Self-organized canals enable long-range directed material transport in bacterial communities
Figures
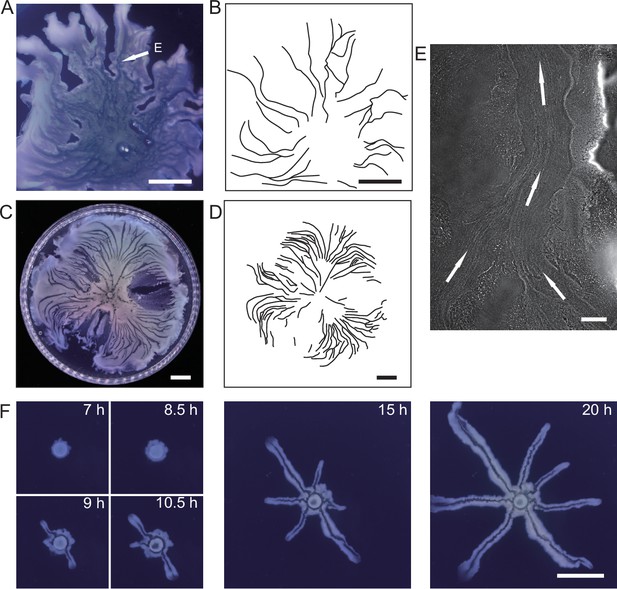
Large-scale open channels in non-flagellated P. aeruginosa colonies support directed fluid transport.
(A) Colony morphology of non-motile P. aeruginosa (PA14 flgK::Tn5 ΔpilA). The arrow indicates the position where the image in panel (E) was taken. (B) Sketch of the open channels in panel (A) for better visualization. (C) Colony morphology of piliated P. aeruginosa (PA14 flgK::Tn5). (D) Sketch of the open channels in panel (C) for better visualization. Scale bars in A–D, 1 cm. (E) Phase-contrast microscopy image of an open channel with cellular flows in a non-motile P. aeruginosa (PA14 flgK::Tn5 ΔpilA) colony, taken at the location indicated by the arrow in panel (A). Arrows indicate flow direction. Scale bar, 100 μm. Cellular flows in the open channel are better visualized in Figure 1—video 1 because the contrast between the open channel and other regions is low in still images. (F) Time-lapse image sequence showing the development of open channels in branching colonies of piliated P. aeruginosa (PA14 flgK::Tn5). Scale bar, 1 cm. Also see Figure 1—video 2.
Cellular flow in an open channel of non-motile P. aeruginosa (PA14 flgK::Tn5 ΔpilA) colony.
This real-time video is associated with Figure 1E.
Development of open channels in branching colonies of piliated P. aeruginosa (PA14 flgK::Tn5).
The colony was grown on M9DCAA agar plates at 30°C. Time label shows the elapsed time from inoculation (hh:mm). The video is associated with Figure 1F.
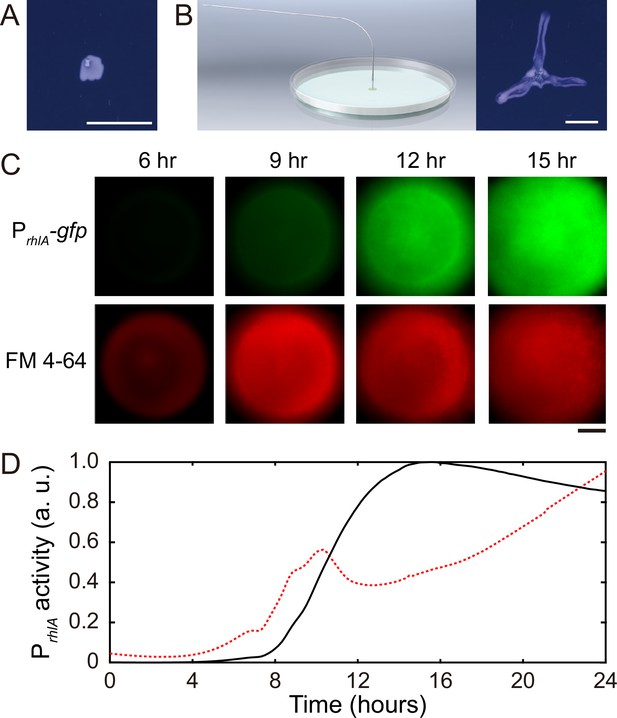
Role of rhamnolipids in canal development.
(A) Image of a representative colony of the rhamnolipid-deficient mutant (PA14 flgK::Tn5 ΔrhlA) after 20 hr growth. The colony failed to develop canals and microscopic flows. (B) Surface tension gradient established by 1 hr injection of exogenous rhamnolipids at the colony center following 20 hr growth (schematics shown as left panel; ‘Methods’) restored the formation of canals (right panel). Scale bars in (A, B), 1 cm. (C) Fluorescence image sequences showing promoter activity of rhamnolipid synthesis at the early stage of canal development. The images were taken at the center of representative canal-forming P. aeruginosa colonies (PA14 flgK::Tn5) grown at room temperature. The upper row shows GFP(ASV) fluorescence from the rhamnolipid synthesis reporter PrhlA-gfp(ASV) (‘Methods’), and the lower row shows red fluorescence of the membrane dye FM 4-64, which serves as a proxy of cell number in the colony. Scale bar, 1 mm. (D) Temporal dynamics of rhamnolipid synthesis level measured by the fluorescence of PrhlA-gfp(ASV) reporter during canal development. Solid and dashed lines represent the overall fluorescence count of the rhamnolipid synthesis reporter and the FM 4-64 dye, respectively (‘Methods’). The colony started to expand at T = ~10 hr, and the expansion caused a slight drop of overall FM 4-64 fluorescence count during T = ~10–12 hr. Three replicate experiments were performed, and they showed the same temporal dynamics.
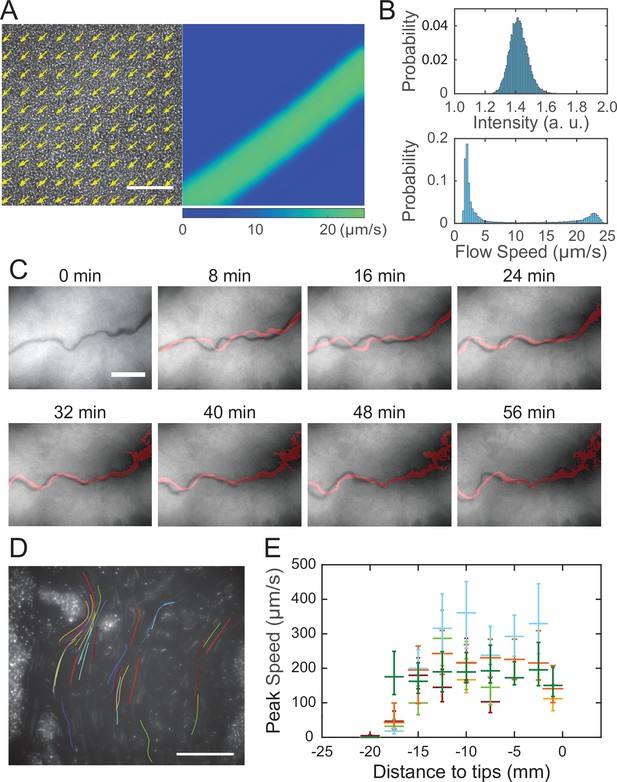
Shear-induced banding during canal development and flow speed profile in canals.
(A) Collective velocity field of cells in a region with homogeneous cell density distribution prior to canal formation. The collective velocity field was measured by particle image velocimetry (PIV) analysis on phase-contrast image sequence and averaged over a time window of 4 s (‘Methods’). Arrows in left panel and colormap in right panel represent collective velocity direction and magnitude, respectively. The velocity direction field in left panel is superimposed onto the phase-contrast image of this region. Both panels share the same scale bar (100 µm). Also see Figure 3—video 1. (B) Probability distributions of phase-contrast image intensity (upper panel) and flow speed (lower panel) associated with panel (A). Unimodal distribution of phase-contrast intensity indicates a homogeneous distribution of cell density. The bimodal distribution of flow speeds shows the occurrence of a high-speed flow regime. (C) Image sequence showing the course of a developing canal in a colony. Cells in the colony hosted the GFP-reporter plasmid expressing PrhlA-gfp(ASV) and the colony was imaged by fluorescence microscopy (‘Methods’). The course of the canal (across the center of each image) had lower cell density due to flushing by rapid flows and thus appeared darker than other areas. The red trace in each image starting from T = 8 min indicates the course of the canal in the previous image (8 min earlier). Scale bar, 125 µm. Also see Figure 3—video 2. (D) The fluorescence image of a canal in a P. aeruginosa colony seeded with ~1% GFP-expressing cells. The image was taken at a distance of ~12.5 mm from the tip of a canal. Colored traces show the trajectories of 38 fluorescent cells being transported in the canal, which were recorded during 0.5 s exposure time of a single image frame. Scale bar, 100 μm. Different colors serve to distinguish trajectories of different cells. (E) Peak flow speed at different locations of canals. The horizontal axis indicates the distance from the canal tips to the measuring position. Horizontal error bars indicate the uncertainty of canal position measurement (1 mm). Vertical error bars indicate the full range of peak speeds measured from >20 cell trajectories. Data from independent experiments are presented in different colors.
Distinct flow regimes occurring in a homogeneous region of a piliated P. aeruginosa (PA14 flgK::Tn5) colony.
The video is associated with Figure 3A and B.
The course of a developing canal in a colony.
Cells in the colony hosted a plasmid expressing the rhamnolipid synthesis reporter PrhlA-gfp(ASV) and the colony was imaged by fluorescence microscopy. Scale bar, 125 µm. Time label is in the format of hh:mm:ss. The video is associated with Figure 3C.
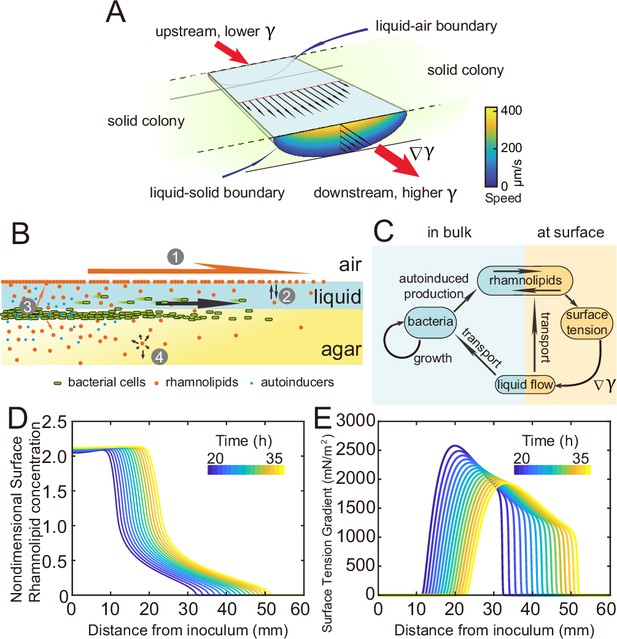
Modeling fluid transport and spatial-temporal dynamics of surface tension gradient in canals.
(A) Hydrodynamic simulation of fluid transport in a simplified canal geometry. The cross-sectional profile of the canal was modeled as a half ellipse with the major and minor axis being 150 μm and 10 μm, respectively. Note that the vertical and horizontal length scales are different. Red arrows indicate the direction of surface tension gradient . Black arrows and colormap show the surface and the bulk flow speed profiles, respectively, generated by a surface tension gradient of imposed at the canal’s upper surface (liquid–air boundary). (B) Schematic showing the key processes involved in the establishment of surface tension gradient in canals. Major constituents of the colony are represented by symbols of different colors: surfactant molecules, orange dots; QS molecule, blue dots; cells, green rods. The key processes are labeled by numbers: ‘1,’ transport of bacteria and biosurfactant at the liquid–air interface driven by surface tension gradient ; ‘2,’ biosurfactant exchange between the liquid–air interface and the bulk phase; ‘3,’ growth of bacteria and the production of biosurfactant under QS regulation; ‘4,’ diffusion of biosurfactant, QS molecules, and nutrient. Arrows indicate the direction of material transport. (C) Schematic showing the coupling of the key processes described in panel (A) and in main text. See Appendix 1 for details. (D, E) Spatio-temporal dynamics of surface-associated surfactant concentration and surface tension gradient obtained by numerical simulations of the mathematical model, as shown in panels (D) and (E), respectively. Also see Appendix 1—figure 4 for the numerical result of the spatial-temporal evolution of other quantities in the model.
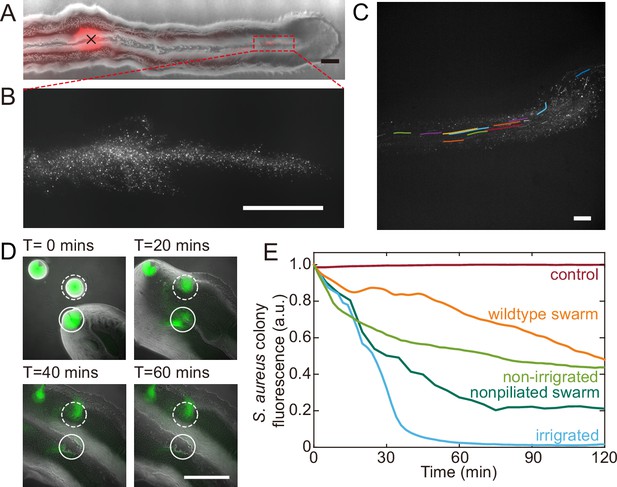
Fluid flows in P. aeruginosa canals transport outer membrane vesicles (OMVs) and help to eradicate S. aureus colonies.
(A) Phase-contrast image of a canal overlaid with the fluorescence image of OMVs (in red). The cross sign marks the location of microinjecting OMV dispersion. The loaded OMVs were transported downstream along the canal and accumulated near the tip. Scale bar, 1 mm. (B) Enlarged view of the OMV fluorescence image at the red dashed box in panel (A). Scale bar, 500 μm. (C) Colored traces show the trajectories of fluorescently labeled OMVs during 1 s exposure time. Scale bar, 100 μm. Also see Figure 5—video 1. (D) Image sequence showing the eradication of an S. aureus colony (GFP-labeled, enclosed by the solid line) that was irrigated by P. aeruginosa canal flows. A nearby S. aureus colony (enclosed by the dashed line) came into contact with the P. aeruginosa colony but did not encounter canal flows. In each panel the fluorescence image of S. aureus colonies is superimposed onto the phase-contrast image. Also see Figure 5—video 2. (E) Temporal dynamics of S. aureus colony biomass after encountering P. aeruginosa PA14 colonies under different conditions. To compare with the effect of potential material transport by flagellar motility (Wu et al., 2011; Xu et al., 2019), curves labeled as ‘wildtype swarm’ and ‘non-piliated swarm’ were shown for S. aureus colonies encountering wildtype P. aeruginosa (with both flagellar and type IV pilus motilities) and non-piliated P. aeruginosa (PA14 ΔpilB; with flagellar motility alone), respectively (see Appendix 1—figure 6 for details; ‘Methods’). At least three replicates were performed for each condition.
Transport of outer membrane vesicles (OMVs) along a canal.
This real-time video records at 10 frames per second the fluorescence of FM1-43 FX-labeled OMVs that were being transported along a canal in a P. aeruginosa (PA14 flgK::Tn5) colony. Purified OMVs were injected into the canal at ~15 mm upstream from the tip of the canal, and the video was acquired after ~2 min at ~5 mm upstream from the tip of the canal. The video is associated with Figure 5C.
Eradication of an S. aureus colony irrigated by P. aeruginosa canal flows.
S. aureus (with constitutive GFP expression) colonies were co-cultured with P. aeruginosa (PA14 flgK::Tn5); see ‘Methods’. Time label (in hh:mm format) shows the elapsed time from full contact between the P. aeruginosa colony and the S. aureus colony near the center. The fluorescence image of S. aureus colonies is displayed in logarithmically transformed pixel values in order to accommodate the broad dynamic range. In each video frame, the fluorescence image is superimposed onto the corresponding phase contrast image acquired simultaneously. As shown in the video, the S. aureus colony irrigated by P. aeruginosa canal flows was quickly eradicated, while a nearby S. aureus colony that came into full contact with the P. aeruginosa colony but did not encounter canal flows retained ~40% colony biomass after ~60 min. The video is associated with Figure 5D.
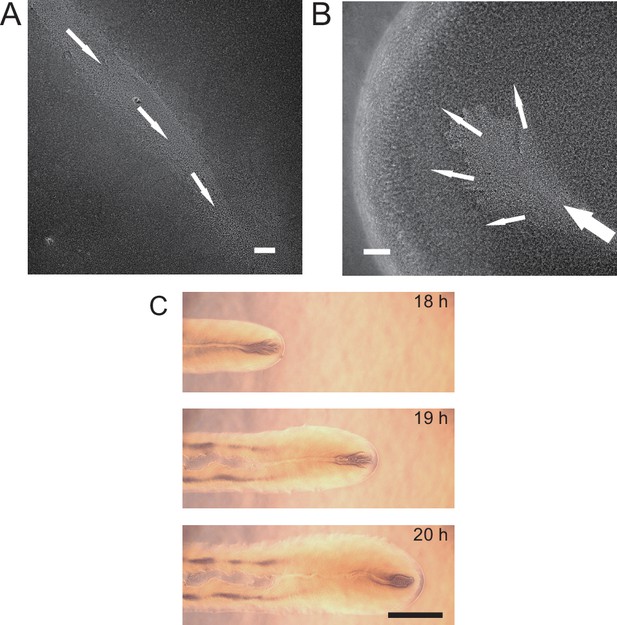
Open channels supporting long-range directed material transport in P. aeruginosa colonies.
(A) Phase-contrast microscopy image of an open channel in a P. aeruginosa (PA14 flgK::Tn5) colony. Arrows indicate the flow direction. Scale bar, 100 μm. Also see Appendix 1—figure 1—video 1. (B) Phase-contrast microscopy image taken near the tip of an open channel in a P. aeruginosa (PA14 flgK::Tn5) colony. Arrows indicate the flow direction. Scale bar, 100 μm. Also see Appendix 1—figure 1—video 2. (C) Image sequence taken by a DSLR camera via a ×4 phase-contrast objective lens showing the development of an open channel (or canal) in a branching colony of P. aeruginosa (PA14 flgK::Tn5). Scale bar, 1 mm. Also see Appendix 1—figure 1—video 3. The fluid flow in bacterial canals was sensitive to water content in the air environment and it was easily disrupted by decrease of humidity. Cells translocating along the open channels eventually settled in near the colony edge and they may contribute to colony expansion. Fluid flow in open channels on average went toward the colony edge and stopped abruptly at the very end (i.e., the tip) of an open channel, disappearing into the dense layer of cells near the edge (panel B; Appendix 1—figure 1—video 2).
Cellular flow in an open channel of a piliated P. aeruginosa (PA14 flgK::Tn5) colony.
This real-time video is associated with Appendix 1—figure 1A.
Cellular flow near the tip of an open channel in a piliated P. aeruginosa (PA14 flgK::Tn5) colony.
The flow slowed down and stopped abruptly at the tip, disappearing into the dense layer of cells near the edge of the colony. Note that the colony edge had ceased expansion and in general, canal formation does not necessarily coincide with colony expansion. This real-time video is associated with Appendix 1—figure 1B.
Zoomed-in view of a developing bacterial canal in a branching colony of piliated P. aeruginosa (PA14 flgK::Tn5).
Time label shows the elapsed time from inoculation (hh:mm). The video is associated with Appendix 1—figure 1C.
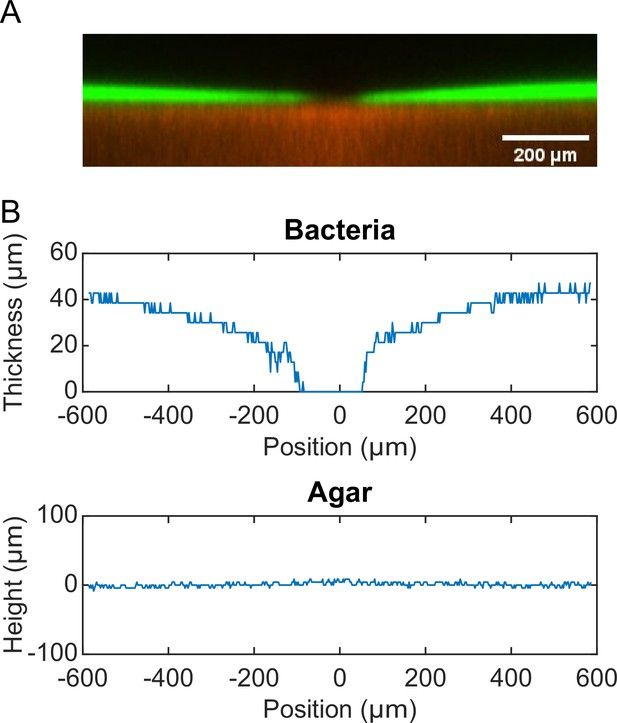
Height profiles of colony and agar.
(A) Cross-sectional view of the colony (green fluorescence) and the agar underneath the colony (red fluorescence) measured by laser scanning confocal microscopy (see ‘Methods’). A canal is located at the center of the image. (B) Thickness profile of the colony (upper panel) and height profile of the agar underneath the colony (lower panel) associated with panel (A). The height of agar at position 600 µm was chosen as the reference level (i.e., height = 0 µm). The thickness of the colony is low inside the canal region due to flushing of fluid flows. The height of agar is uniform, showing that canal formation is not associated with agar degradation.
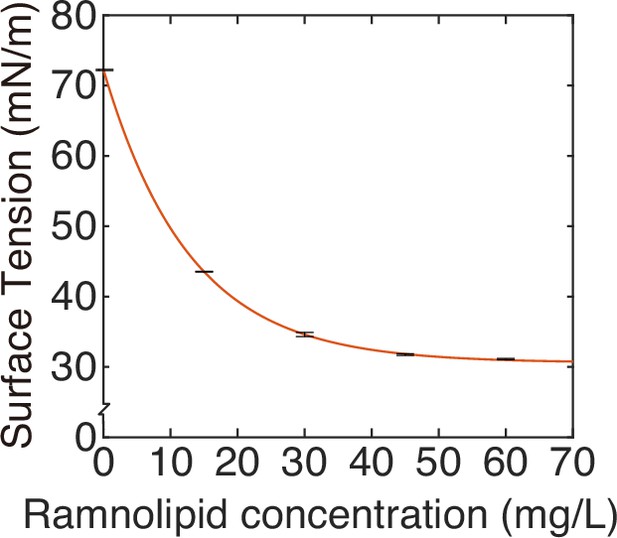
Surface tension of rhamnolipid solutions as a function of rhamnolipid concentration.
The relation between bulk rhamnolipid concentration and the steady-state surface tension. Steady-state surface tension was measured by pendant drop assay with a commercial contact-angle meter (OCA25, DataPhysics, Germany). The rhamnolipid solutions were prepared by dissolving rhamnolipids (in solid form) in M9DCAA medium. Error bars indicate the standard deviation (N=5). The line is a fit of data points to an exponential decay function (Equation 1 of Appendix 1 – text).
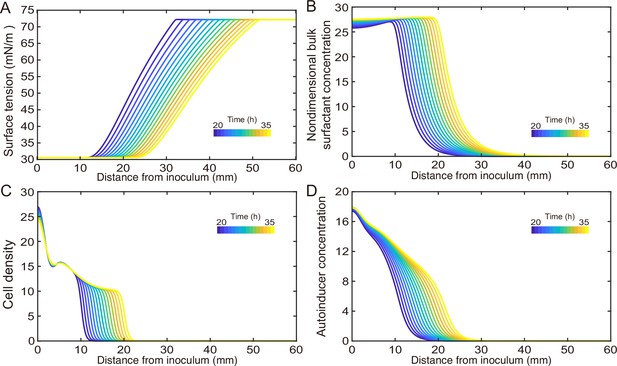
Spatial-temporal dynamics of key model variables related to the development of surface tension gradient in canals.
This figure is associated with Figure 4. The spatial-temporal dynamics of the following variables used in the model presented in main text and in the text of Appendix 1 were plotted: surface tension (A); bulk biosurfactant concentration (B); bacterial density (C); autoinducer concentration (D).
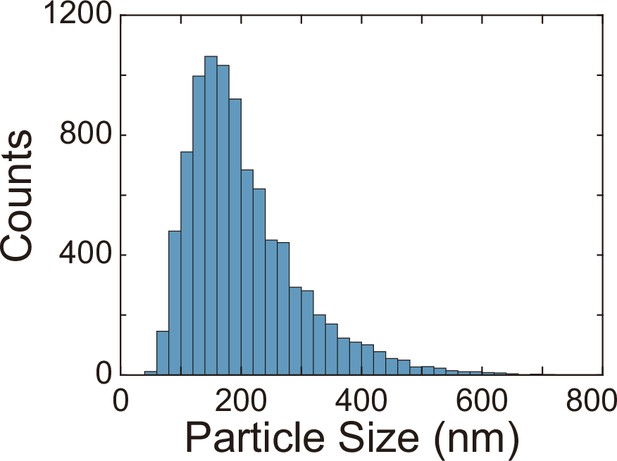
Size distribution of outer membrane vesicles (OMVs).
The OMVs were derived from canal-forming P. aeruginosa (PA14 flgK::Tn5) and the size distribution was measured by a particle sizer (NanoSight LM10, Malvern Instruments). See ‘Methods.’
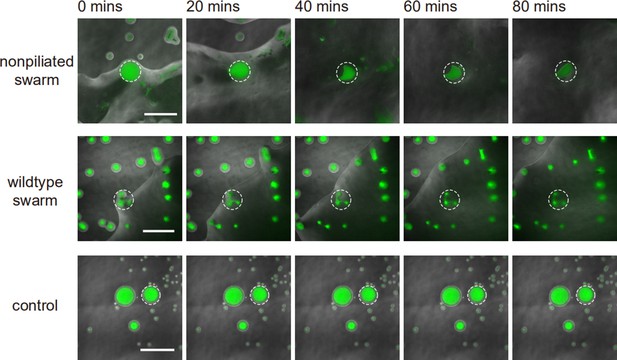
Interaction between S. aureus and P. aeruginosa PA14 colonies.
This figure is associated with Figure 5D and E. To compare with the effect of potential OMV transport due to flagellar motility, we co-cultured swarms of flagellated P. aeruginosa PA14 with S. aureus colonies under the same conditions as used in Figure 5D. The image sequences show the temporal variation of S. aureus colony biomass (green fluorescence) under the following conditions: top, in contact with the swarming colony of PA14 ΔpilB mutant; middle, in contact with the swarming colony of wildtype PA14; bottom, control experiment in the absence of P. aeruginosa. Both PA14 ΔpilB mutant and wildtype PA14 have flagellar motility, but neither of them can form canals. In each panel, the fluorescence image of S. aureus colonies (green) is superimposed onto the phase-contrast image. The dashed circles mark the region with S. aureus colonies for fluorescence analysis in Figure 5E. As shown in Figure 5E, the S. aureus colonies encountering expanding P. aeruginosa swarms were destructed to a lesser extent compared to the case that were irrigated by canal flows, retaining ~20% and ~50% of cell mass after 60 min of contact with swarming colonies of P. aeruginosa PA14 ΔpilB mutant and wildtype PA14, respectively.
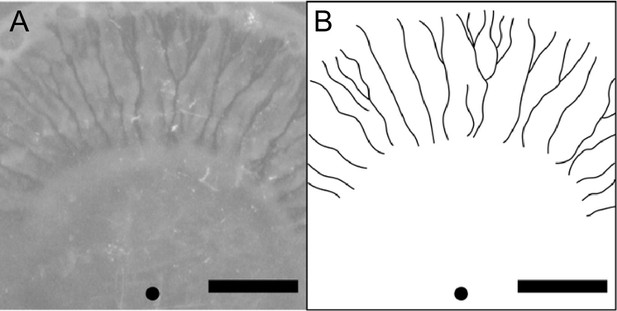
Canal formation in Serratia marcescens colonies.
Overnight culture of S. marcescens grown in LB medium was inoculated on 0.6% Eiken agar plates and photographed in a custom-built imaging incubator made of PMMA. The temperature of the incubator was maintained at 30°C and the images of canals were photographed by a digital single-lens reflex camera. (A) Canal appeared ~4 hr after colony inoculation. (B) Sketch of the canals in panel (A) for better visualization. The black dot represents the inoculum. Scale bars, 500 µm.
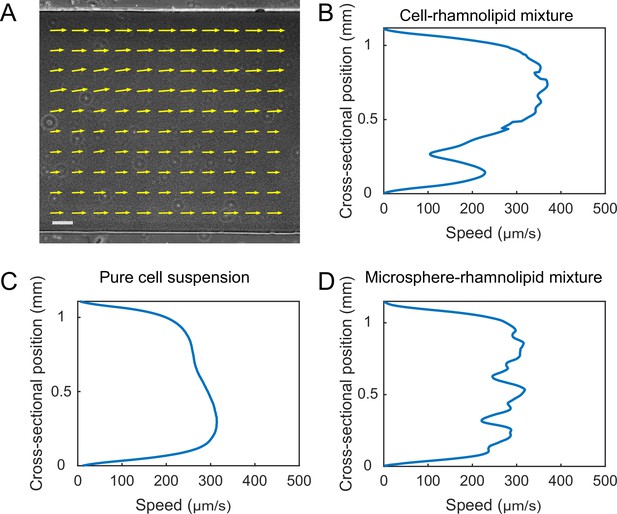
Shear banding of cell-rhamnolipid mixture in PDMS microfluidic channels.
(A) Flow velocity field of cell-rhamnolipid mixture in a microfluidic channel. The microfluidic channel had a rectangular cross section (200 μm of height, 1.1 mm of width). Cells (PA14 flgK::Tn5; ZK3367) and rhamnolipids were mixed at a final density (concentration) of 1.9 × 1010 cells/mL and 10 mg/mL, respectively, and driven by a syringe pump at a flow rate of 5 µL/min. The velocity field was measured by particle image velocimetry (PIV) analysis on phase-contrast image sequence and averaged over a time window of 5 s. The velocity field is superimposed onto one of the analyzed phase-contrast images. Arrows indicate velocity vectors. Scale bar, 100 µm. (B) Cross-sectional flow speed profile computed based on velocity field data shown in panel (A). Each data point represents local velocity averaged in a domain of 41.6 µm × 41.6 µm centered around the cross-sectional position. (C, D) Cross-sectional flow speed profile in microfluidic channels filled with other fluids. Data in panel (C) was obtained with pure cell suspension (1.9 × 1010 cells/mL), and data in panel (D) with a mixture of rhamnolipids (10 mg/mL) and 1.1 µm microsphere suspension (~1.9 × 1010 particles/mL). These fluids were driven through microfluidic channels by a syringe pump at a flow rate of 3 µL/min. The flow speed profiles were computed based on the respective time-averaged velocity field, which was obtained by PIV analysis on phase-contrast image sequence and averaged over a time window of 5 s. Each data point in (C, D) represents local velocity averaged in a domain of 41.6 µm × 41.6 µm centered around the cross-sectional position. See ‘Methods’ for details of experiments and image analysis associated with all panels.
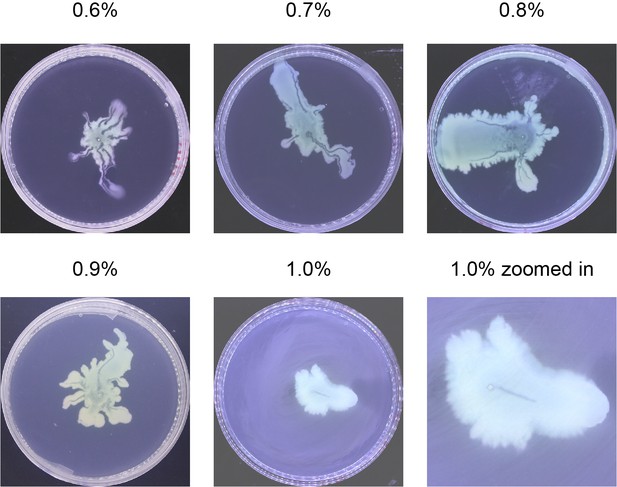
Representative images of canal development at different agar concentrations.
The colonies were from the same batch and incubated under the same condition (30°C) for the following durations: 0.6–0.8%, 19 hr; 0.9%, 24 hr; 1.0%, 36 hr. Canals can be found in different agar (infused with M9DCAA medium) concentrations from 0.6% to 1.0%, in addition to the 0.5% agar used in the main text. Note that a canal in the colony on the 1.0% plate is located at the center (see the zoomed-in view).
Videos
Rapid cellular flow streaming through a non-piliated P. aeruginosa (PA14 ΔpilB) colony.
Cells are motile outside the stream.
Effect of externally applied counteracting surface tension gradient on fluid transport in canals.
An agar patch infused with surfactant Tween 20 (50 mg/mL) was placed at ~1 cm in front of a colony branch of piliated P. aeruginosa (PA14 flgK::Tn5); see ‘Methods’. As shown in the video, immediately following this operation, fluid flows in the canal gradually ceased and the canal slowly retracted. Time label shows the elapsed time from placing the agar patch (mm:ss).
Tables
Reagent type (species) or resource | Designation | Source or reference | Identifiers | Additional information |
---|---|---|---|---|
Strain, strain background (Pseudomonas aeruginosa) | Wildtype PA14 | Roberto Kolter, Harvard University (Lee et al., 2011) | ZK3436 | Having both flagellar and type IV pilus motilities |
Strain, strain background (P. aeruginosa) | Piliated P. aeruginosa PA14 | Roberto Kolter, Harvard University (Lee et al., 2011) | ZK3367 | Without flagellar motility but retaining type IV pilus motility |
Strain, strain background (P. aeruginosa) | Non-piliated P. aeruginosa PA14 | Roberto Kolter, Harvard University (Lee et al., 2011) | ZK3351 | Without type IV pilus motility but retaining flagellar motility |
Strain, strain background (P. aeruginosa) | Non-motile P. aeruginosa PA14 | George A. O’Toole, Dartmouth College (Beaussart et al., 2014) | SMC6589 | Without either flagellar or type IV pilus motility |
Strain, strain background (P. aeruginosa) | P. aeruginosa deficient in rhamnolipid production ΔrhlA | This paper | PA14 flgK::Tn5 ΔrhlA | Deficient in rhamnolipid production |
Strain, strain background (Staphylococcus aureus) | Staphylococcus aureus clinical strain that expresses GFP constitutively | Toledo-Arana et al., 2005 | Isolate 15981 harboring a plasmid pSB2019-gfp expresses GFP constitutively | |
Strain, strain background (Serratia marcescens) | Serratia marcescens | ATCC | ATCC 274 | |
Sequence-based reagent | 1-rhlA_UpF | This paper | PCR primers | agctcggtacccggg GGGTGATTTCCTACGGGGTG |
Sequence-based reagent | 2-rhlA_UpR | This paper | PCR primers | CTTCGCAGGTCAAGGGTTC ACCGCATTTCACACCTCCCAA |
Sequence-based reagent | 3-rhlA_DownF | This paper | PCR primers | TTGGGAGGTGTGAAATGCGG TGAACCCTTGACCTGCGAAG |
Sequence-based reagent | 4-rhlA_DownR | This paper | PCR primers | cgacggccagtgcca CCGTACTTCTCGTGAGCGAT |
Sequence-based reagent | rhlA_F | This paper | PCR primers | GACAAGTGGATTCGCCGCA |
Sequence-based reagent | rhlA_R | This paper | PCR primers | TTGAACTTGGGGTGTACCGG |
Sequence-based reagent | rhlAGENE_F | This paper | PCR primers | GGTCAATCACCTGGTCTCCG |
Sequence-based reagent | rhlAGENE_R | This paper | PCR primers | GCTGATGGTTGCTGGCTTTC |
Sequence-based reagent | Pk18-F | This paper | PCR primers | TGCTTCCGGCTCGTATGTTG |
Sequence-based reagent | Pk18-R | This paper | PCR primers | GCGAAAGGGGGATGTGCTG |
Recombinant DNA reagent | Plasmid pMHRA | Yang et al., 2009 | Plasmid | Contains an RlhR-regulated PrhlA-gfp(ASV) fusion inserted into the vector pMH391 |
Chemical compound, drug | Q5 High-Fidelity DNA Polymerase | NEB, USA | Cat# M0515 | |
Chemical compound, drug | Gibson Assembly Master Mix | NEB, USA | Cat# E2611 | |
Chemical compound, drug | Taq Polymerase | Thermo Fisher, USA |
Primers.
No. | Oligo name | Sequence 5' to 3' |
---|---|---|
1 | 1-rhlA_UpF | agctcggtacccgggGGGTGATTTCCTACGGGGTG |
2 | 2-rhlA_UpR | CTTCGCAGGTCAAGGGTTCACCGCATTTCACACCTCCCAA |
3 | 3-rhlA_DownF | TTGGGAGGTGTGAAATGCGGTGAACCCTTGACCTGCGAAG |
4 | 4-rhlA_DownR | cgacggccagtgccaCCGTACTTCTCGTGAGCGAT |
5 | rhlA_F | GACAAGTGGATTCGCCGCA |
6 | rhlA_R | TTGAACTTGGGGTGTACCGG |
7 | rhlAGENE_F | GGTCAATCACCTGGTCTCCG |
8 | rhlAGENE_R | GCTGATGGTTGCTGGCTTTC |
9 | Pk18-F | TGCTTCCGGCTCGTATGTTG |
10 | Pk18-R | GCGAAAGGGGGATGTGCTG |
Simulation parameters.
Parameter | Description | Value | Unit | Robust range * |
---|---|---|---|---|
Biosurfactant synthesis rate | - | |||
Bacterial growth rate | - | |||
Nutrient concentration for half maximal metabolic activity | 1 | Nondimensio-nal | N/A | |
Half-activation threshold of QS auto-inducer | 1 | Nondimensio-nal | N/A | |
Auto-inducer synthesis rate | - | |||
Auto-inducer degradation rate | 0 - | |||
Auto-inducer basal production rate | - | |||
m † | Hill coefficient of QS regulation | 2 | N/A | |
Parameter of growth-repression due to QS-regulated biosurfactant production | 0.3 | Nondimensio-nal | 0–0.5 | |
k ‡ | Reaction rate constant for biosurfactant transfer between the bulk phase and the interface | N/A | ||
A § | Ratio between bulk surfactant concentration and square of the steady-state surface density | 6.125 | Nondimensio-nal | 2–10 |
ηρ‡ | Parameter of advective bacterial transport | N/A | ||
ηΓ‡ | Parameter of advective biosurfactant transport | N/A | ||
Diffusion coefficient of biosurfactant in bulk phase | 0 - | |||
Diffusion coefficient of biosurfactant at liquid–air interface | 0 - | |||
Diffusion coefficient of bacteria | 0 - | |||
Diffusion coefficient of auto-inducers | - | |||
Diffusion coefficient of nutrient | 0 - |
-
*
The results shown in Figure 4D and E are robust to variation of parameters within the indicated range.
-
†
The value of this parameter was taken from Payne et al., 2013.
-
‡
These parameters are chosen to yield the width of the plateau of surface tension gradient distribution (Figure 4D) being ~25 mm (matching the experimental results).
-
§
The value of this parameter was taken from Hanyak et al., 2012.