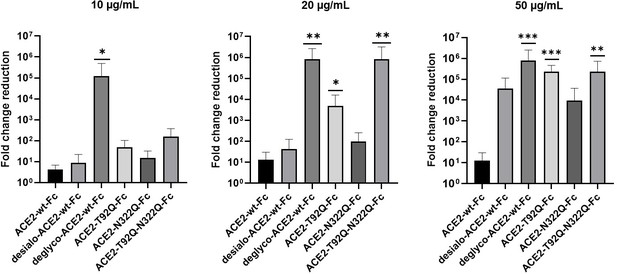
Critical role of ACE2 glycosylation for SARS-CoV-2 infectivity.
Inhibition of SARS-CoV-2 infection of Vero E6 cells using wild-type ACE2-Fc and the indicated glyco-engineered ACE2-Fc variants at final concentrations of 10–50 µg/mL. The viral RNA content of the culture supernatants was quantified by RT-qPCR and expressed as fold change reduction relative to untreated controls. With the exception of ACE2-T92Q-Fc, all data are presented as mean ± SEM of three independent experiments each performed in triplicates. In the case of ACE2-T92Q-Fc, only two independent experiments could be performed due to the limited availability of this protein. Deglyco-ACE2-wt-Fc, enzymatically deglycosylated wild-type ACE2-Fc; desialo-ACE2-wt-Fc, desialylated wild-type ACE2-Fc. *p < 0.05; **p < 0.01; ***p < 0.001 (Kruskal-Wallis).
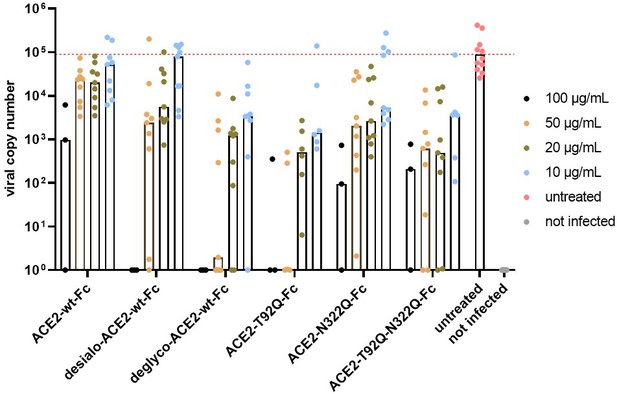
Critical role of ACE2 glycosylation for SARS-CoV-2 infectivity.
Inhibition of SARS-CoV-2 infection of Vero E6 cells (MOI: 0.002) using wild-type ACE2-Fc and the indicated glyco-engineered ACE2-Fc variants at final concentrations of 10–100 µg/mL. The viral RNA content of the culture supernatants was quantified by RT-qPCR. Data are derived from 1 to 4 experiments performed in triplicates. Deglyco-ACE2-wt-Fc, enzymatically deglycosylated wild-type ACE2-Fc; desialo-ACE2-wt-Fc, enzymatically desialylated wild-type ACE2-Fc.
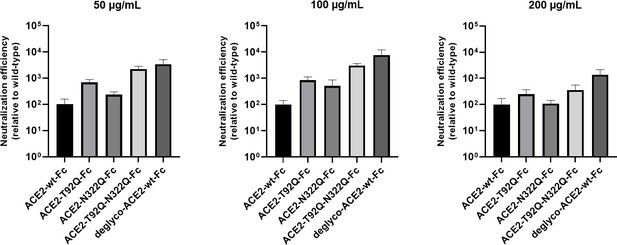
Critical role of ACE2 glycosylation for SARS-CoV-2 infectivity.
Inhibition of SARS-CoV-2 infection of Vero E6 cells (MOI: 20) using wild-type ACE2-Fc and the indicated glyco-engineered ACE2-Fc variants at final concentrations of 50–200 µg/mL. The viral RNA content of the infected cells was quantified by RT-qPCR and expressed as neutralization efficiency relative to ACE2-wt-Fc (set to 100%). Data are presented as mean ± SD of triplicates. Deglyco-ACE2-wt-Fc, enzymatically deglycosylated wild-type ACE2-Fc.
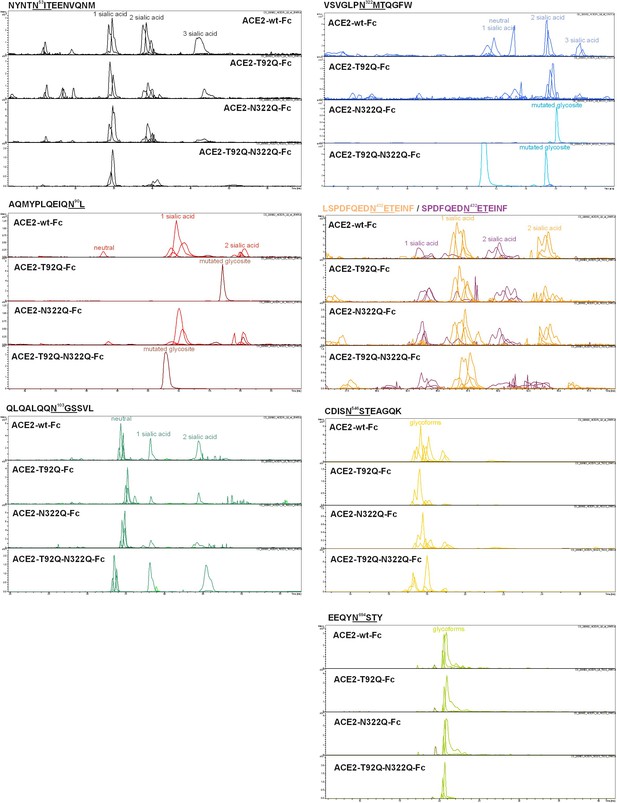
Site-specific ablation of ACE2 N-glycans by mutagenesis.
Prior to analysis by LC-ESI-MS/MS, the indicated reduced and S-alkylated ACE2-Fc variants were digested in-solution with chymotrypsin and trypsin. Comparative analysis of extracted ion chromatograms of glycopeptides confirmed the absence of glycans attached to N90 and/or N322 in ACE2-T92Q-Fc, ACE2-N322Q-Fc, and ACE2-T92Q-N322Q-Fc. The peptide sequences surrounding the different glycosites are shown.
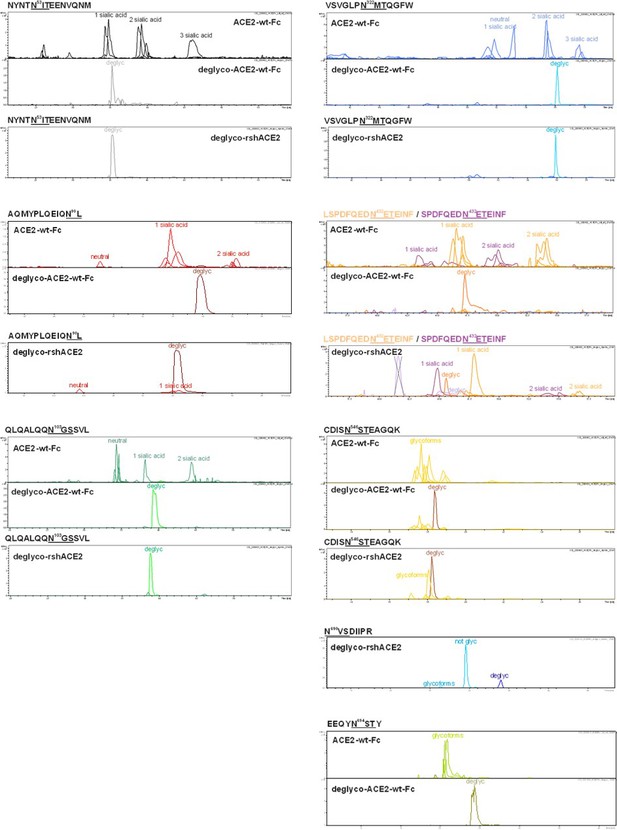
Deglycosylation of ACE2-wt-Fc and rshACE2 by PNGase F.
Prior to analysis by LC-ESI-MS/MS, native and deglycosylated (deglyco) ACE2-wt-Fc and rshACE2 were reduced, alkylated and then digested in-solution with chymotrypsin and trypsin. Comparative analysis of extracted ion chromatograms of glycopeptides confirmed the absence of N-glycans attached to N53, N90, N103, and N322 of deglyco-ACE2-wt-Fc and deglyco-rshACE2. Peptide sequences surrounding the different glycosites are shown.
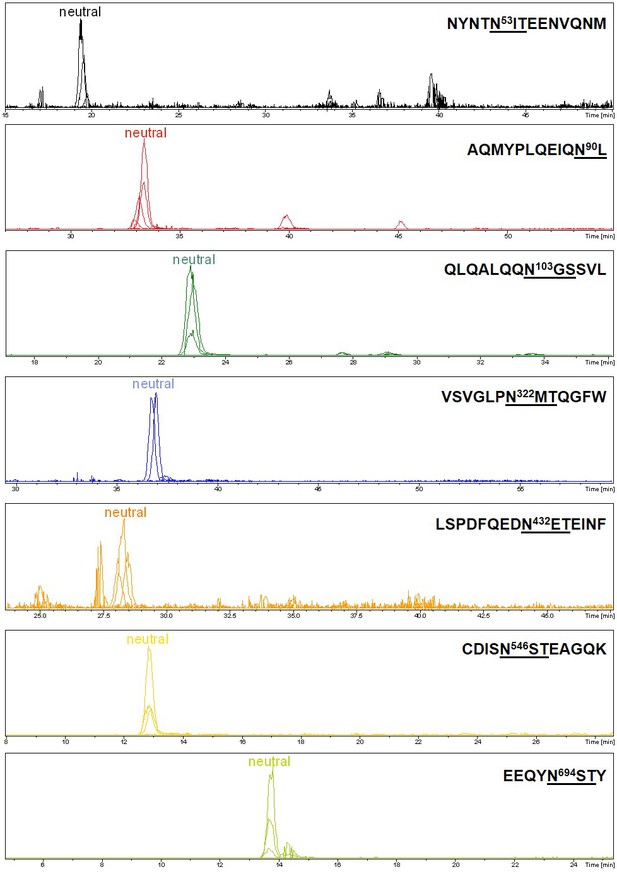
Analysis of enzymatically desialylated ACE2-wt-Fc.
The absence of sialic acids attached to N53, N90, N103, N322, N432, and N546 of desialo-ACE2-wt-Fc was confirmed by ESI-LC-MS/MS. Peptide sequences surrounding the different glycosites are shown.
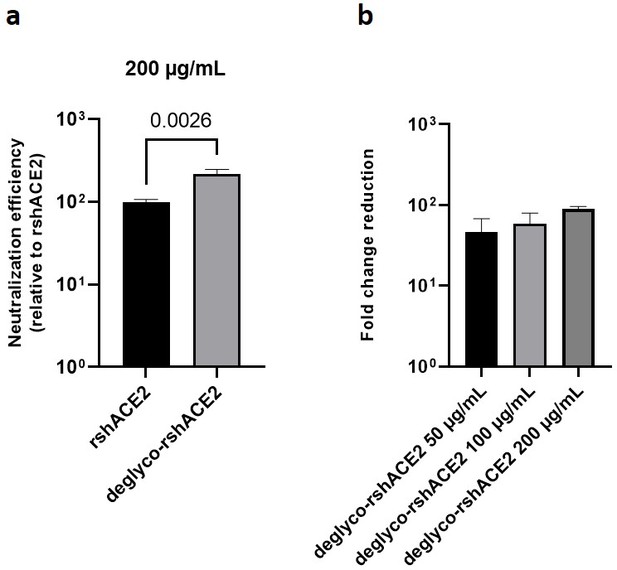
Deglycosylated rshACE2 is a potent SARS-CoV-2 decoy receptor.
(a) Inhibition of SARS-CoV-2 infection of Vero E6 cells at an MOI of 20 using native and enzymatically deglycosylated rshACE2 at final concentrations of 200 µg/mL. The viral RNA content of the infected cells was quantified by RT-qPCR and expressed as neutralization efficiency relative to native rshACE2 (set to 100%). Data are presented as mean ± SEM of four independent experiments. Deglyco-rshACE2, enzymatically deglycosylated rshACE2; p = 0.0026 (Student’s t-test). (b) Inhibition of SARS-CoV-2 infection of Vero E6 cells at an MOI of 20 using deglyco-rshACE2 at final concentrations of 50–200 µg/mL. The viral RNA content of the infected cells was quantified by RT-qPCR and expressed as fold change reduction relative to untreated controls. Data are presented as mean ± SD of triplicates.
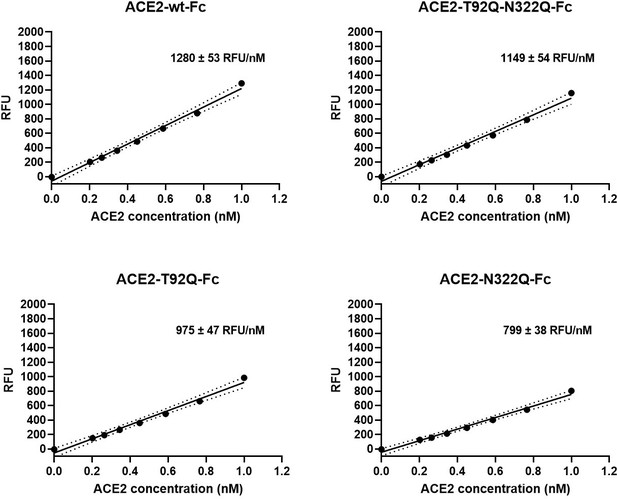
Enzymatic activity of ACE2-Fc mutants.
Hydrolysis of 100 µM 7-methoxycoumarin-4-yl-acetyl-Ala-Pro-Lys-2,4-dinitrophenyl was continuously monitored by spectrofluorimetry. Hydrolytic activity is plotted as relative fluorescence units (RFU) over ACE2-Fc concentration (in nM). All assays were performed in technical triplicates. One representative experiment out of two is shown.
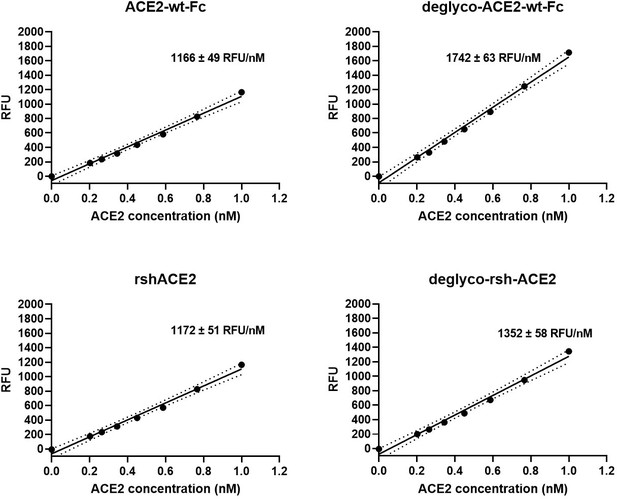
Enzymatic activity of enzymatically deglycosylated ACE2.
Hydrolysis of 100 µM 7-methoxycoumarin-4-yl-acetyl-Ala-Pro-Lys-2,4-dinitrophenyl was continuously monitored by spectrofluorimetry. Hydrolytic activity is plotted as relative fluorescence units (RFU) over ACE2 concentration (in nM). All assays were performed in technical triplicates. One representative experiment out of two is shown.
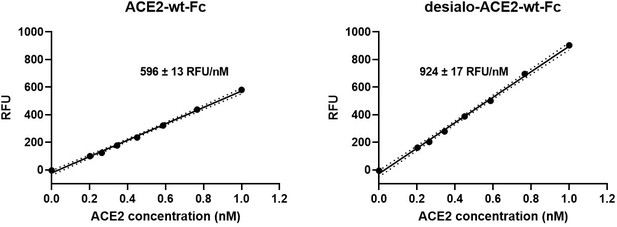
Enzymatic activity of desialo-ACE2-wt-Fc.
Hydrolysis of 100 µM 7-methoxycoumarin-4-yl-acetyl-Ala-Pro-Lys-2,4-dinitrophenyl was continuously monitored by spectrofluorimetry. Hydrolytic activity is plotted as relative fluorescence units (RFU) over ACE2 concentration (in nM). All assays were performed in triplicates. One representative experiment out of three is shown.
Videos
Molecular dynamics simulation of trimeric Spike bound to ACE2.
The movie highlights a three nano-second time segment of the molecular dynamics simulation (from 25 to 28 ns). Trimeric Spike is shown in green, RBD in dark green, and human ACE2 in gray. Complex glycosylation is shown in magenta, Man5 N-glycans in light blue and Man9 N-glycans in orange. Glycans of ACE2 at N53, N90, N322, and N546 are shown in blue, yellow, black, and red, respectively.
Tables
Reagent type (species) or resource | Designation | Source or reference | Identifiers | Additional information |
---|---|---|---|---|
Cell line (Homo sapiens) | HEK293-6E | National Research Council of Canada | RRID: CVCL_HF20 | Durocher et al., 2002 |
Cell line (Cercopithecus aethiops) | Vero E6 | ATCC | CRL-1586;RRID: CVCL_0574 | also known as VERO C1008 |
Recombinant DNA reagent | PCAGGS-RBD (plasmid) | Florian Krammer, Icahn School of Medicine at Mount Sinai | Amanat et al., 2020 | |
Recombinant DNA reagent | pCAGGS-Spike (plasmid) | Florian Krammer, Icahn School of Medicine at Mount Sinai | Amanat et al., 2020 | |
Recombinant DNA reagent | pcDNA3-sACE2(WT)-Fc(IgG1) (plasmid) | Addgene | Cat #: 145163; RRID: Addgene_145163 | Chan et al., 2020 |
Recombinant DNA reagent | pcDNA3-sACE2-T92Q-Fc(IgG1) (plasmid) | Addgene | Cat #: 145170; RRID: Addgene_145170 | Chan et al., 2020 |
Recombinant DNA reagent | pcDNA3-sACE2-N322Q-Fc(IgG1) (plasmid) | this paper | ||
Recombinant DNA reagent | pcDNA3-sACE2-T92Q-N322Q-Fc(IgG1) (plasmid) | this paper | ||
Peptide, recombinant protein | soluble human ACE2 | Apeiron Biologicals, Vienna, Austria | APN01 | Monteil et al., 2020 |
Biological sample (Severe acute respiratory syndrome coronavirus 2) | SARS-CoV-2 | Monteil et al., 2020 | GenBank MT093571 | |
Biological sample (Severe acute respiratory syndrome coronavirus 2) | SARS-CoV-2 | Charité, Berlin, Germany | Ref-SKU #: 026 V-03883 | |
Commercial assay or kit | QuikChange Lightning Site-Directed Mutagenesis kit | Agilent Technologies | Cat #: 210,518 | |
Commercial assay or kit | EZ-Link Sulfo-NHS-LC-Biotin kit | Thermo Fisher Scientific | Cat #: 21,435 | |
Commercial assay or kit | QiaAmp Viral RNA Minikit | Qiagen | Cat #: 52,904 | |
Commercial assay or kit | QuantiTect Multiplex RT-qPCR Kit | Qiagen | Cat #: 204,443 | |
Sequence-based reagent | 2019-nCoV_N1-F | This paper | qPCR primer | GACCCCAAAATCAG CGAAAT |
Sequence-based reagent | 2019-nCoV_N1-R | This paper | qPCR primer | TCTGGTTACTGCCAG TTGAATCTG |
Sequence-based reagent | 2019-nCoV_N1-P | This paper | qPCR probe | FAM-ACCCCGCATTACGTT TGGTGGACC-BHQ1 |
Other | FreeStyle F17 medium | Thermo Fisher Scientific | Cat #: A1383502 | |
Other | PNGase F | New England BioLabs | Cat #: P0705 | 180,000 U mL–1 |
Other | Neuraminidase | New England BioLabs | Cat #: P0720 | 2,500 U mL–1 |
Chemical compound, drug | Mca-Ala-Pro-Lys (Dnp)-OH | Bachem, Bubendorf, Switzerland | Cat #: 4042638 | 7-methoxycoumarin-4-yl- acetyl-Ala-Pro-Lys-2, 4-dinitrophenyl |
Software, algorithm | Gromacs | https://www.gromacs.org/ | 2019.4;RRID: SCR_014565 | |
Software, algorithm | GROMOS | http://www.gromos.net/ | 1.5.0 | |
Software, algorithm | Octet data analysis software | ForteBio | 11.1.1.39 | |
Software, algorithm | ASTRA six software | Wyatt Technology | 6 | |
Software, algorithm | Origin 7.0 for DSC software | Malvern Panalytical | 7.0 | |
Software, algorithm | GraphPad Prism 8 | GraphPad Software | 8;RRID: SCR_002798 |
Additional files
-
Supplementary file 1
Glycosylation sites and N-glycan structures used in the models of SARS-CoV-2 Spike and human ACE2 are indicated.
Blue squares indicate N-acetylglucosamine, green circles mannose, yellow circles galactose, purple squares sialic acid and green triangles fucose residues. Anomericity and positions of glycosidic linkages are indicated where necessary.
- https://cdn.elifesciences.org/articles/73641/elife-73641-supp1-v2.xlsx
-
Transparent reporting form
- https://cdn.elifesciences.org/articles/73641/elife-73641-transrepform1-v2.pdf