A common cis-regulatory variant impacts normal-range and disease-associated human facial shape through regulation of PKDCC during chondrogenesis
Figures
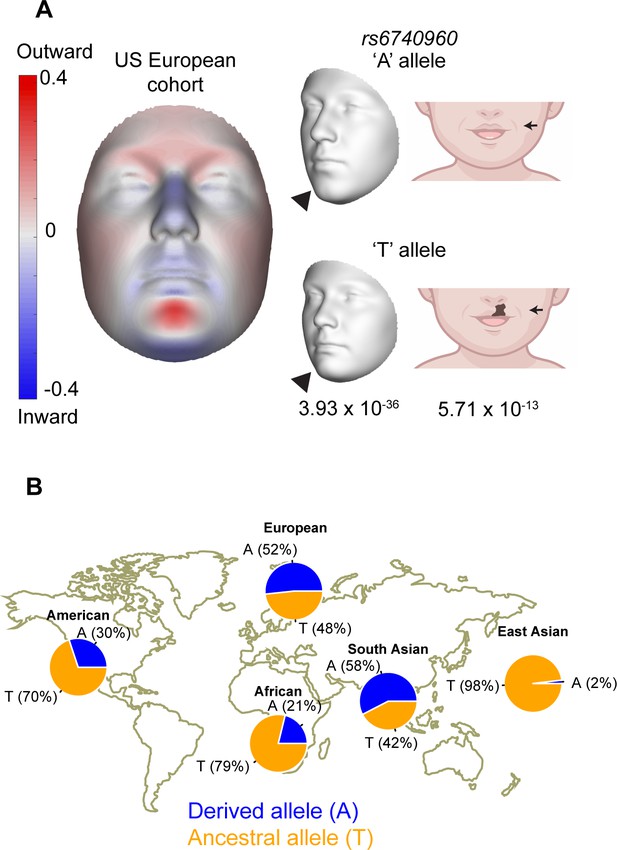
Association of rs6740960 (A/T) with normal-range facial shape variation and its allele frequency among world populations.
(A) Genome-wide association studies (GWAS) of normal-range facial variation in Europeans identified rs6740960 as a lead single nucleotide polymorphism (SNP) at the 2p21 locus (Claes et al., 2018). 3D facial morphs and facial effects of the rs6740960 are shown, with blue and red indicating a local shape depression and protrusion, respectively, due to the ‘T’ allele. Note the protrusion of the lower jaw and zygomatic regions and retrusion of the entire central midface associated with the ‘T’ allele. An independent GWAS linked rs6740960 with susceptibility to non-syndromic cleft lip with or without palate in Europeans (Ludwig et al., 2017). (B) Allele frequency distribution for rs6740960 across populations of the 1000 Genomes Project. (see also Figure 1—figure supplements 1–2).
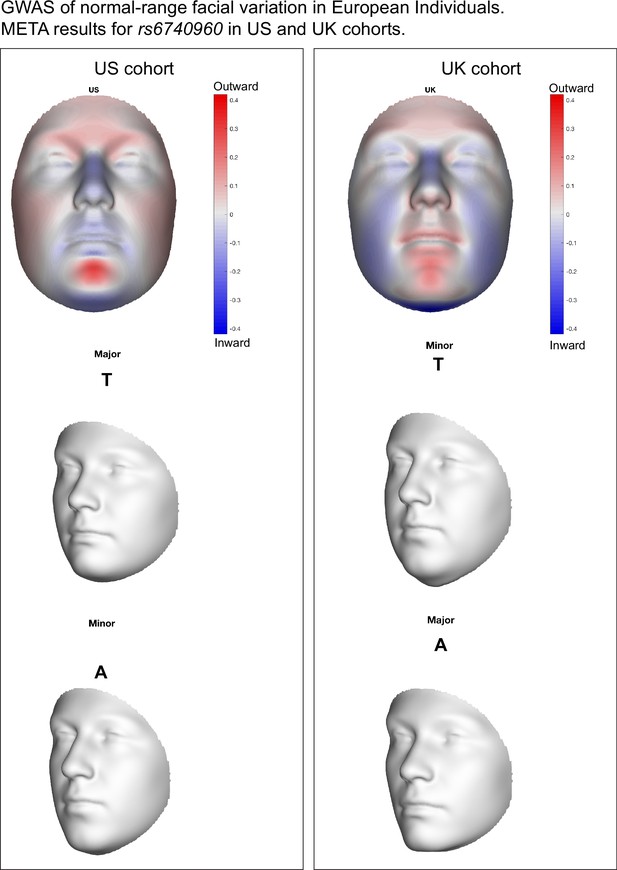
Facial effects for the rs6740960 (A/T) single nucleotide polymorphism (SNP) implicated in normal-range facial variation within the United Kingdom and the United States of America European study cohorts of White et al., 2021.
Blue/red coloring indicates depression/protrusion local variation due to the effect of the ‘T’ allele. Gray 3D face morphs show the averaged effects of the ‘A’ or ‘T’ alleles within either the UK or US cohorts from White et al., 2021. Note that the ‘minor’ and ‘major’ allele designations are reversed amongst these two cohorts due to rs6740960’s approximate 50% heterozygosity in European populations.
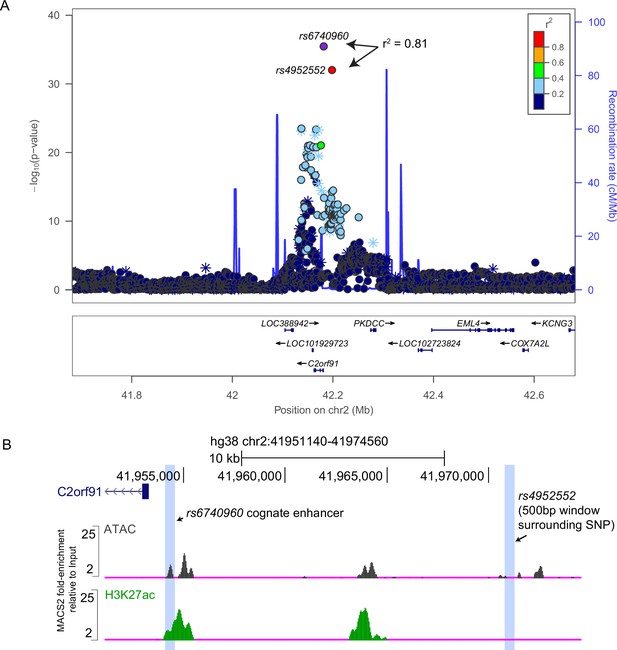
Linkage Disequilibrium (LD) calculation, and epigenetic features, at the 2p21 locus associated with normal-range lower jaw variation (see White et al., 2021).
(A) LocusZoom plots indicating gene annotations, Genome-wide association studies (GWAS) significant single nucleotide polymorphisms (SNPs) (as -log10(p-value)), linkage disequilibrium (as r2 using the 1000 Genomes Project European cohort), and recombination rates (as centimorgans per megabase). Note that rs6740960 has r2=0.813 with only one other SNP, rs4952552, indicated in red. (B) Genome browser image showing genomic location of rs4952552, and its lack of chromatin marks indicative of an active cis-regulatory element. Chromatin accessibility, as ATAC-seq, and ChIP-seq signals of the active chromatin mark H3K27ac from human CNCCs are shown (see Prescott et al., 2015). rs6740960 and rs4952552 are ~16.5 Kb apart and reside within the same haplotype block.
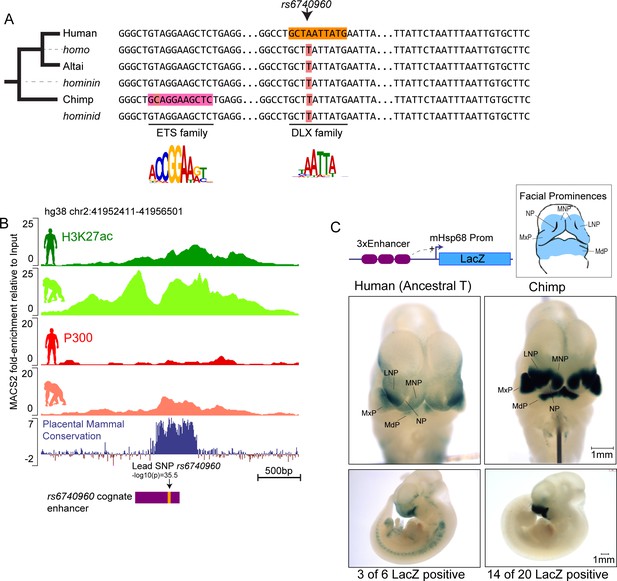
Sequence and activity changes of rs6740960 cognate enhancer in hominids.
(A) Multi-species abridged alignment of the genomic region surrounding rs6740960. Extant species are Human, Altai Neanderthal and Chimp, and ancestral reconstructed species are Homo, Hominin, and Hominid. Human-specific substitution (‘A’ at rs6740960) and chimp-specific substitution (T→C) are highlighted, along with transcription factors predicted to gain binding affinity via the substitution and their consensus sequence motifs. (B) Genome browser track showing the location of rs6740960 and its overlap with H3K27ac and P300 ChIP-seq signal from human and chimpanzee CNCCs. Note higher enrichments in the chimpanzee. Region corresponding to the sequence tested in (C) is highlighted in purple. ChIP-seq data from Prescott et al., 2015 (C) LacZ Transgenic Mouse Reporter assays performed using Human (with an ancestral ‘T’ allele) and Chimp orthologs of the genomic region surrounding rs6740960. Triplicate copies of the 500 bp sequence orthologs are used in the reporter vector. Both human and chimp orthologs show LacZ reporter activity restricted to the head and face prominences in E11.5 transgenic mice and overlapping upper and lower jaw primordia (Maxillary Prominence-MxP and Mandibular Prominence-MdP). The Chimp ortholog shows stronger activity within both MxP and MdP and within the Lateral and Medial Nasal Processes (LNP and MNP). NP – Nasal Pit. LacZ-positive animals that show the same staining profile of expression domains are numbered. See Figure 2—figure supplement 1 for images of all LacZ-positive embryos.
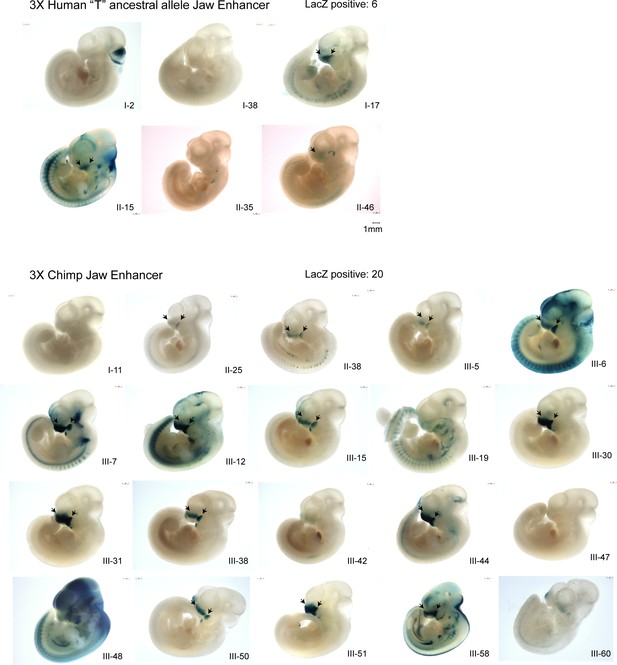
LacZ transgenic mouse reporter assay results for the 500 bp Human ‘T’ allele and Chimp ortholog of the rs6740960 cognate enhancer.
Triplicate copies of each ortholog were cloned into the LacZ reporter construct and tested. At least three LacZ-positive embryos were needed to show the same stained expression domain (denoted by arrows). The identification label per embryo denotes injection batch, as a Roman numeral, and an embryo unique ID in said batch.
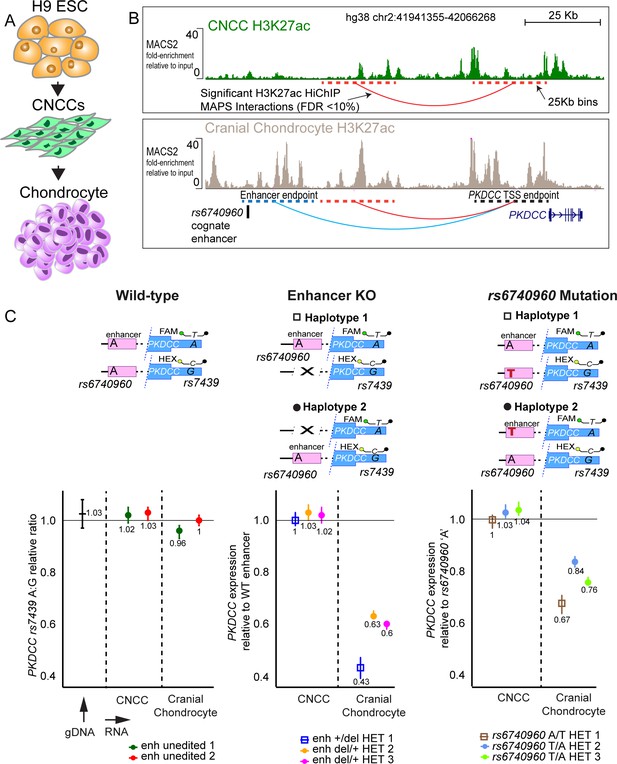
rs6740960 regulates expression of PKDCC in cranial chondrocytes.
(A) Schematic of the in vitro differentiation protocol for obtaining CNCCs and cranial chondrocytes from human embryonic stem cells (hESCs). (B) Mapping of long-range chromatin interactions by H3K27ac HiChIP in CNCCs and cranial chondrocytes revealed significant contacts between rs6740960 cognate enhancer and PKDCC promoter in chondrocytes. H3K27ac ChIP-seq genome browser tracks at the locus from CNCCs (top) and cranial chondrocytes (bottom) are shown. Significant HiChIP interactions, called at FDR <10% and within 25 Kb bins given the resolution of this dataset (dotted lines), are shown underneath ChIP-seq tracks. Fold-change relative to input samples, as computed by the ENCODE chipseq2 pipeline, are shown on the y-axis. (C) Changes in allele-specific PKDCC expression upon deletion of rs6740960 cognate enhancer or mutation of rs6740960 to heterozygosity. Wild-type un-edited cells, homozygous for rs6740960 (A/A), show no PKDCC allelic imbalance, as expected. Three independent CRISPR-Cas9 heterozygous enhancer deletion hESC lines show a 40–60% decrease in PKDCC expression for the enhancer deletion allele selectively in cranial chondrocytes. Three independent heterozygous rs6740960 (A/T) edited lines show a 15–30% decrease in PKDCC expression due to the ancestral ‘T’ allele. FAM/HEX droplet-digital PCR (ddPCR) probes that distinguish PKDCC alleles based on rs7439 (A/G) heterozygous single nucleotide polymorphism (SNP) are shown. This same SNP was used to phase the enhancer deletion or SNP mutation with PKDCC (see Figure 3—figure supplements 3–4). Genomic DNA from H9 wild-type cells was used to verify the FAM/HEX ratio of one at rs7439.
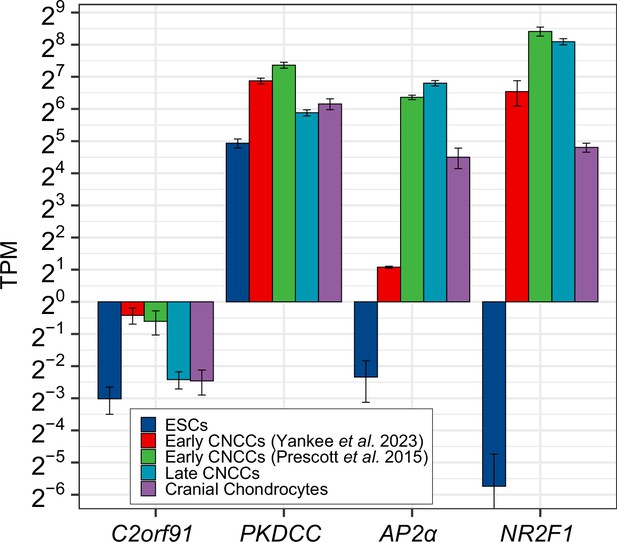
RNA expression of lincRNA C2orf91/LINC02898 and protein-coding gene PKDCC, and two marker genes of CNCC identity (AP2α and NR2F1).
RNA-seq data, as uniformly processed through the ENCODE RNA-seq pipeline, is shown for human embryonic stem cells (hESCs) and in vitro derived CNCC and cranial chondrocyte cells. In all cell types surveyed, lincRNA C2orf91 is very low expressed (TPM <1). Data for hESCs, early and late CNCCs were obtained from Prescott et al., 2015, data for early CNCCs obtained from a different hESC to CNCC in vitro differentiation protocol were obtained from Yankee et al., 2023, and data for cranial chondrocytes obtained from Long et al., 2020.
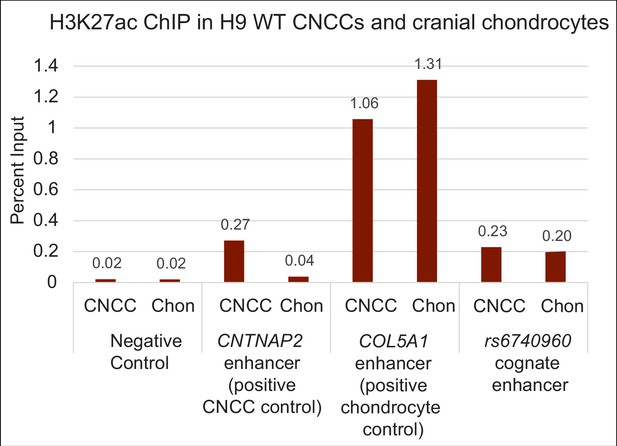
H3K27ac ChIP-qPCR data from H9 human embryonic stem cell (hESC) derived CNCCs and cranial chondrocyte cells, as shown in Figure 3A and B.
Primers are designed for an intergenic region devoid of active chromatin marks (negative control), a CNCC-enriched enhancer adjacent to the gene CNTNAP2, a chondrocyte-enriched enhancer adjacent to the COL5A1 gene, and the rs6740960 cognate enhancer. H3K27ac ChIP-qPCR data indicates a similar deposition of this chromatin modification mark at the rs6740960 cognate enhancer in CNCCs and cranial chondrocytes.
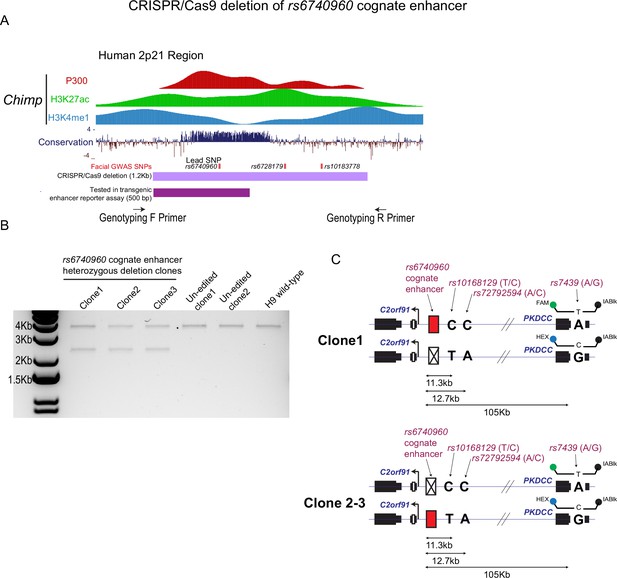
Generation of a 1.2 Kb deletion at the rs6740960 cognate enhancer using CRISPR/Cas9 genome editing of H9 human embryonic stem cells (hESCs).
(A) Genome browser tracks depicting P300, H3K27ac, and K3K4me1 ChIP-seq data from Chimpanzee C0818 cell-line collected in Prescott et al., 2015. The P300 profile guided our selection of the 1.2 Kb enhancer region for deletion. Face-shape-associated Genome-wide association studies (GWAS) single nucleotide polymorphisms (SNPs) from White et al., 2021 are noted. (B) Genotyping PCR results of clonal hESC lines indicate three heterozygous enhancer deletion clones (Clones 1–3). Two hESC lines that underwent the editing process but remained un-edited wild-type clones are shown. H9 hESC cells are shown as a reference. (C) PCR strategy for phasing the 1.2 Kb enhancer deletion with PKDCC 3’UTR heterozygous SNP (rs7439). PCR primers were used to amplify a 12.7 Kb region spanning the enhancer deletion with the closest pre-phased heterozygous SNPs (rs10168129 and rs72792594) within H9 hESCs, as identified by 10 X Genomics whole-genome sequencing data from Long et al., 2020. rs10168129 and rs72792594 allelic configuration was used to phase the enhancer deletion allele with PKDCC’s 3’UTR heterozygous SNP (rs7439), which resides 105 Kb away from the enhancer. The enhancer deletion allele was targeted to alternate diploid haplotypes.
-
Figure 3—figure supplement 3—source data 1
Unprocessed gel blot, as SCN file, and labeled TIFF image corresponding to Figure 3—figure supplement 3B.
SCN file can be viewed using Bio-Rad’s Image Lab Software.
- https://cdn.elifesciences.org/articles/82564/elife-82564-fig3-figsupp3-data1-v1.zip
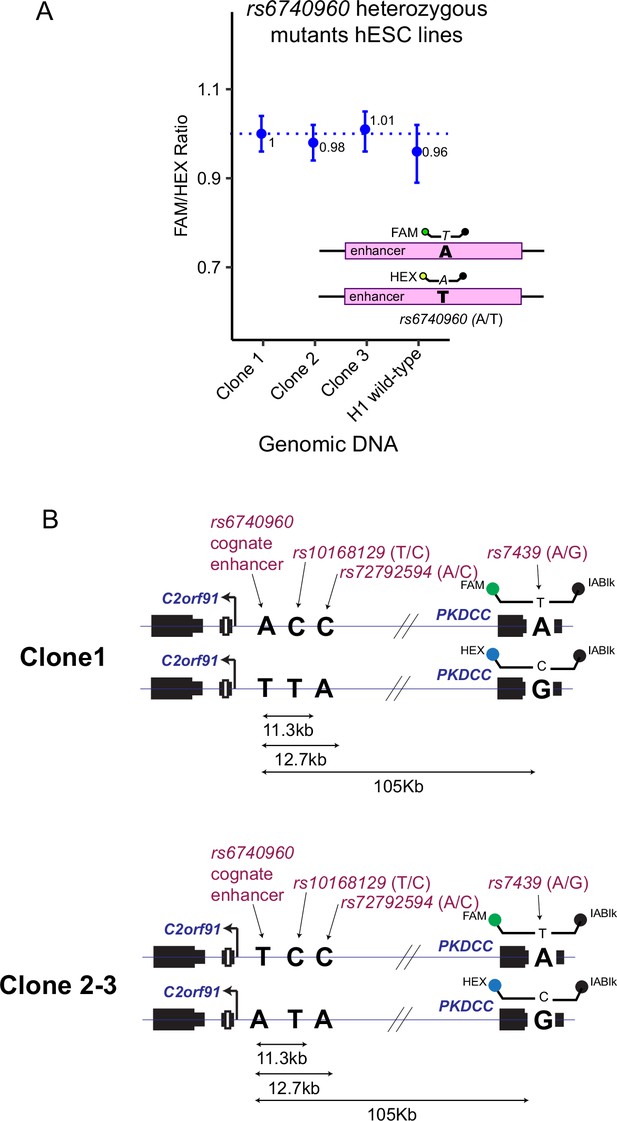
Generation of A-to-T point mutation at rs6740960 using CRISPR/Cas9 genome editing of H9 human embryonic stem cells (hESCs).
(A) Droplet-digital PCR (ddPCR) probes sensing either the ‘A’ or ‘T’ allele at rs6740960 showed a ratio of 1 in the genomic DNA of three heterozygous clones. Results from H1 hESCs, which are naturally A/T heterozygous at rs6740960, are shown as a reference. (B) PCR strategy was used to phase the rs6740960 heterozygous alleles with the pre-phased alleles of rs10168129, rs72792594, and ultimately, PKDCC’s 3’UTR heterozygous single nucleotide polymorphism (SNP) (rs7439).
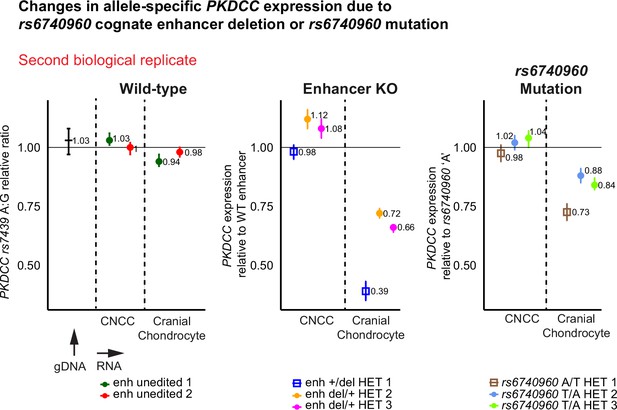
PKDCC allele-specific droplet-digital PCR (ddPCR) expression profiling conducted from a second independent differentiation of unedited wild-type, heterozygous enhancer knock-out, and heterozygous rs6740960 mutant human embryonic stem cell (hESC) lines.
These results are concordant between biological replicates, where both the enhancer deletion allele and the enhancer mutant rs6740960 ‘T’ allele show a reduction in PKDCC expression when compared to the wild-type enhancer bearing rs6740960 ‘A’ allele. This reduction is larger in the enhancer knock-out clones versus the rs6740960 mutant clones, and this effect is only observed within cranial chondrocytes and not in CNCCs.
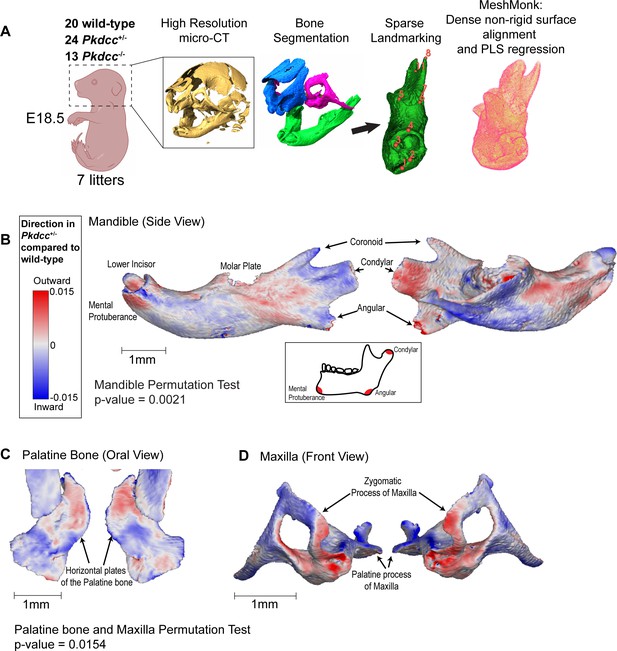
Morphogenetic assessment of Pkdcc dosage on cranial skeletal variation in mice.
(A) Workflow of cranial morphometric analysis from wild-type and Pkdcc mutant mice. Following colony expansion, wild-type, Pkdcc+/- and Pkdcc-/- E18.5 embryos were fixed and imaged using high-resolution micro-CT. Following bone segmentation and sparse landmarking, bone surfaces were non-rigidly aligned to template samples with the MeshMonk software, which facilitated a dense correspondence map between all animals’ surfaces. Partial least squares (PLS) regression was used to analyze genotype-to-phenotype effects. (B, C, D) Bone surface variation between wild-type and Pkdcc+/- heterozygous mutant mice for the Mandible (B), Palatine Bone (C), and the Maxilla (D). Blue and red coloring represent local depression and protrusion of the bone surface, respectively, due to 50% reduction in Pkdcc expression. (B) Pkdcc+/- mice exhibit overall significant shape change in the mandible (Permutation test global p-value = 0.0021). Local variation in the mandibular ramus, angular process, condylar process, and the mental protuberance contribute to the overall elongation of the mandible (See also Figure 4—videos 6–7). (C,D) Pkdcc+/- mice exhibit overall significant shape change in the Maxilla and Palatine bones (Permutation test global p-value = 0.015). (C) Pkdcc+/- mice have a wider distance between the horizontal plates of the palatine bone, a structure that forms the posterior part of the hard palate (See also Figure 4—videos 8–10). (D) Significant variation in the shape of maxilla, especially within the zygomatic arches between wild-type and Pkdcc+/- animals. Similar analysis performed between wild-type and Pkdcc-/- animals are shown in Figure 4—videos 1–2 (mandible), and Figure 4—videos 3–5 (maxilla/palatine). (see also Figure 4—figure supplement 1).
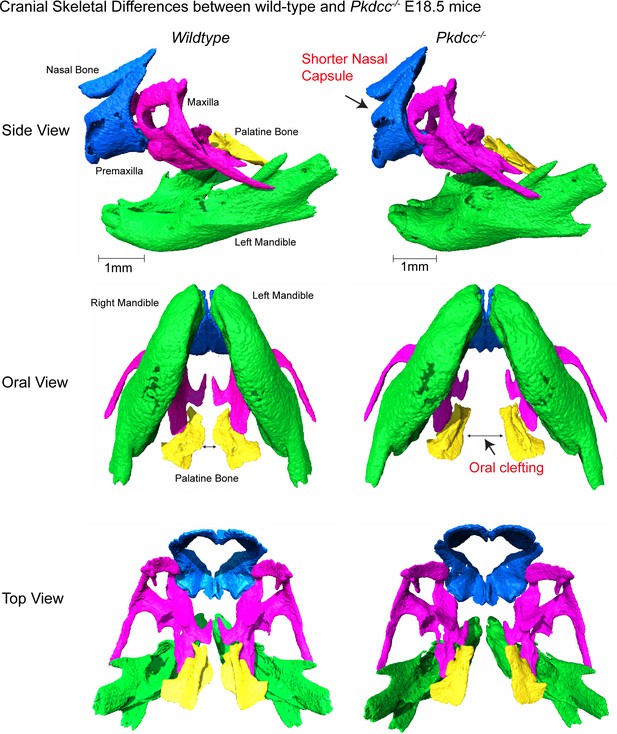
Micro-CT images demonstrating cranial skeletal differences between wild-type and Pkdcc-/- mutant E18.5 mouse embryos.
As reported in previous studies (Imuta et al., 2009; Kinoshita et al., 2009), Pkdcc-/- animals have shorter nasal capsule, and oral clefting phenotypes compared to wild-type animals. Cranial bone colors are nasal and premaxilla bones (blue), maxilla (pink), left and right mandibles (green), and palatine bone (yellow).
Medial side view of the left mandible shape effect changes between averaged wild-type and Pkdcc-/- E18.5 mouse embryos.
The movie progresses from the phenotype in wild-type embryos to Pkdcc-/- embryos and back to wild-type embryos. Movies depict significant shape variation in the mandibular ramus and mental regions in Pkdcc-/- embryos.
Lateral side view of the left mandible shape effect changes between averaged wildtype and Pkdcc-/- E18.5 mouse embryos.
The movie progresses from the phenotype in wild-type embryos to Pkdcc-/- embryos and back to wild-type embryos. Movies depict significant shape variation in the mandibular ramus and mental regions in Pkdcc-/- embryos.
Oral view of the maxilla and palatine bone shape effect changes between averaged wild-type and Pkdcc-/- E18.5 mouse embryos.
The movie progresses from the phenotype in wild-type embryos to PKDCC-/- embryos and back to wild-type embryos. Movies depict a widened distance between the symmetric palatine process of the maxilla and the horizontal plates of the palatine bone in Pkdcc+/- embryos.
Top view of the maxilla and palatine bone shape effect changes between averaged wild-type and Pkdcc-/- E18.5 mouse embryos.
The movie progresses from the phenotype in wild-type embryos to PKDCC-/- embryos and back to wild-type embryos. Movies depict a widened distance between the symmetric palatine process of the maxilla and the horizontal plates of the palatine bone in Pkdcc+/- embryos.
Front view of the maxilla and palatine bone shape effect changes between averaged wild-type and Pkdcc-/- E18.5 mouse embryos.
The movie progresses from the phenotype in wild-type embryos to PKDCC-/- embryos and back to wild-type embryos. Movies depict a widened distance between the symmetric palatine process of the maxilla and the horizontal plates of the palatine bone in Pkdcc+/- embryos.
Medial side view of the left mandible shape effect changes between averaged wild-type and Pkdcc+/- E18.5 mouse embryos.
The movie progresses from the phenotype in wild-type embryos to Pkdcc+/- embryos and back to wild-type embryos. Movies depict elongation in the angular process and shape variation within the mental protuberance region in Pkdcc+/- embryos.
Lateral side view of the left mandible shape effect changes between averaged wild-type and Pkdcc+/- E18.5 mouse embryos.
The movie progresses from the phenotype in wild-type embryos to Pkdcc+/- embryos and back to wild-type embryos. Movies depict elongation in the angular process and shape variation within the mental protuberance region in Pkdcc+/- embryos.
Oral view of the maxilla and palatine bone shape effect changes between averaged wild-type and Pkdcc+/- E18.5 mouse embryos.
The movie progresses from the phenotype in wild-type embryos to PKDCC+/- embryos and back to wild-type embryos. Movies depict a widened distance between the symmetric horizontal plates of the palatine bone and shape variation within the zygomatic arches in Pkdcc+/- embryos.
Top view of the maxilla and palatine bone shape effect changes between averaged wild-type and Pkdcc+/- E18.5 mouse embryos.
The movie progresses from the phenotype in wild-type embryos to PKDCC+/- embryos and back to wild-type embryos. Movies depict a widened distance between the symmetric horizontal plates of the palatine bone and shape variation within the zygomatic arches in Pkdcc+/- embryos.
Front view of the maxilla and palatine bone shape effect changes between averaged wild-type and Pkdcc+/- E18.5 mouse embryos.
The movie progresses from the phenotype in wild-type embryos to PKDCC+/- embryos and back to wild-type embryos. Movies depict a widened distance between the symmetric horizontal plates of the palatine bone and shape variation within the zygomatic arches in Pkdcc+/- embryos.
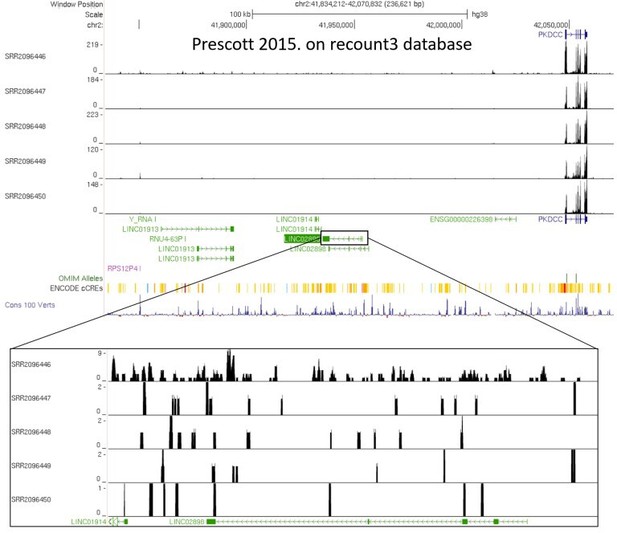
RNA-seq datasets from in vitro derived late CNCC differentiated from H9 hESCs (from Prescott et al., 2015).
Datasets were re-processed using recount3 pipeline recommended by a reviewer. Shown here are the limited number of raw reads that align to lincRNA C2orf91 (LINC02898), versus the hundreds of raw reads that align to PKDCC.