A ratchet-like apical constriction drives cell ingression during the mouse gastrulation EMT
Figures
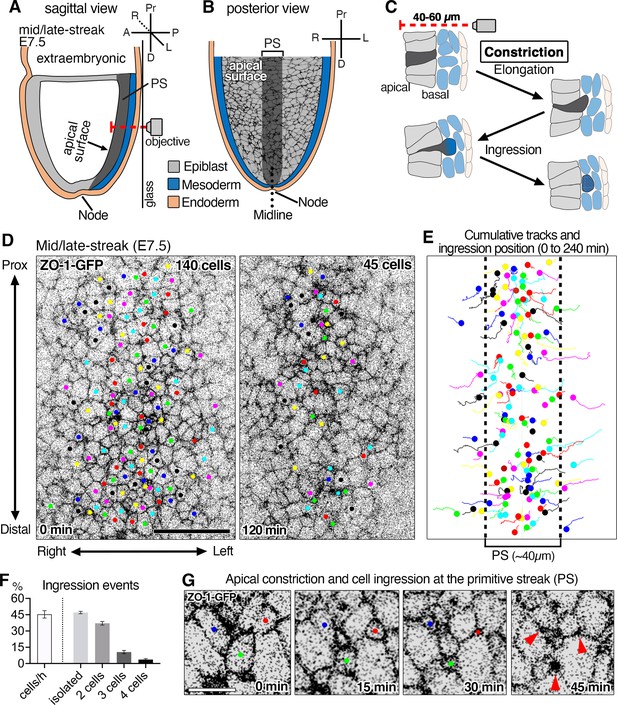
Time-lapse imaging of ZO-1-GFP reporter reveals epithelial-to-mesenchymal transition (EMT) events at the primitive streak of the mouse gastrula and apical constriction associated with cell ingression.
(A) Schematic sagittal section view of embryonic day (E)7.5 mouse embryo and 3D time-lapse imaging performed from the posterior side in glass-bottom dishes with the objective positioned adjacent to the primitive streak (PS). In this configuration, the epiblast apical surface situated furthest from the objective (red dashed line shows the microscope light path). (B) Schematic of a view (from the inner cavity) of the apical surface of cells within the epiblast layer. The midline (dotted line) separates the right and left sides of the embryo. (C) High magnification schematic of a sagittal view of an EMT event at the primitive streak depicting a cell constricting its apical surface, elongating basally (dark gray), and ingressing out of the epiblast layer to integrate into the mesoderm (blue). Note the apical surface of epiblast cells is located 40–60 µm away from the imaging objective. (D) Single time points at t=0 min and t=120 min of a time-lapse of a ZO-1-GFP embryo. Movies were analyzed as maximum intensity projections of z-stacks to visualize the apical surfaces of cells. All the cells that can be followed and observed ingressing during 4 hr were tracked. Of 140 cells initially tracked, 95 cells constricted and ingressed over the course of 120 min (45 tracked cells remaining). The time-lapse was acquired with a 5 min time interval, 290 cells were in the field of view, with 140 ingressing cells tracked. (E) Cumulative tracking of the 140 cells showing cell tracks over time (lines) and cell position at the time of ingression (dots). Epiblast cells converge toward the primitive streak (~40 µm, dotted lines) where the majority of ingression events occur. (F) Graphs showing 44 ± 2.1% of cells in the primitive streak ingress each hour, with 48 ± 1.1% ingressing as single cells, 37 ± 1.7% as pairs of cells, 11 ± 1.5% as triplets (groups of three cells), and 4% as groups of four cells (of a total of 378 ingressing cells analyzed in three embryos). (G) High magnification view showing the apical constriction of three cells at the primitive streak. Pr: proximal, D: distal, A: anterior, P: posterior, R: right, L: left, Ext Emb: extra embryonic region, PS: primitive streak. Error bars represent s.e.m. See Supplementary file 1 for n. Scale bars, D, 40µm; G, 10µm.
-
Figure 1—source data 1
Ingression events per hour and percentage of different types of events.
- https://cdn.elifesciences.org/articles/84019/elife-84019-fig1-data1-v1.xlsx
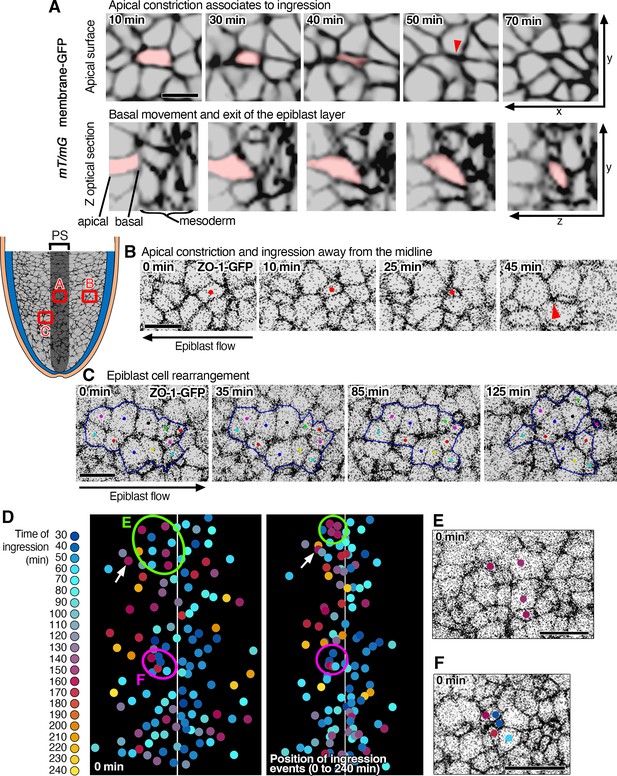
Apical constriction and ingression of epiblast cells in the vicinity of the mouse primitive streak.
(A) Snapshots of a time-lapse of membrane-GFP in an mTmG embryo showing apical surface constriction in a single Z-plane below the apical membrane (top row) associated with ingression out of the epiblast layer and integration into the mesoderm seen on an apicobasal Z optical section (bottom row) at the primitive streak (region located on the schematic). (B) Example of cell constricting away from the midline, as epiblast cells move toward the primitive streak. (C) Example showing cell rearrangement among a cluster of epiblast cells near the primitive streak. (D) Color-coded time-series and position of ingression events of tracked cells shown in Figure 1D and E. The initial position of a group of cells (green circle) is shown in E; cells are not all neighbors at the start (0 min) but ingress together as neighbors (after 165 min). Another group of cells (purple circle) whose initial position is shown in F; cells are neighbors at 0 min, but they do not ingress synchronously. Cells are considered to constrict and ingress as isolated cells when their ingression takes place 30 min or more apart from their neighbors. White arrows highlight the ingression of an isolated cell. Scale bars, A,B,C, 10 µm; E,F, 20 µm.
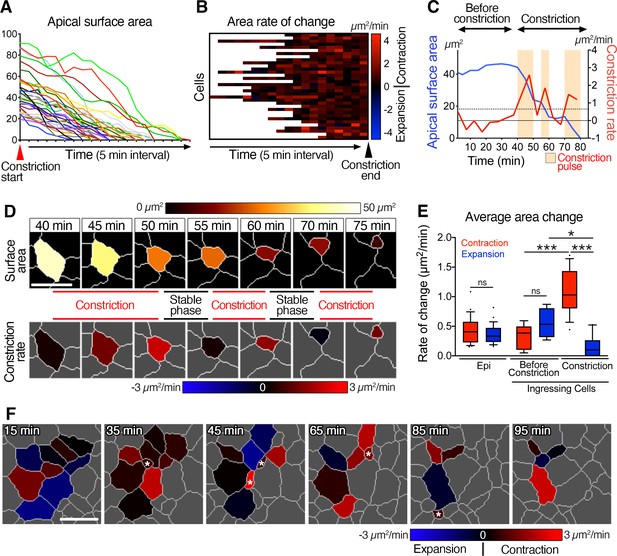
Pulsed racket-like apical constriction during epiblast cell ingression and asynchronous constriction at the multi-cellular level.
(A) Graph showing the apical surface area of a few cells during constriction preceding ingression. Data are aligned to the start of constriction. (B) Heat map showing the rate of change of apical surface area of cells during the final constriction period, revealing minimal expansion and pulsed contractions. Each row represents data for an individual cell, with all data aligned to the end of the constriction period. (C) Graph of apical surface area and rate of constriction of a single cell exhibiting a slight oscillation in its apical surface before undergoing a final constriction period with (three – beige regions) pulses of constriction. (D) Membrane segmentation and color-coded time-series of apical surface area and constriction rate of a single cell exhibiting three pulses of constriction separated by periods of stability. The highlighted cell corresponds to the plot shown in C. (E) Plot showing the average rate of changes in apical area. Epiblast cells located at a distance from the primitive streak before ingression show low and equivalent levels of contraction and expansion. Cells at the primitive streak show no significant differences between contraction and expansion prior to their period of constriction, whereas rate of contraction is significantly higher, and expansion reduces during the subsequent constriction period. (F) Color-coded time-series and heat map of rate of change of apical surface area showing asynchronous oscillation in area and asynchronous constriction and ingression among neighbors. Asterisks show ingression events. Forty-one cells quantified from four embryos. Error bars represent s.e.m. See Supplementary file 1 for n and p values. Scale bars: 10µm. *p<0.05, ***p<0.001 (unpaired bilateral Mann-Whitney test).
-
Figure 2—source data 1
Apical constriction and constriction rate.
- https://cdn.elifesciences.org/articles/84019/elife-84019-fig2-data1-v1.xlsx

Details and features of constriction pulses and cell parameters during constriction.
(A) Single time points at t=0 min of a time-lapse of a myosin heavy chain IIB-GFP embryo. Time-lapse was acquired with a 5 min time interval. Red box shows the cell highlighted in B. (B) Membrane segmentation and color-coded time-series of apical surface area of the cell boxed in A, showing apical constriction, and graph of apical surface area and rate of constriction (C). (D) Plot showing the apical surface area of several cells at the primitive streak during the total time of the analysis; 0 min marks the beginning of the constriction period. Cells begin with a range of different initial apical surface areas prior to their constriction. (E) Heat map plot of the apical surface area during constriction showing the reduction in area and the pulses of constriction. Each row represents data for an individual cell. (F) Fraction of time cells spend contracting or expanding their apical surfaces revealing equilibrium in lateral epiblast and at the streak prior to the constriction period, followed by significantly longer contraction time during constriction. (G) Plot of frequency distribution illustrating the proportion of cells undergoing two to four main constriction pulses. (H) Dot plot showing the average magnitude of constriction pulses. (I) Plot showing the apical surface area and heat map of rate of change of epiblast cells outside the region occupied by the primitive streak before ingression. Cells exhibit a wide variety of apical areas, and fluctuate their apical surfaces, with a low rate of contraction and expansion through time. Corresponding measurements are shown in F and Figure 2E. (J) Plot showing the number of cell edges during the constriction period. Cells have different initial numbers of edges which gradually decrease during the constriction period. (K) Plot showing the apical surface elongation index calculated by Tissue Analyzer, from 0 to 1, with 0 being perfectly round, and 1 corresponding to a line. Cells show fluctuations in their elongation during constriction, but exhibit no significant trend (trend depicted by linear regression). (L) Plot showing apical surface orientation in the plane of the epiblast epithelial layer. Cells exhibit fluctuations of their orientation axis during constriction, and do not show a significant pattern of elongation. Forty-one cells were quantified from four embryos. *p<0.05 (unpaired bilateral Mann-Whitney test). Error bars represent s.e.m. See Supplementary file 1 for n and p values. Scale bars, A, 40µm; B, 10µm.
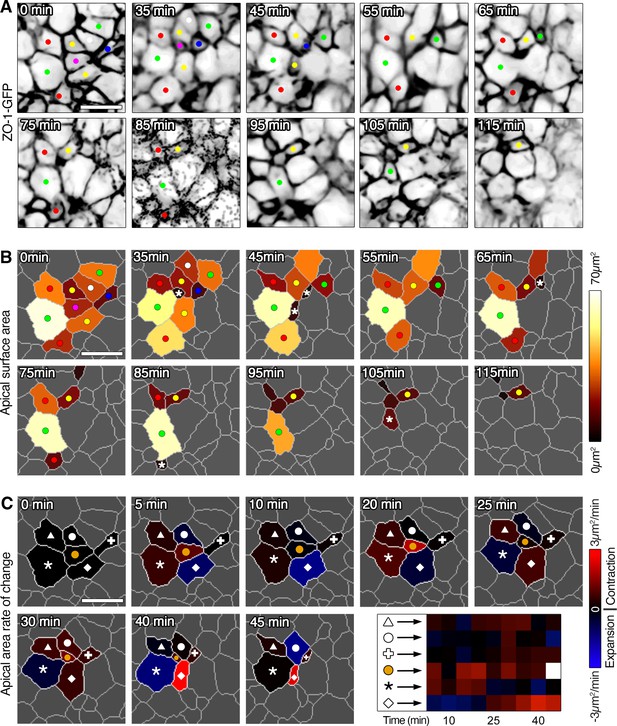
Asynchronous apical constriction at the multi-cellular level.
(A) Time-series of ZO-1-GFP reporter expression where a cluster of epiblast cells exhibit apparent stochastic apical constriction and cell ingression. (B) Membrane segmentation and color-coded time-series of apical surface area showing oscillation in apical surfaces in the cell cluster and asynchronous reduction of apical surfaces during ingression (asterisks). (C) Color-coded time-series and heat map plot of rate of change of apical surface area showing asynchronous oscillation in area and asynchronous constriction. Note that as one cell in the middle constricts and ingresses (orange dot), neighboring cells asynchronously change their apical surfaces; some will constrict and ingress shortly after, while others will only ingress at a later time point. Each row represents data for an individual cell. See Supplementary file 1 for n and p values. Scale bars, 10µm.
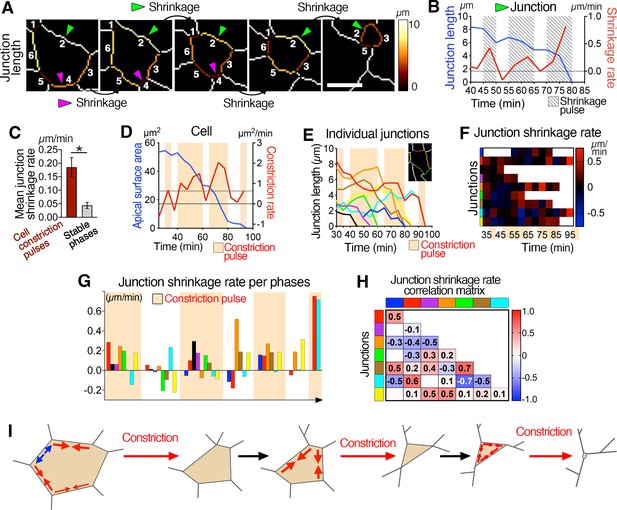
Asynchronous junctional shrinkage during apical constriction.
(A) Membrane segmentation and color-coded time-series of a single junction showing a reduction of the junction’s length during apical constriction. Here, the six initial junctions are numbered, and two are highlighted (arrowheads) and shrink at different time. (B) Graph showing the length and rate of shrinkage of the junction identified with the green arrowhead in A, indicating the three main pulses of shrinkage (hatched regions). (C) Graph showing increased rate of junctional shrinkage during the pulses of cell constriction compared to stable phases between constriction pulses from multiple cells (three embryos, 51 junctions). (D) Graph of apical surface area and rate of constriction of a single cell showing pulses of constriction, and associated graph showing the length of its individual junctions over time (E). Junctions reduce their length differentially during the constriction pulses (beige regions). (F) Heat map of the shrinkage rate of junctions, showing junctions shrinking asynchronously during the constriction pulses (beige time points). Each row represents data for an individual junction, junctions are color-coded as corresponding in E, and ordered as neighboring junctions. (G) Plot of the average shrinkage rate of each junction over time during each constriction pulse and intervening stable phases, illustrating the asynchronous shrinkage of different sets of junctions with variable magnitude during the different cell constriction pulses. Junctions are color-coded as neighbors, as in E. (H) Matrix heat map showing the correlation of junction length changes over time. Some junctions show correlation and change their length, as others show anti-correlation. Each row and column represent a single junction and are color-coded as in E. Numbers represent the correlation coefficients for each pair of junctions. (I) Model of ratchet-like pulsed constriction associated with asynchronous junctional shrinkage. Error bars represent s.e.m. *p<0.05 (unpaired bilateral Mann-Whitney test). See Supplementary file 1 for n and p values. Scale bars, 5µm.
-
Figure 3—source data 1
Junction length and shrinkage rate.
- https://cdn.elifesciences.org/articles/84019/elife-84019-fig3-data1-v1.xlsx
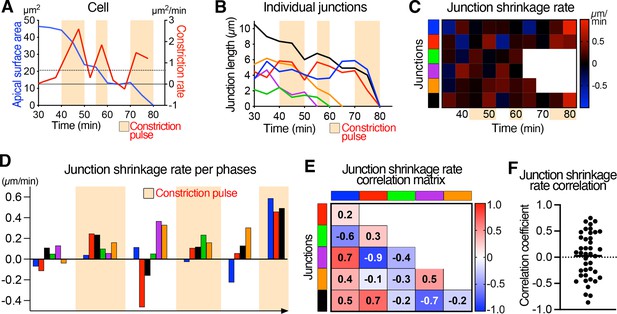
Further example illustrating the asynchronous junctional shrinkage during apical constriction.
(A) Graph of apical surface area and rate of constriction of a single cell showing pulses of constriction, and associated graph showing the length of its individual junctions over time (B). (C) Heat map of the rate of shrinkage of junctions, showing junctions shrinking asynchronously during the constriction pulses (beige time points). Each row represents data for an individual junction, with junctions color-coded as in B. (D) Plot of the average shrinkage rate of each junction over time during each constriction pulse and stables phases, showing the asynchronous shrinkage of different sets of junctions with variable magnitude during the different cell constriction pulses. Junctions are color-coded as neighbors, as in B. (E) Matrix heat map showing the correlation of junction length changes through time. Each row and column represent a single junction and are color-coded as in B. Numbers represent the correlation coefficients of each pair of junction being compared. (F) Dot plot showing the correlation coefficients of junction length changes over time of junctions from multiple cells and several embryos. See Supplementary file 1 for n and p values.
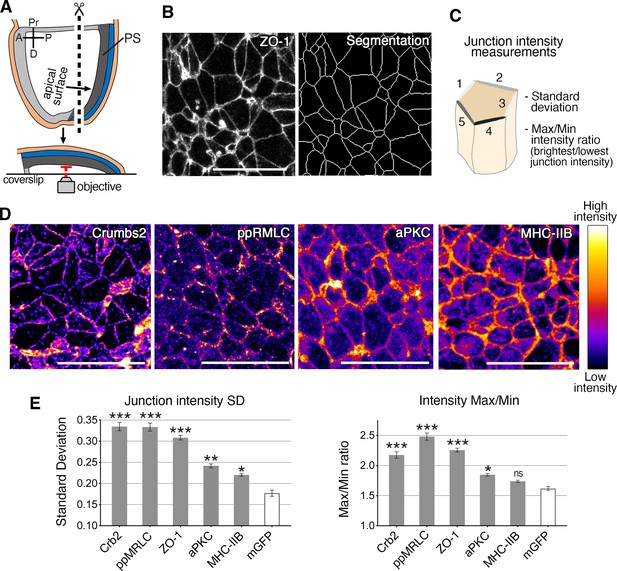
Anisotropic distribution of apical components in epiblast cells at the primitive streak.
(A) Schematic of embryo processed for fixed tissue imaging after immunostaining. Embryos are microdissected and their posterior side is mounted between a slide and coverslip so that the apical surface of the epiblast is directly adjacent to the objective for imaging. (B) ZO-1 apical membrane localization, and corresponding skeleton of membrane segmentation used to quantify junctional intensities and anisotropy parameters, as schematized. (C) The average intensities of proteins associated with the junctions of individual cells are used to calculate the standard deviation between junctions, and the difference between junctions with the maximum and minimum intensities. (D) Lookup table (LUT) of immunostaining of Crumbs2, di-phosporylated myosin regulatory light chain (ppMRLC), aPKC, and myosin heavy chain IIB. These proteins show different distributions at apical junctions. Myosin heavy chain IIB and aPKC are present on almost every junction, and have a low anisotropy distribution, whereas ppMRLC and Crumbs2, which are not present on every junction, show a more anisotropic distribution. (E) Plots showing the anisotropy parameters, standard deviation, and Max/Min. Proteins such as Crumbs2, ZO-1, and ppMRLC show elevated parameters corresponding to a high level of anisotropy, whereas aPKC and myosin heavy chain IIB show lower anisotropy. The membrane-GFP (recombined mTmG mouse reporter line) is used as a control as it exhibits a homogeneous distribution of GFP across all junctions with low anisotropy. A range of 407–4135 junctions were analyzed in three to five embryos for each protein. Statistical tests compare each protein to GFP. Pr: proximal, D: distal, R: right, L: left, PS: primitive streak. **p<0.01, ***p<0.001 (one-way ANOVA test). Error bars represent s.e.m. See Supplementary file 1 for n and p values. Scale bars, 20µm.
-
Figure 4—source data 1
Standard deviation and Max/Min ratio of the junctional intensity of the different proteins.
- https://cdn.elifesciences.org/articles/84019/elife-84019-fig4-data1-v1.xlsx
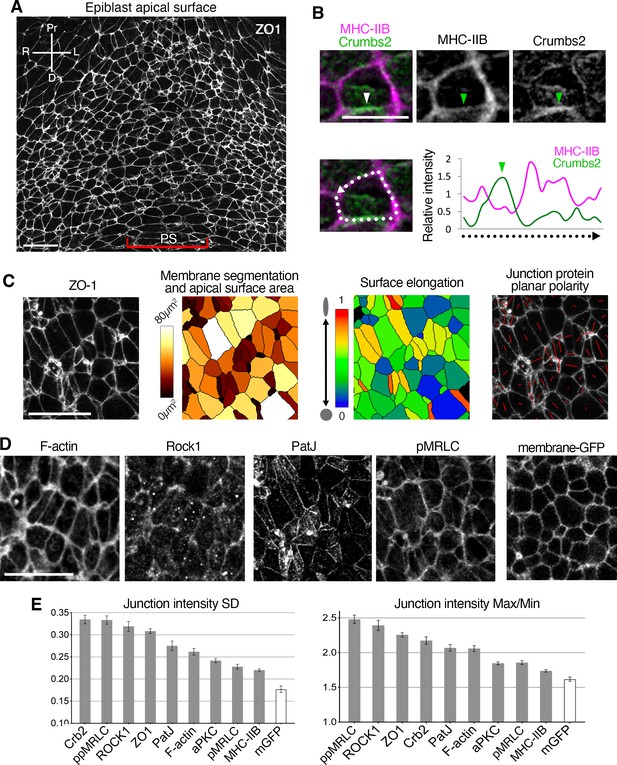
Reciprocal enrichment of Crumbs2 and myosin heavy chain IIB, and anisotropy of apical components at the primitive streak.
(A) ZO-1 immunostaining on a fixed embryonic day (E)7.5 embryo showing the apical surface of epiblast cells in the posterior. (B) High magnification view of a single cell stained for myosin heavy chain IIB (magenta) and Crumbs2 (green) showing strong Crumbs2 accumulation on one junction (arrowhead) and predominant myosin accumulation on other junctions. White dashed line and arrow indicate the membrane intensity measurement shown in the plot. The junction with the highest level of Crumbs2 (arrowhead) has the lowest level of myosin heavy chain IIB, whereas myosin heavy chain IIB predominates (and Crumbs2 is reduced) at the rest of the junctions. (C) ZO-1 immunostaining used for cell apical membrane identification and segmentation, allows quantification of junctional intensity of staining, and determination of a number of parameters, including apical surface area, surface elongation, and protein planar polarity. (D) F-actin, Rock1, PatJ, and pMRLC exhibiting different patterns of accumulation at apical cell-cell junctions, and a membrane-GFP reporter which is homogeneously distributed at the apical membrane. (E) Plots of anisotropy parameters, junctional standard deviation, and Max/Min. Proteins exhibit a range of distributions and anisotropy levels, some, such as Crumbs2 and ppMRLC, show a high level of anisotropy, while others, such as PatJ and F-actin, show moderate anisotropy, and others such as aPKC, pMRLC, and myosin heavy chain IIB show a lower level of anisotropy closer to the level of the negative control membrane-GFP. Error bars represent s.e.m. See Supplementary file 1 for n. Scale bars, A, C, D, 20µm; B, 10µm.
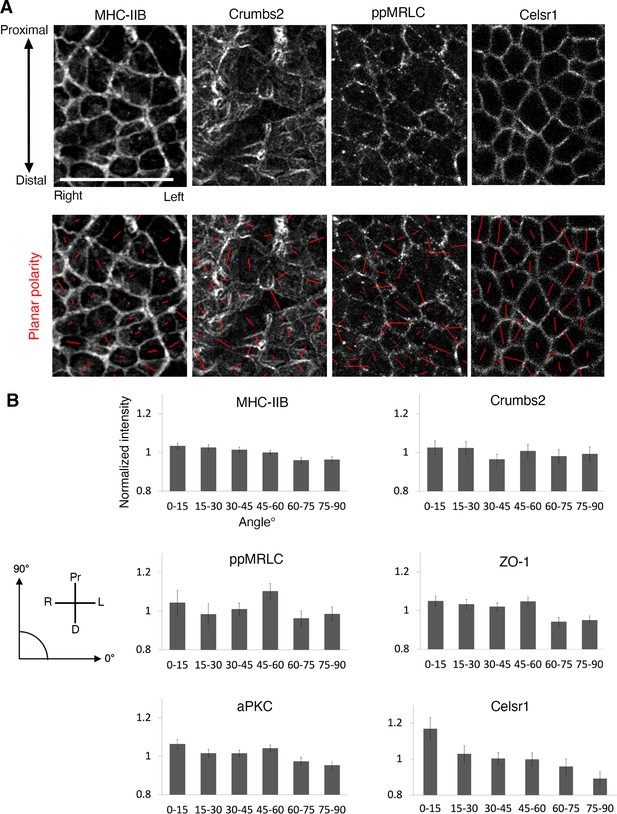
Apical proteins show anisotropy accumulation but no planar polarity.
(A) Immunostaining for myosin heavy chain IIB, Crumbs2, di-phosporylated myosin regulatory light chain (ppMRLC), and Celsr1 with associated polarity visualization (red tensor) calculated after membrane segmentation, showing the orientation (orientation of the tensor) and amplitude of polarity (length of the tensor). (B) Junctional protein intensity plotted as a function of junctional angle. 0° is parallel to the left/right axis and 90° is parallel to the proximal/distal axis. The apical proteins described previously and measured here (myosin heavy chain IIB, Crumbs2, ppMRLC, ZO-1, and aPKC) do not show planar polarity, red bars in A showing polarity of intensity are not oriented along any specific axis, and intensity in B shows no bias at a particular angle. By contrast, the planar cell polarity (PCP) protein Celsr1 exhibits polarity. Red bars tend to be vertically oriented, and intensity in B shows a significant increase in Celsr1 levels at edges oriented parallel to the left/right axis. Celsr1 preferentially accumulates at horizontal junctions (left-right embryo orientation). Error bars represent s.e.m. Scale bars, 20µm.
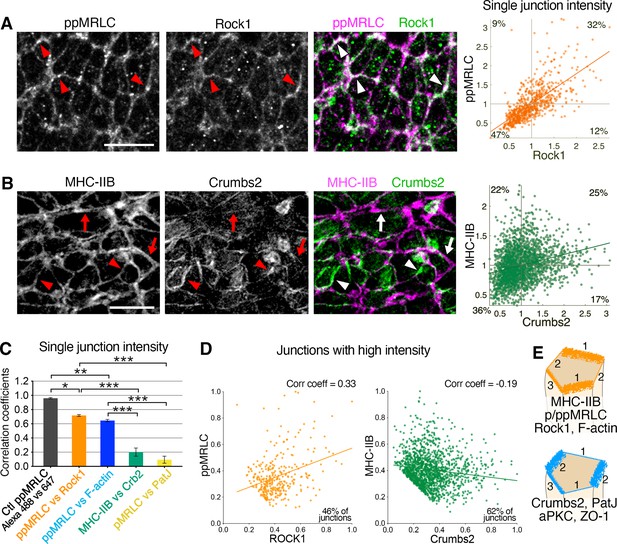
Reciprocal enrichment of apical components at apical junctions.
(A) Co-immunostaining of di-phosphorylated myosin regulatory light chain (ppMRLC) and Rock1 reveals their tendency to accumulate on the same junctions. Note colocalization of fluorescent signal on most junctions (arrowheads). Scatter plot on the right illustrating positive intensity correlation of the two proteins. Each point represents the mean intensity at a single junction. (B) Co-immunostaining of myosin heavy chain IIB and Crumbs2 reveals that they tend to accumulate on different junctions. Note junctions where myosin heavy chain IIB accumulation predominates (arrows), and junctions where Crumbs2 predominates (arrowheads). Corresponding scatter plot shows a low correlation. (C) Plot showing the correlation coefficients associated with the different scatter plots. Proteins such as ppMRLC, Rock1, and F-actin exhibit a strong correlation with a high coefficient (ppMRLC vs Rock1=0.72 ± 0.01, ppMRLC vs F-actin=0.65 ± 0.02), whereas others including myosin heavy chain IIB and Crumbs2, PatJ, and pMRLC exhibit much lower correlation with low coefficient (myosin heavy chain IIB vs Crumbs2=0.20 ± 0.06, pMRLC vs PatJ = 0.09 ± 0.05). (D) Scatter plot showing the junctional intensity correlation of ppMRLC and Rock1; and Crumbs2 and myosin heavy chain IIB where junctions with low intensity were removed from the analysis (intensities were normalized from 0 to 1). ppMRLC and Rock1 continue to show a positive correlation as in A, while myosin heavy chain IIB and Crumbs2 show anti-correlation. (E) Based on co-staining for multiple apical proteins and measurements of anisotropy and correlation, two groups of proteins can be defined with a tendency to accumulate on same junctions: actomyosin components, and apical determinants and junctional proteins. Junction 1 predominantly accumulates the myosin group, with very little accumulation of the Crumbs group. Junction 2, on the other hand, predominantly accumulates the Crumbs group with very little accumulation of the myosin group. While junction 3 shows an intermediate accumulation of both groups of proteins; 711–2453 junctions were quantified in three to four embryos for each pair of proteins. *p<0.05, ***p<0.001 (one-way ANOVA test). Error bars represent s.e.m. See Supplementary file 1 for n and p values. Scale bars, 10 µm.
-
Figure 5—source data 1
Mean junctional intensity of the different proteins.
- https://cdn.elifesciences.org/articles/84019/elife-84019-fig5-data1-v1.xlsx
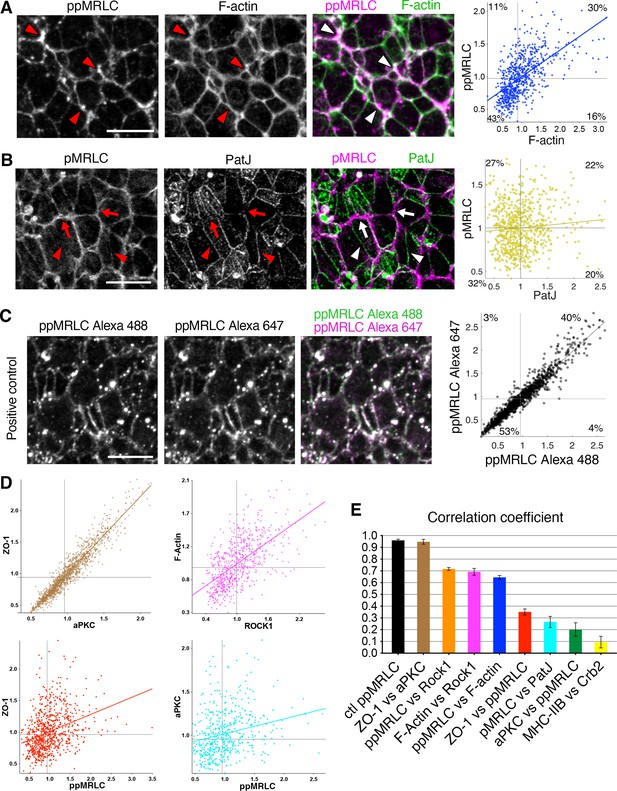
Correlation of protein distributions at apical junctions.
(A) Co-immunostaining of di-phosphorylated myosin regulatory light chain (ppMRLC) and F-actin reveals their tendency to accumulate on the same junctions. Note colocalization of fluorescent signal on most junctions (arrowheads). Scatter plot on the right showing elevated intensity correlation of the two proteins. Each point represents the mean intensity at a single junction. (B) Co-immunostaining of mono-phosphorylated myosin regulatory light chain (pMRLC) and PatJ reveals that they tend to accumulate on different junctions. Note junctions where myosin accumulation predominates (arrows), and junctions where Crumbs2 predominates (arrowheads). Corresponding scatter plot reveals a broader distribution and no correlation. (C) Immunostaining for ppMRLC simultaneously revealed by two distinct secondary antibodies (Alexa 488 and 647) as positive control, and corresponding scatter plot showing strong junctional intensity correlation after membrane segmentation. (D) Scatter plots showing the correlation of intensity of other pairs of proteins. (E) Correlation coefficients associated with scatter plots shown in A,B,C,D and Figure 5. Error bars represent s.e.m. See Supplementary file 1 for n. Scale bars, 10µm.
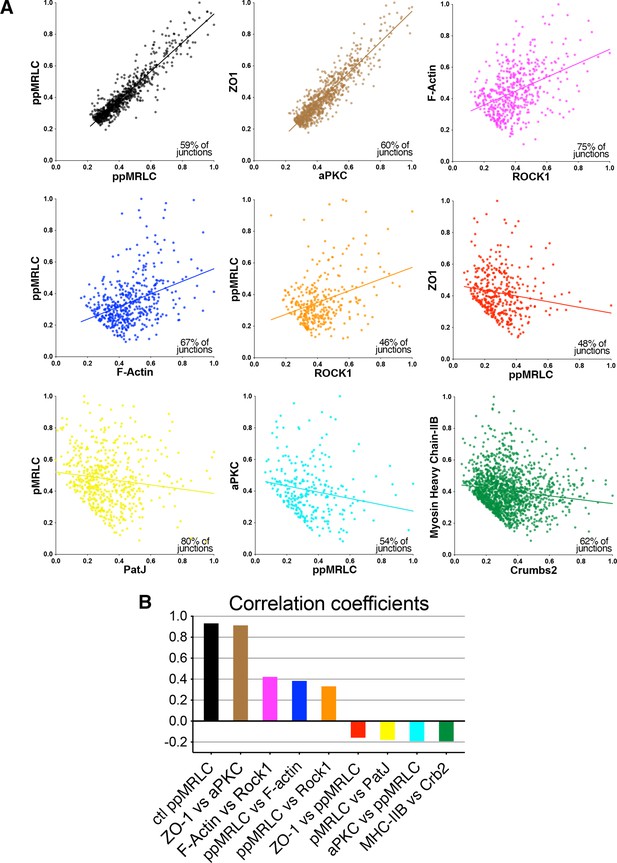
Correlation of protein distributions at junctions of high intensity.
(A) Scatter plots showing the correlation of intensity of pairs of proteins when junctions with low intensity were removed (intensities were normalized from 0 to 1), and corresponding correlation coefficients (B). Here some pairs of proteins show positive correlation, while others show anti-correlation.
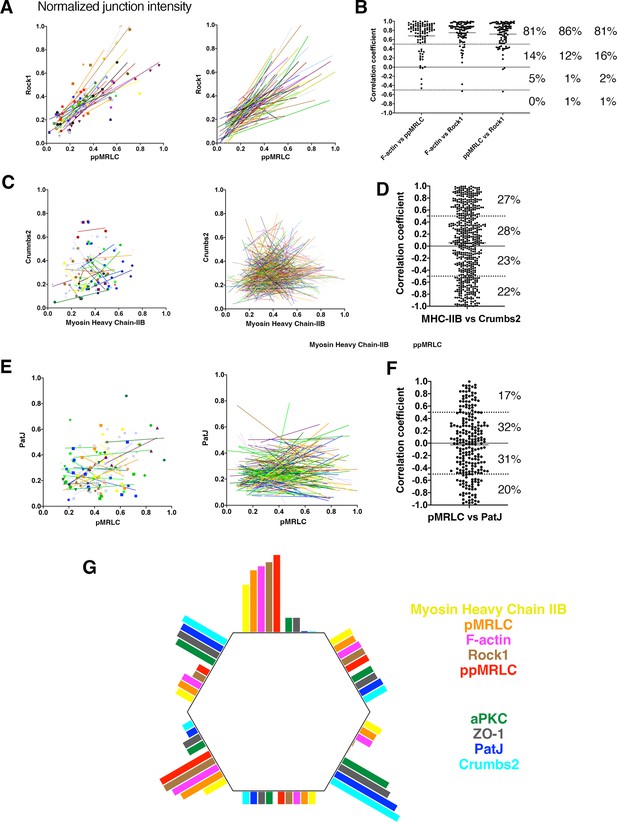
Junctional correlation of proteins at the single cell level.
(A) Example of a scatter plot showing the junctional intensity correlation of di-phosphorylated myosin regulatory light chain (ppMRLC) and Rock1 in a cohort of cells. Each symbol represents one junction, grouped by cells (color-coded) with respective correlation trends. The plot on the right shows only the correlation trend for a larger number of cells. (B) Correlation coefficients of ppMRLC and Rock1 from plot shown in A, as well as F-actin and ppMRLC, and F-actin and Rock1. Each dot represents the coefficient of correlation of junctional intensity of a single cell. % depicts the distribution of correlation coefficients in the different quarters. (C) An example of the junctional intensity correlation of single cells for Crumbs2 and myosin heavy chain IIB, depicting a mix of cells with some exhibiting correlation, some with no correlation, and some with anti-correlation. (D) Correlation coefficients reflecting the scatter plot shown in C, showing a broad distribution, from correlation (+1) to anti-correlation (–1). % depicts the distribution of correlation coefficients. (E) An example of the junctional intensity correlation of single cells for PatJ and mono-phosphorylated myosin regulatory light chain (pMRLC), depicting a mix of cells with correlation, some with no correlation, and some with anti-correlation. (F) Correlation coefficients reflecting the scatter plot shown in C, showing a broad distribution, from correlation (+1) to anti-correlation (–1). % depicts the distribution of correlation coefficients. (G) Schematic representing proteins anisotropic localization at the apical junctions and their reciprocal distribution, showing actomyosin network (myosin heavy chain IIB, p/ppMRLC, F-actin, Rock1), and Crumbs2 complex and junctional proteins (aPKC, PatJ, Crumbs2, ZO-1) accumulating preferentially on different junctions.
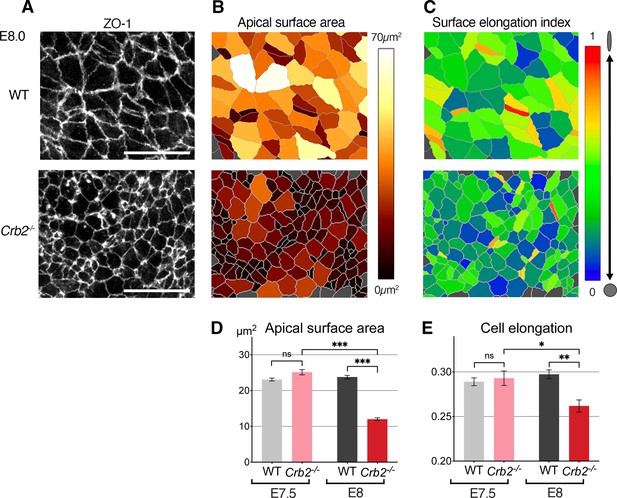
Cellular defects in Crb2 mutant embryos at the primitive streak.
(A) ZO-1 immunostaining of the apical surface of wild-type (WT) and Crb2 mutant embryos at embryonic day (E)7.5 and E8.0. (B) Segmentation and color code of apical surface areas reveals smaller surfaces in Crb2 mutants at E8.0. (C) Color code of cell surface elongation index calculated by Tissue Analyzer (regardless of their axis) reveals rounder surfaces in Crb2 mutants at E8.0. (D) Graph of quantifications showing significantly smaller apical surfaces in Crb2 mutants at E8.0 compared to E7.5, and to WT embryos. (E) Graph showing significantly less elongated apical surfaces in Crb2 mutants at E8.0 compared to E7.5, and to WT embryos; 334–1187 cells from three to five embryos were quantified for each genotype and stage. ***p<0.001 (unpaired bilateral Mann-Whitney test). Error bars represent s.e.m. See Supplementary file 1 for n and p values. Scale bars, 20 µm.
-
Figure 6—source data 1
Apical surface area and cell elongation in wild-type (WT) and Crb2 mutant embryos.
- https://cdn.elifesciences.org/articles/84019/elife-84019-fig6-data1-v1.xlsx
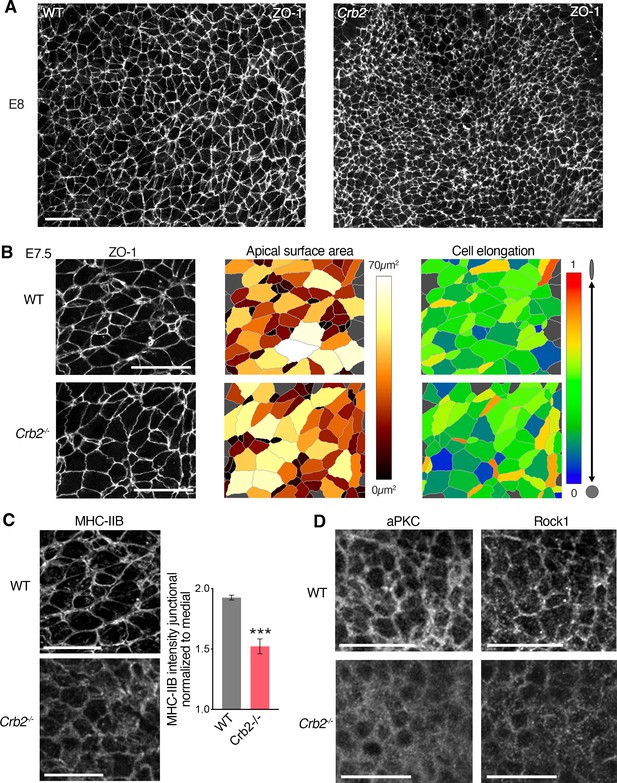
Cellular and molecular defects in Crb2 mutant embryos.
(A) Low magnification of ZO-1 localization (immunostaining) in the posterior epiblast of wild-type (WT) and Crb2 mutants at embryonic day (E)8.0. (B) ZO-1 immunostaining at the apical surface of WT and Crb2 mutant embryos at E7.5. Segmentation and color code of apical surface areas and surface elongation reveal normal size and shape of apical surface area in Crb2 mutants at E7.5. (C) Immunostaining for myosin heavy chain IIB at E7.5, showing reduced intensity in Crb2 mutant embryos compared to WT. Graph shows significantly reduced myosin heavy chain IIB intensity at junctions. (D) Immunostaining for aPKC and Rock1 in WT and Crb2 mutants at E7.5 (intensity quantifications shown in Figure 7C). ***p<0.001 (unpaired bilateral Mann-Whitney test). Error bars represent s.e.m. See Supplementary file 1 for n and p values. Scale bars, 20µm.
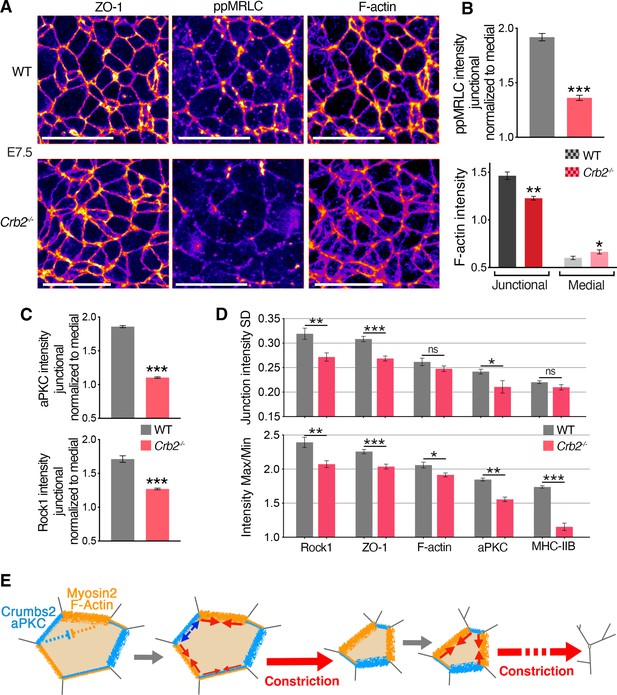
Molecular defects in Crb2 mutants preceding visible cellular defects.
(A) Co-immunostaining for ZO-1, di-phosphorylated myosin regulatory light chain (ppMRLC) and F-actin in wild-type (WT) and Crb2 mutants at embryonic day (E)7.5 reveal reduced ppMRLC and F-actin junctional intensities. (B) Plots showing intensity measurements of junctional ppMRLC (junction intensities normalized by the medial signal) and F-actin. ppMRLC shows a significant decrease in junctional accumulation in Crb2 mutants. F-actin shows lower junctional accumulation and increased diffuse medial intensity in Crb2 mutants. (C) Graphs of junctional intensity measurements normalized by the medial signal, showing significantly junctional decreased aPKC and Rock1 intensity in Crb2 mutants compared to WT. (D) Anisotropy parameters, standard deviation and Max/Min showing perturbed junctional accumulation anisotropy in Crb2 mutants compared to WT embryos. ZO-1, F-actin, myosin heavy chain IIB, aPKC, and Rock1 show reduced anisotropy in Crb2 mutants. (E) Working model. Actomyosin network and Crumbs2 complex show anisotropic and reciprocal enrichment at apical junctions of epiblast cells at the primitive streak. These distributions are likely dynamic through time and heterogenous among cells, and thus could potentially regulate each other’s distribution. Junctions asynchronously shrink and allow a ratchet-like constriction of apical surfaces, thereby facilitating cell constriction and ingression without creating increased tension across the tissue or compromising its integrity; 122–501 cells in three to four embryos were quantified for each genotype and proteins. *p<0.05, **p<0.01, ***p<0.001 (B,C: unpaired bilateral Mann-Whitney test; D: one-way ANOVA test). Error bars represent s.e.m. See Supplementary file 1 for n and p values. Scale bars, 20 µm.
-
Figure 7—source data 1
Intensity measurement of di-phosphorylated myosin regulatory light chain (ppMRLC), F-actin, aPKC, and Rock1 in wild-type (WT) and Crb2 mutant embryos.
- https://cdn.elifesciences.org/articles/84019/elife-84019-fig7-data1-v1.xlsx
Videos
Time-lapse imaging of a membrane-GFP reporter (recombined mT/mG) expressing embryonic day (E)7.5 embryo.
Apical constriction (right panel) associated with ingression, as cells move out of the epiblast epithelial layer basally to join the underlying mesoderm (left panel). Images representing single planes of optical sections and apical cell surfaces were denoised and deconvolved. The time-lapse spans 70 min with 10 min time interval between frames.
Time-lapse imaging of the posterior side of a ZO-1-GFP reporter expressing embryonic day (E)7.5 embryo in the vicinity of the primitive streak.
Tracking of individual cells reveals lateral epiblast cells converging toward the midline prior to their ingression. Cells around the midline (middle row) do not exhibit extensive movement in the plane of the epithelium. Movie of 240 min with 5 min interval between frames.
Time-lapse of ZO-1-GFP reveals dynamic cell behaviors at the primitive streak.
Rearrangement of cells in the epiblast near the primitive streak (top panel, 125 min), and cell apical constriction and ingression away from the primitive streak, as the epiblast flows (to the left side) toward the midline (bottom panel, 55 min). Five min time interval between frames.
Time-lapse of ZO-1-GFP highlighting three cells apically constricting and ingressing in the primitive streak region.
Sixty min total with 5 min time interval between frames.
Time-lapse of ZO-1-GFP highlighting a single cell at the primitive streak apically constricting.
Membrane segmentation is performed over time such that different parameters can be followed and quantified, including the apical surface area, cell elongation and orientation, and the number of junctions: 95 min total with 5 min interval between frames.
Segmentation of time-lapse showing color code of cell apical surface (left panel), constriction rate (middle panel), and junction length (right panel) of a cell shown in Figure 2: 40 min total with 5 min interval between frames.
Time-lapse of ZO-1-GFP showing a cell cluster at the primitive streak.
Most of the cells gradually constrict and ingress, and cells tracked after segmentation and color-coded for apical surface area and changes in surface area. Images were denoised and deconvolved: 115 min with 5 min interval between frames.
Additional files
-
MDAR checklist
- https://cdn.elifesciences.org/articles/84019/elife-84019-mdarchecklist1-v1.pdf
-
Supplementary file 1
Details of the n values and p values presented in the figures.
- https://cdn.elifesciences.org/articles/84019/elife-84019-supp1-v1.xlsx