A coarse-grained NADH redox model enables inference of subcellular metabolic fluxes from fluorescence lifetime imaging
Figures
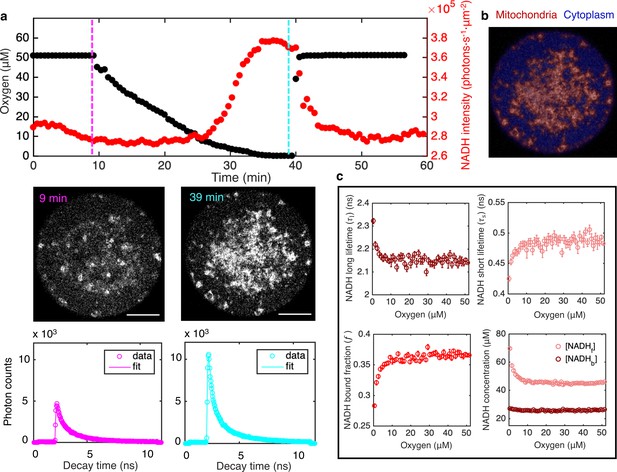
FLIM measurements of the response of mitochondrial NADH as a function of oxygen level.
(a) Top row: oxygen level (black circles) and mitochondrial NADH intensity (red circles) as a function of time. Middle row: NADH intensity images of MII mouse oocyte at high and low oxygen levels corresponding to times indicated by the vertical lines. Scale bar, 20 µm. Bottom row: NADH fluorescence decay curves of the corresponding oocyte at low and high oxygen levels, with corresponding fits. (b) NADH-intensity-based segmentation of mitochondria and cytoplasm. (c) Mitochondrial NADH long fluorescence lifetime (upper left), short fluorescence lifetime (upper right), and bound fraction (lower left) as a function of oxygen level (n=68 oocytes). These FLIM parameters can be used in combination with intensity, , and proper calibration, to obtain the concentration of free NADH, , and the concentration of enzyme-bound NADH, , in mitochondria as a function of oxygen (lower right). Error bars are standard error of the mean (s.e.m) across individual oocytes. FLIM, fluorescence lifetime imaging microscopy.

Machine learning based segmentation of mitochondria from NADH intensity images.
The NADH-based segmentation image is overlaid with MitoTracker-based segmentation image. The white region corresponds to the overlap. The accuracy of the NADH-based segmentation is quantified as the ratio of the photon count from the overlap pixels to the photon count from mitochondrial pixels based on NADH segmentation.
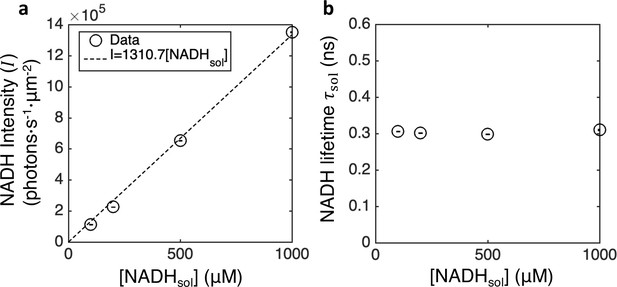
Calibration and conversion of NADH concentrations from fluorescence intensities and lifetimes in vitro.
(a) NADH intensity versus titrated NADH concentrations in AKSOM solution. (b) Fluorescence lifetime of NADH in AKSOM solution.
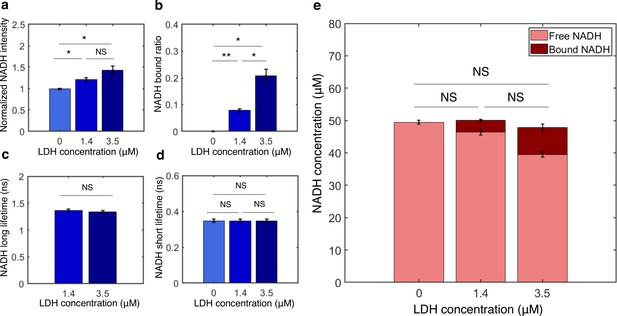
Measurement of concentrations of free and bound NADH in vitro from FLIM of NADH.
(a–d) NADH intensity, bound ratio, long lifetime, and short lifetime from FLIM of NADH with various concentrations of lactate dehydrogenase (LDH) in vitro. (e) Concentrations of free and bound NADH calculated from FLIM of NADH. Error bars are standard error across replicates. N=2. Student’s t-test is performed. *p<0.05, **p<0.01, ***p<0.001. FLIM, fluorescence lifetime imaging microscopy.
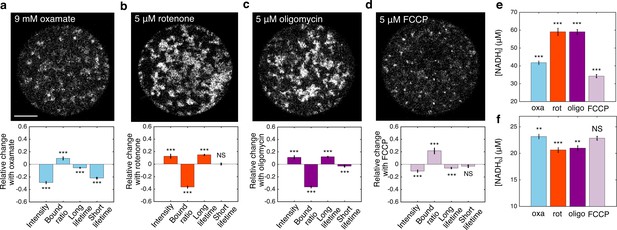
FLIM measurements of mitochondrial NADH under the impact of metabolic inhibitors.
(a–d) NADH intensity images (scale bar, 20 μm) and the corresponding changes of FLIM parameters in response to 9 mM oxamate (a) (n=28), and with an additional 5 µM rotenone (b) (n=28), 5 µM oligomycin (c) (n=37), and 5 µM FCCP (d) (n=31) perturbations. n is the number of oocytes. 15–30 min have elapsed between the administration of the drugs and the measurements. (e) Free NADH concentrations (). (f) Bound NADH concentrations (). Error bars represent standard error of the mean (s.e.m) across different oocytes. Student’s t-test is performed between parameters before and after the perturbation. *p<0.05, **p<0.01, ***p<0.001. FLIM, fluorescence lifetime imaging microscopy.
-
Figure 2—source data 1
Excel spreadsheet of single-oocyte FLIM data used for Figure 2a–f.
- https://cdn.elifesciences.org/articles/73808/elife-73808-fig2-data1-v2.xlsx
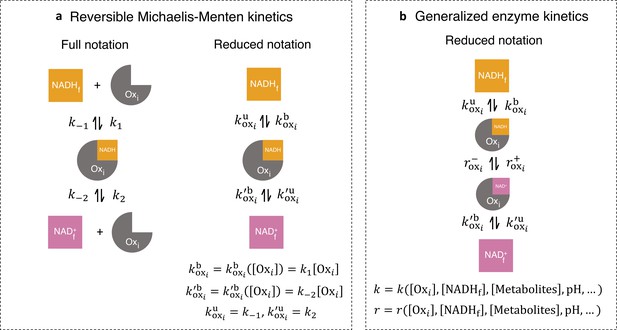
Generalized enzyme kinetics with reduced notation.
(a) (left) Full notation for reversible Michaelis-Menten kinetics. (right) A mathematically equivalent reduced notation, in which the free enzyme concentration, is incorporated into the binding rates. (b) Generalized enzyme kinetics where all kinetic rates are general functions of enzyme and metabolite concentrations and other factors.
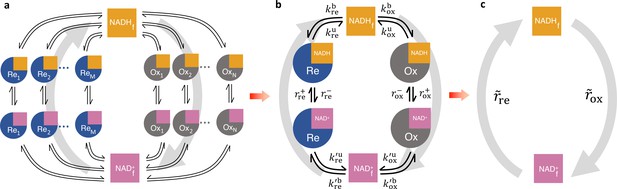
Coarse-graining the NADH redox model.
(a) Schematic of the detailed NADH redox model. We consider all possible NADH redox pathways by modeling N oxidases (Ox) and M reductases (Re). Free NADH, , and free NAD+, , can bind and unbind with each oxidase and reductase. Once bound, NADH can be oxidized reversibly to NAD+ by the oxidases, and NAD+ can be reduced reversibly to NADH by the reductases, forming a redox cycle. Gray arrows represent the total fluxes through all oxidases and reductases of the redox cycle. (b) Coarse-grained NADH redox model. All oxidases and reductases are coarse-grained into a single effective oxidase and reductase, respectively. and are the coarse-grained forward and reverse oxidation rates of the oxidase; and are the coarse-grained forward and reverse reduction rates of the reductase. and are the coarse-grained binding and unbinding rates of NADH and NAD+, respectively, to the oxidase and reductase. (c) At steady-state, all the kinetics of the model can be further coarse-grained into the turnover rate of free NADH, , and the turnover rate of free NAD+, , characterizing the two branches of the cycle.

The detailed NADH redox model.
The model consists of redox loops with N oxidases and M reductases. Each of the oxidase and reductase follows the generalized enzyme kinetics. The binding and unbinding rate of NADH to the i th oxidase (reductase) is and ( and ). The binding and unbinding rate of NAD+ to the ith oxidase (reductase) is and ( and ). Once bound, the forward and reverse reaction rates are and for the ith oxidase; and for the ith reductase. All rates can be arbitrary functions of metabolite concentrations, enzyme concentrations, and other factors (such as pH and mitochondrial membrane potential).
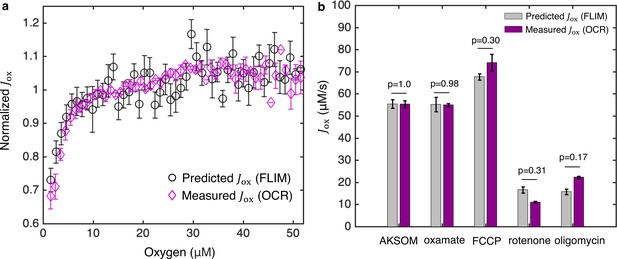
Coarse-grained NADH redox model enables accurate prediction of flux through the ETC from FLIM measurements of NADH.
(a) Predicted flux through the ETC, , from the FLIM of NADH (n=68 oocytes) agrees quantitatively with from oxygen consumption rate (OCR) measurements (N=3 measurements) for all oxygen concentrations. is normalized by its value at 50 μM oxygen. (b) Predicted from FLIM and measured from OCR for AKSOM (n=68, N=4) and with perturbations of 9 mM oxamate (n=20, N=2), 5 μM FCCP (n=31, N=2), 5 μM rotenone (n=28, N=2) and 5 μM oligomycin (n=37, N=3). Predicted agrees with measured in all cases. n denotes number of oocytes for single-oocyte FLIM measurements. N denotes number of replicates for batch oocytes OCR measurements. Each batch contains 10–15 oocytes. p values are calculated from two-sided two-sample t-test. Error bars denote standard error of the mean across individual oocytes for FLIM measurements and across batches of oocytes for OCR measurements. ETC, electron transport chain; FLIM, fluorescence lifetime imaging microscopy; OCR, oxygen consumption rate.
-
Figure 5—source data 1
Excel spreadsheet of single-oocyte FLIM data and batch OCR data used for Figure 5b.
- https://cdn.elifesciences.org/articles/73808/elife-73808-fig5-data1-v2.xlsx
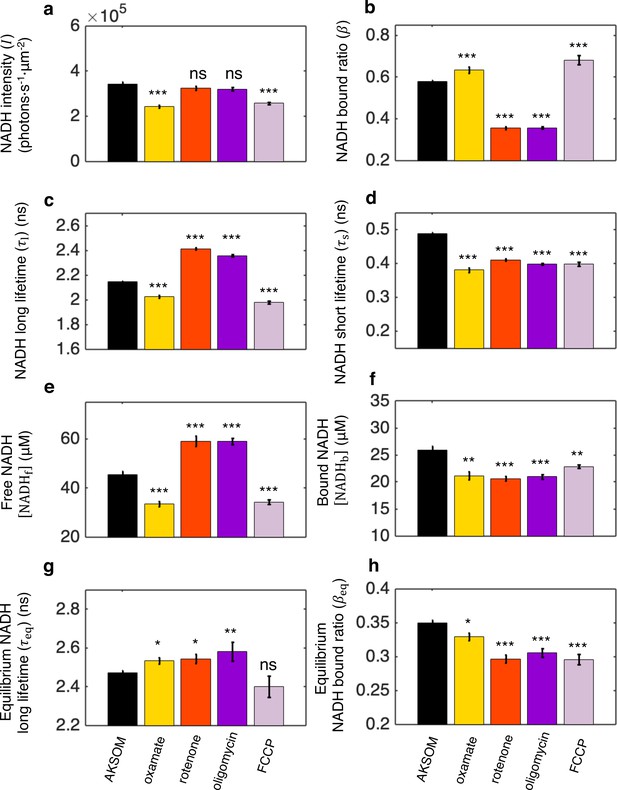
FLIM measurements of NADH in mitochondria under different biochemical perturbations.
(a) NADH intensity. (b–d) NADH bound ratio and NADH long and short fluorescence lifetimes obtained from fitting FLIM decay curves using the two-exponential decay model (Materials and methods). (e, f) Free and bound NADH concentrations obtained by using Equations S2 and S3, and the calibration from Equation S4. (g, h) Equilibrium NADH long lifetime and bound ratio, measured at the lowest oxygen level under different conditions. 9 mM sodium oxamate was present in all conditions except for AKSOM to suppress the cytoplasmic signal for better mitochondrial segmentation. AKSOM (n=68), 9 mM oxamate (n=20), 5 μM rotenone (n=28), 5 μM oligomycin (n=37), and 5 μM FCCP (n=31). n is the number of oocytes. Error bars represent standard error of the mean (s.e.m).Student’s t-test is performed pairwise between perturbations and AKSOM condition. *p<0.05, **p<0.01,***p<0.001. FLIM, fluorescence lifetime imaging microscopy.
-
Figure 5—figure supplement 1—source data 1
Excel spreadsheet of single-oocyte FLIM data used for Figure 5—figure supplement 1a-h.
- https://cdn.elifesciences.org/articles/73808/elife-73808-fig5-figsupp1-data1-v2.xlsx
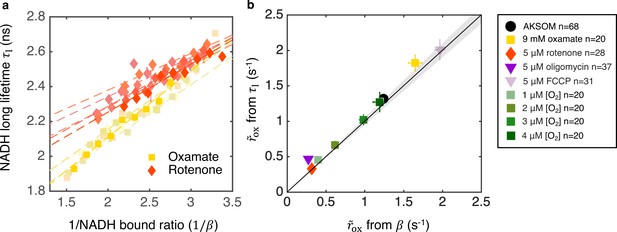
Coarse-grained NADH redox model self-consistently predicts NADH turnover rate from bound ratio and long fluorescence lifetime.
(a) NADH long lifetime, , is linearly related to the inverse of NADH bound ratio, , from the oxygen drop experiment of individual oocytes treated with oxamate and rotenone (results from five representative oocytes are shown for each condition). Each shade corresponds to results from an individual oocyte (symbols are experimental measurements and dashed lines are linear fits). (b) NADH turnover rate obtained from NADH long lifetime () using Equation 8 agrees quantitatively with that from NADH bound ratio (), obtained from Equation 5b, across all perturbations (p=0.73). The solid line denotes where from lifetime equals that from bound ratio, the gray region denotes ±5% variation from equality. Error bars represent standard error of the mean (s.e.m) across different oocytes. p value is calculated from Student’s t-test.
-
Figure 6—source data 1
Excel spreadsheet of single-oocyte FLIM data used for Figure 6b.
- https://cdn.elifesciences.org/articles/73808/elife-73808-fig6-data1-v2.xlsx

NADH long fluorescence lifetime is linearly related to the inverse of the NADH bound ratio .
(a–e) versus during oxygen drop for all drug perturbations and the corresponding linear fitting. The fitting is performed for each oocyte independently. The plot shows the average fitting across all oocytes. (f, g) Slope (A) and offset (B) obtained by fitting Equation (S50) to versus . Student’s t-test is performed pairwise between perturbations and AKSOM condition. Error bars represent standard error of the mean (s.e.m). *p<0.05, **p<0.01, ***p<0.001.
-
Figure 6—figure supplement 1—source data 1
Excel spreadsheet of single-oocyte FLIM data used for Figure 6—figure supplement 1f,g.
- https://cdn.elifesciences.org/articles/73808/elife-73808-fig6-figsupp1-data1-v2.xlsx
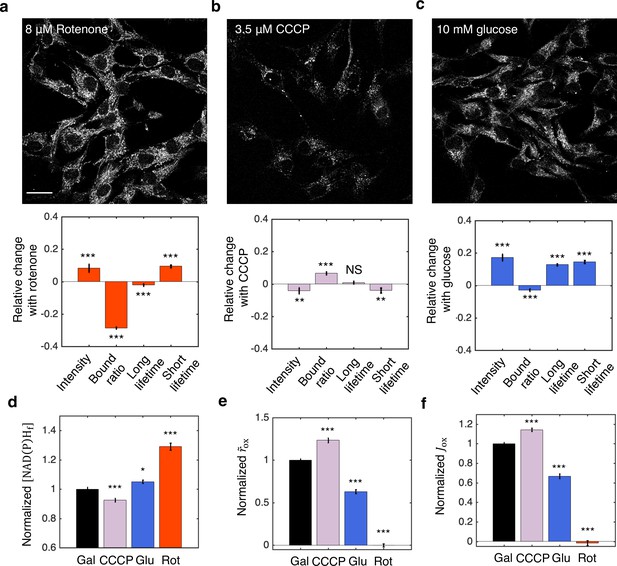
NADH redox model accurately predicts ETC flux in hTERT-RPE1 human tissue culture cells.
(a–c) NAD(P)H intensity images (scale bar, 30 μm) and the corresponding changes of FLIM parameters in response to metabolic perturbations with the addition of 8 μM rotenone (a) (N=61), 3.5 μM CCCP (b) (N=72), and the change of nutrients from 10 mM galactose to 10 mM glucose (c) (N=77). Rotenone and CCCP are added to culturing media with 10 mM galactose (N=145). Measurements were taken within 30 min after the addition of the drugs. N specifies the number of images analyzed for each condition. A typical image contains dozens of cells as shown in (a–c). (d–f) Free NAD(P)H concentrations () (d), NAD(P)H turnover rate () (e), and inferred ETC flux () (f) in response to CCCP, rotenone, and glucose perturbations. Student’s t-test is performed pairwise between perturbations and the 10 mM galactose condition. *p<0.05, **p<0.01, ***p<0.001. Error bars represent standard error of the mean (s.e.m) across different images. ETC, electron transport chain; FLIM, fluorescence lifetime imaging microscopy.
-
Figure 7—source data 1
Excel spreadsheet of single image FLIM data used for Figure 7a–f.
- https://cdn.elifesciences.org/articles/73808/elife-73808-fig7-data1-v2.xlsx

NAD(P)H FLIM parameters and TMRM measurements in response to mitochondrial inhibitors and nutrient perturbations for hTERT-RPE1 cells.
-
Figure 7—figure supplement 1—source data 1
Excel spreadsheet of single image TMRM data used for Figure 7—figure supplement 1.
- https://cdn.elifesciences.org/articles/73808/elife-73808-fig7-figsupp1-data1-v2.xlsx
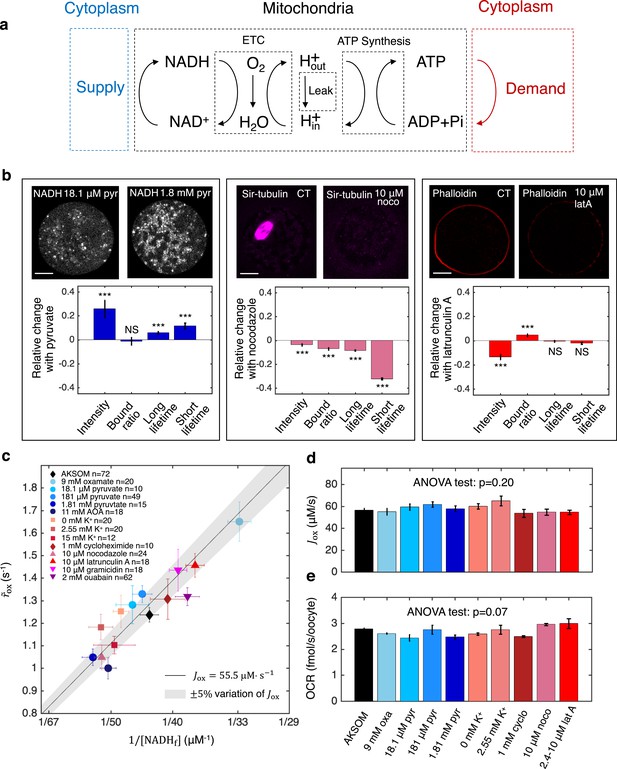
Homeostasis of ETC flux in mouse oocytes: perturbations of nutrient supply and energy demand impact NADH metabolic state but do not impact ETC flux.
(a) The three coupled cycles of mitochondrial-based energy metabolism: the NADH/NAD+ redox cycle, the proton pumping/dissipation cycle, and the ATP/ADP production/consumption cycle. Nutrients supplied from the cytoplasm (blue) power the reduction of NAD+ to NADH. Energy-demanding cellular processes in the cytoplasm (red) hydrolyze ATP to ADP. (b) Oocyte images (top) and change in NADH FLIM parameters relative to control (bottom) for changing pyruvate concentration (left), addition of 10 μM nocodazole (center) and addition of 10 μM latrunculin A (right). Student’s t-test were performed for the change of FLIM parameters (*p<0.05, **p<0.01, ***p<0.001). The spindle disassembles after addition of 10 μM nocodazole (top, center) and the actin cortex disassembles after addition of 10 μM latrunculin A (top, right). (c) NADH turnover rate () and NADH free concentrations () inferred from FLIM measurements under a variety of perturbations of nutrient supply and energy demand. Error bars are standard error of the mean (s.e.m) across oocytes. The black line corresponds to and values with an inferred flux of , and the gray shaded region corresponds to a variation of ±5% around that value. (d) The inferred ETC flux and (e) measured OCR show no change across different perturbations of nutrient supply and energy demand (ANOVA, p=0.20 and p=0.07, respectively). ETC, electron transport chain; FLIM, fluorescence lifetime imaging microscopy; OCR, oxygen consumption rate.
-
Figure 8—source data 1
Excel spreadsheet of single-oocyte FLIM data and batch OCR data used for Figure 8c–e.
- https://cdn.elifesciences.org/articles/73808/elife-73808-fig8-data1-v2.xlsx
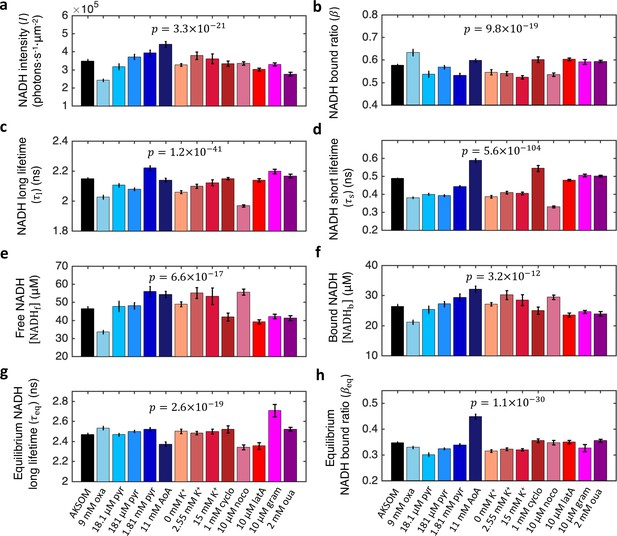
NADH FLIM parameters for mouse oocytes under all nutrient supply and energy demand perturbations.
All FLIM parameters display significant changes in response to all nutrient supply and energy demand perturbations. p value results from the ANOVA test. Number of oocytes: n=72, 20, 10, 49, 15, 18, 20, 20, 12, 10, 24, 18, 18, and 62 in corresponding order from AKSOM to ouabain. Error bars represent standard error of the mean (s.e.m) across different oocytes. FLIM, fluorescence lifetime imaging microscopy.
-
Figure 8—figure supplement 1—source data 1
Excel spreadsheet of single-oocyte FLIM data used for Figure 8—figure supplement 1.
- https://cdn.elifesciences.org/articles/73808/elife-73808-fig8-figsupp1-data1-v2.xlsx
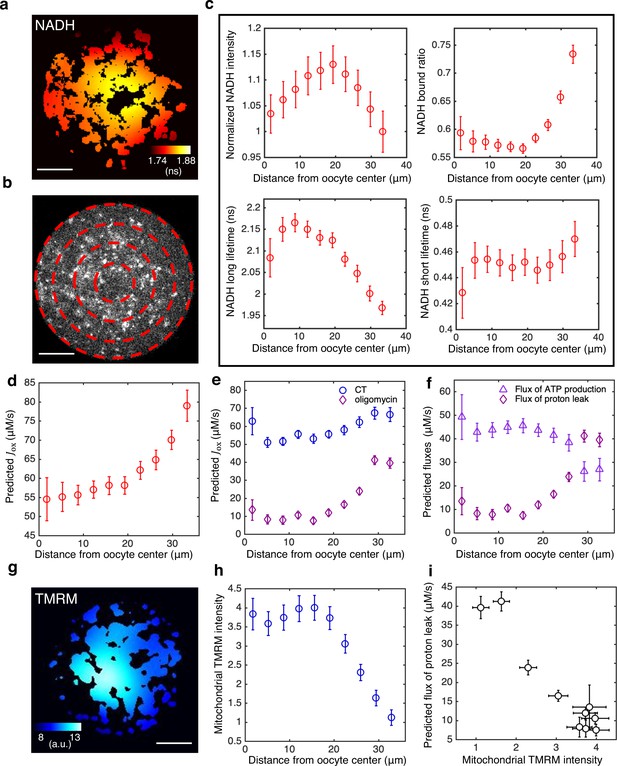
Subcellular mitochondrial heterogeneity in mouse oocytes: spatially inhomogeneous mitochondrial proton leak leads to a higher ETC flux in mitochondria closer to cell periphery.
(a) Heatmap of the mean NADH fluorescence decay time in mitochondria exhibits a subcellular spatial gradient within oocytes. (b) NADH intensity image of the oocyte partitioned with equally spaced concentric rings. (c) Mitochondrial normalized NADH intensity (upper left), bound ratio (upper right), long fluorescence lifetime (lower left), and short fluorescence lifetime (lower right) as a function of distance from the oocyte center (n=67). (d) Predicted ETC flux from FLIM of NADH as a function of distance from the oocyte center (n=67). (e) ETC flux gradient is enhanced by 5 oligomycin (n=37), suggesting the flux gradient is determined by proton leak. CT is AKSOM with oxamate (n=32). 9 mM oxamate is present in oligomycin condition to reduce cytoplasmic NADH signal for better mitochondrial segmentation. (f) Opposing flux gradients of proton leak and ATP production, where proton leak (ATP production) is maximal (minimal) at the cell periphery. (g) Heatmap of the TMRM intensity in mitochondria, which increases with mitochondrial membrane potential, exhibits a subcellular spatial gradient within oocytes. (h) Mitochondrial TMRM intensity as a function of distance from the oocyte center (n=16). (i) Predicted flux of proton leak correlates negatively with mitochondrial membrane potential as measured by mitochondrial TMRM intensity. Scale bar, 20 μm. Error bars represent standard error of the mean (s.e.m) across different oocytes. ETC, electron transport chain; FLIM, fluorescence lifetime imaging microscopy.
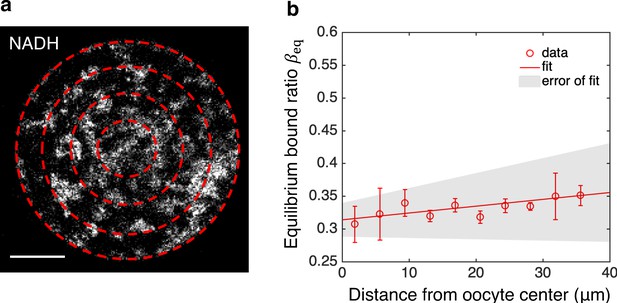
is uniform within the oocyte.
(a) NADH intensity image with oocyte partitioned by equal-distanced concentric rings. (b) Equilibrium bound ratio as a function of distance from the oocyte’s center obtained by completely inhibiting ETC with rotenone (n=10). The error bar denotes the SEM across individual oocyte. The line is from a linear fit of the data. The shaded region represents the error of fit from SEM of the slope and offset across 10 oocytes. ETC, electron transport chain.
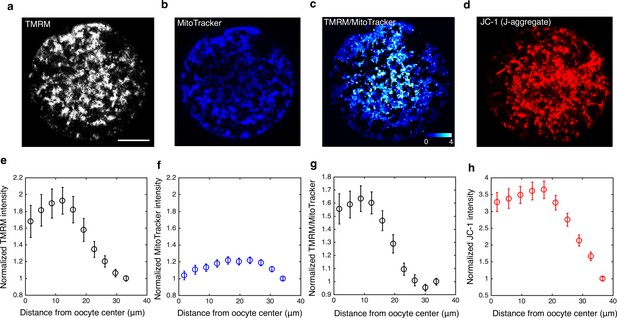
Subcellular spatial gradient of mitochondrial membrane potential.
(a–d) Intensity images of TMRM, MitoTracker Red FM, TMRM/MitoTracker ratio, and JC-1 (J-aggregate). (e–h) Normalized subcellular intensity gradient of the corresponding dyes as a function of distance from the oocyte’s center (n=18 for each dye). The intensities are normalized by the intensity of the dye closest to the cell periphery.
Videos
NADH intensity in mouse oocyte as a function of oxygen level.
Left: imaging of NADH from autofluorescence of mouse oocyte. Right: real time measurement of oxygen level in the imaging chamber.
Tables
Reagent type (species) or resource | Designation | Source or reference | Identifiers | Additional information |
---|---|---|---|---|
Cell line (Homo sapiens) | hTERT-RPE1 | Iain Cheeseman Lab | ATCC Cat# CRL-4000, RRID:CVCL_4388 | |
Biological sample (mouse) | MII oocytes | EmbryoTech | Strain: B6C3F1 | |
Commercial assay or kit | MitoTracker Red FM | Thermo Fisher Scientific | Cat.#: M22425 | |
Commercial assay or kit | TMRM | Sigma-Aldrich | Cat.#: T5428CAS: 115532-50-8 | |
Commercial assay or kit | JC-1 | Thermo Fisher Scientific | Cat.#: T3168 | |
Commercial assay or kit | SiR-Tubulin | Cytoskeleton Inc | Cat.#: CY-SC006 | |
Commercial assay or kit | Phalloidin | Thermo Fisher Scientific | Cat.#: F432 | |
Chemical compound, drug | Sodium oxamate | Sigma-Aldrich | Cat.#: O2751CAS: 565-73-1 | |
Chemical compound, drug | Rotenone | Sigma-Aldrich | Cat.#: R8875CAS: 83-79-4 | |
Chemical compound, drug | Oligomycin A | Sigma-Aldrich | Cat.#: 75351CAS: 579-13-5 | |
Chemical compound, drug | FCCP | Sigma-Aldrich | Cat.#: C2920CAS: 370-86-5 | |
Chemical compound, drug | CCCP | Sigma-Aldrich | Cat.#: C2759CAS: 555-60-2 | |
Chemical compound, drug | Glucose | Sigma-Aldrich | Cat.#: D9434CAS: 50-99-7 | |
Chemical compound, drug | Galactose | Millipore | Cat.#: 48260CAS: 59-23-4 | |
Chemical compound, drug | Pyruvate | Sigma-Aldrich | Cat.#: P2256CAS: 113-24-6 | |
Chemical compound, drug | Cycloheximide | Sigma-Aldrich | Cat.#: C4859CAS: 66-81-9 | |
Chemical compound, drug | Nocodazole | Sigma-Aldrich | Cat.#: M1404CAS: 31430-18-9 | |
Chemical compound, drug | Latrunculin A | Sigma-Aldrich | Cat.#: L5163CAS: 76343-93-6 | |
Chemical compound, drug | Gramicidin | Sigma-Aldrich | Cat.#: 50845CAS: 11029-61-1 | |
Chemical compound, drug | Ouabain | Sigma-Aldrich | Cat.#: O3125CAS: 11018-89-6 | |
Chemical compound, drug | Aminooxyacetic acid (AOA) | Sigma-Aldrich | Cat.#: C13408CAS: 2921-14-4 | |
Software, algorithm | FLIM data acquisition (SPCM) | Becker & Hickl | RRID:SCR_018310 | |
Software, algorithm | FLIM data acquisition (Labview) | National Instruments | RRID:SCR_014325 | |
Software, algorithm | FLIM data analysis (MATLAB R2015b) | MathWorks | RRID:SCR_001622 | |
Software, algorithm | OCR data acquisition(SensorTrace Profiling) | Unisense |
Connection of the NADH redox model to detailed models of complex I.
is the proton motive force. is the free energy difference of the reaction at complex I. is the standard free energy difference of the reaction at complex I. , , and are the concentrations of the oxidized, reduced, and total ubiquinone concentrations.
Model | Flux | |||
---|---|---|---|---|
Beard, 2005 | ||||
Chang et al., 2011; Jin and Bethke, 2002 | N/A | N/A | ||
Hill, 1977 | N/A | N/A | ||
Korzeniewski and Zoladz, 2001 | N/A | N/A | ||