Ion permeation pathway within the internal pore of P2X receptor channels
Figures
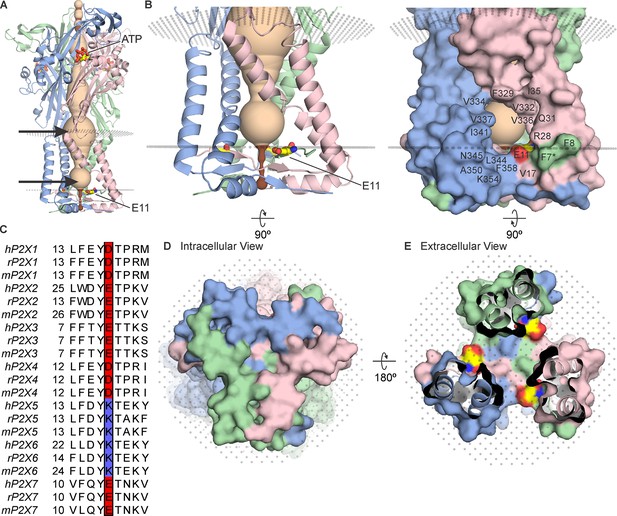
The cytoplasmic cap in P2X3 receptor channels.
(A) Side view of the structure of hP2X3Slow with ATP bound (PDB ID: 6ah4). Ribbon representations of each subunit are colored blue, pink, and green, with a HOLE representation along the central axis colored with radii ≤2 Å in brown and radii larger than 2 Å in tan. E11 (corresponding to E17 in rP2X2) is shown in stick representation with carbon (yellow), nitrogen (blue), and oxygen (red). OPM representation of the membrane is shown in light gray spheres and the location of lateral fenestrations at both extracellular and intracellular ends of the pore are indicated with arrows. (B) Magnified side view of the transmembrane helices and cytoplasmic cap shown in ribbon representation (left) and with surface representation (right). Residues around the lateral fenestrations are labeled. Asterisk at F7 indicates the side chain was modeled as Ala in the structure. (C) Multiple sequence alignment of all human, rat, and mouse P2X subtypes for the region of the cap containing E17 in rP2X2. Residues corresponding to E17 in rP2X2 are outlined with a box, with acidic residues colored red and basic residues colored blue. A full sequence alignment for elements of the cytoplasmic cap is provided in Figure 2—figure supplement 1 and Uniprot accession numbers are provided in the legend to Figure 2—figure supplement 2. (D) Intracellular view of the cytoplasmic cap with surface representation. (E) View of the cytoplasmic cap from within the pore with surface representation and carbon colored yellow, nitrogen blue, and oxygen red for atoms in E11.

Conservation of residues in the cytoplasmic cap in P2X receptor channels.
(A) Intracellular view and (B) side view of the cytoplasmic cap from hP2X3Slow in complex with ATP (PDB ID: 6ah4) using ribbon representations. (C) Intracellular view and (D) side view showing a single subunit of hP2X3Slow with side chains shown as sticks and colored according to the alignment quality score calculated from the multiple sequence alignments in Figure 2—figure supplement 1, where highly conserved residues are colored in blue and poorly conserved residues are colored in white. Alignment quality score calculated in Jalview based on BLOSUM 62 scores. Residue numbering corresponds to the hP2X3 sequence, and three mutated residues (T13P, S15V, and V16I) that slow desensitization in the construct used for structure determination are highlighted in bold. (E) Intracellular view and (F) side view of the cytoplasmic cap from hP2X3Slow showing intersubunit side chain interactions between K356 and E359 (3.2–3.8 Å apart); T12 and K357 (2.2–2.3 Å); and Q352 and E363 (2.8–3.8 Å) in yellow. Also pictured are aromatic side chains F8, F346, and F358 facing into the membrane. (G) Intracellular view of all three subunits of the cytoplasmic cap of hP2X3Slow with side chains shown as sticks and colored according to the alignment quality score calculated from the multiple sequence alignments in Figure 2—figure supplement 1. (H) View from the lateral fenestration of hP2X3Slow of two conserved tyrosine residues (Y10 and Y353) and the side chains of residues within 4.0 Å. Y10 and D340 of the neighboring subunit are 2.1–2.2 Å apart. The same orientations were used for panels A, C, E, and G and for panels B, D, and F, respectively.
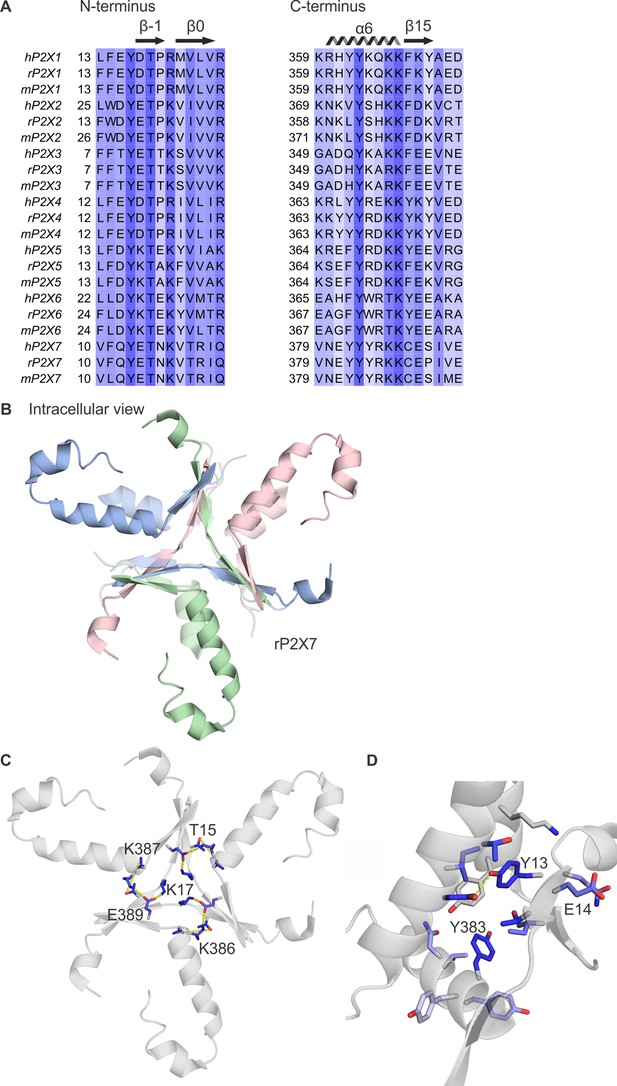
Conservation of the cytoplasmic cap in P2X receptor channels.
(A) Multiple sequence alignment of all human, rat, and mouse P2X subtypes, truncated to show residues that contribute to the cytoplasmic cap in available structures of human P2X3 and rat P2X7. Columns are colored by the alignment quality score calculated in Jalview based on BLOSUM 62 scores. (B) Intracellular view of the cytoplasmic cap from the structure of rP2X7 in complex with ATP (PDB ID: 6u9w) in the same orientation as Figure 2 panel A, with the hP2X3Slow cap overlaid for comparison. (C) Intracellular view of the cytoplasmic cap from the structure of rP2X7 in complex with ATP in the same orientation as panel B, showing intersubunit side chain interactions between K386 and E389 (3.1–3.3 Å apart); T15 and K387 (7.2–7.4 Å apart, though other rotamers would bring them close enough for hydrogen bonding similar to T12 and K357 in P2X3Slow); and K17 and E389 (2.4–2.6 Å apart) in yellow. Side chains are colored according to the alignment quality score calculated from the multiple sequence alignments in panel A, where highly conserved residues are colored in blue and poorly conserved residues are colored in white. Alignment quality score calculated in Jalview based on BLOSUM 62 scores. (D) View from the lateral fenestration of rP2X7 of two conserved tyrosine residues (Y13 and Y383) and the side chains of residues within 4.0 Å. Y13 and D352 of the neighboring subunit are 2.9–3.0 Å apart. This view is from the same orientation as Figure 2H.
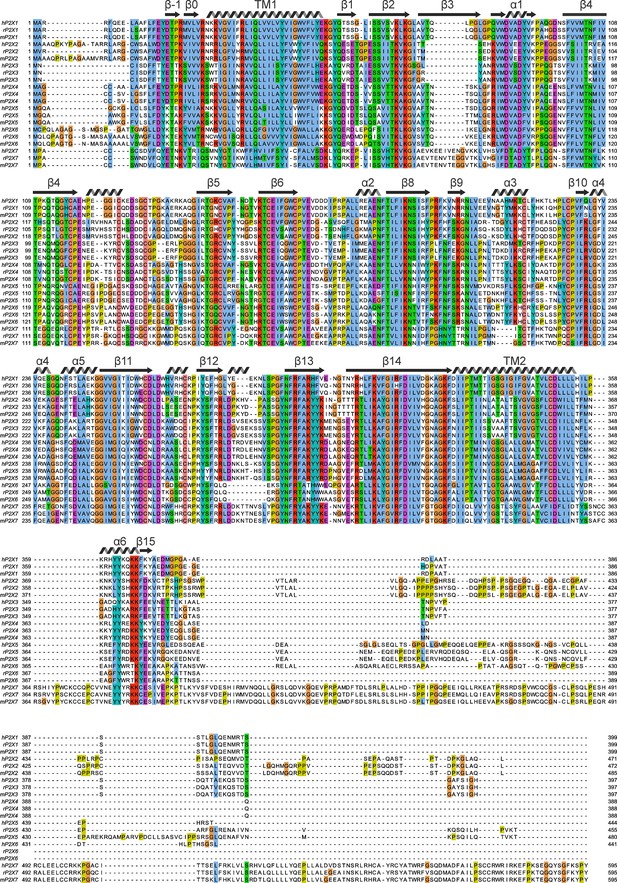
Multiple sequence alignment of human, rat, and mouse P2X receptor channels.
Multiple sequence alignments obtained using TCoffeeWS via Jalview with residues are colored using Jalview’s ClustalX color scheme, which is based on side chain character and conservation at that position of the alignment. Secondary structural elements are from hP2X3Slow. Uniprot accession numbers are as follows: hP2X1, P51575; rP2X1, P47824; mP2X1, P51576; hP2X2, Q9UBL9; rP2X2, P49653; mP2X2, Q8K3P1; hP2X3, P56373; rP2X3, P49654; mP2X3, Q3UR32; hP2X4, Q99571; mP2X4, Q9JJX6; hP2X5, Q93086; rP2X5, P51578; mP2X5, B7ZND5; hP2X6, O15547; rP2X6, P51579; mP2X6, O54803; hP2X7, Q99572; rP2X7, Q64663; mP2X7, Q9Z1M0.
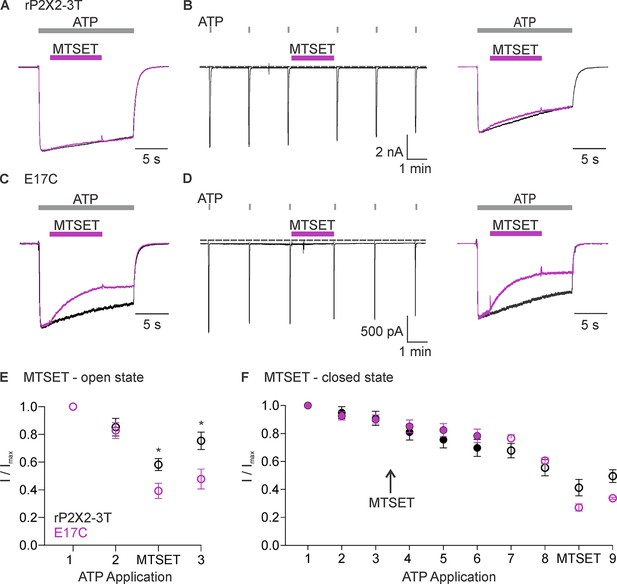
Accessibility of E17C in rP2X2–3 T to extracellular 2-trimethylaminoethyl methanethiosulfonate (MTSET).
(A) Testing for modification of rP2X2–3 T by extracellular MTSET after opening channels with extracellular ATP. Consecutive current traces elicited by extracellular ATP application at -60 mV without (black trace) or with subsequent application of 1 mM extracellular MTSET (purple trace). ATP (1 µM) was applied for 15 s at 2 min intervals three times with MTSET application only during the second ATP application. Superimposed and scaled current traces are shown for the first and second applications of ATP. MTSET (1 mM) inhibited ATP-activated currents by 26 ± 6% (n=4). (B) Testing for modification of rP2X2–3 T by extracellular MTSET when channels are closed. ATP (1 µM) was applied six times for 2 s at 2 min intervals and MTSET (1 mM) was applied in the absence of ATP after the third ATP application. Holding voltage was -60 mV. Following the application of MTSET in the closed state, MTSET was applied again in the presence of ATP to the same cell to serve as a control, using the same protocol described in A. MTSET (1 mM) inhibited ATP-activated currents by 14 ± 4% (n=4) when applied in the closed state and by 26 ± 3% (n=3) when subsequently applied in the presence of ATP. (C) Testing for modification of E17C rP2X2–3 T by extracellular MTSET after opening channels with extracellular ATP. Consecutive current traces elicited by extracellular ATP application at -60 mV without (black trace) or with subsequent application of 1 mM extracellular MTSET (purple trace). Same protocol as in A. MTSET (1 mM) inhibited ATP-activated currents by 53 ± 5% (n=5). (D) Testing for modification of E17C rP2X2–3 T by extracellular MTSET when channels are closed. Same protocol as in B. MTSET (1 mM) inhibited ATP-activated currents by 4 ± 2% (n=7) when applied in the closed state and by 55 ± 5% (n=3) when subsequently applied in the presence of ATP. (E) Normalized ATP current amplitudes for MTSET application in the presence of ATP for rP2X2–3 T (black symbols; n=3) and E17C rP2X2–3 T (purple symbols; n=5) using the protocols illustrated in panels A and C. ATP application one corresponds to the peak current amplitude for the first control ATP application within 1 s of applying ATP, two corresponds to the peak current amplitude immediately before MTS application for the second ATP application, measured within 1 s of ATP application. The ATP application labeled as MTSET corresponds to the current amplitude 10 s into the second ATP application at the end of the methanethiosulfonate (MTS) application. Three corresponds to the peak current amplitude during the third ATP application measured within 1 s of applying ATP. (F) Normalized ATP current amplitudes during MTSET application in the absence of ATP. Filled black circles (n=3) are from experiments with rP2X2–3 T and filled purple circles (n=5) are from experiments with E17C rP2X2–3 T using the protocols illustrated in panels B and D. MTSET was applied in the absence of ATP between ATP applications three and four as indicated. Open symbols are ATP current amplitudes when testing for open-state modification by MTSET after testing for closed-state modification. Open black circles (n=3) for rP2X2–3 T and open purple circles (n=3) for E17C rP2X2–3 T using the protocols illustrated on the right in panels B and D. For open state modification following closed state modification, application seven is a control ATP application, and MTSET was applied during application 8 along with ATP. Data point eight is the initial peak current immediately before MTSET was applied and the subsequent data point is at the end of the MTSET application in the presence of ATP after the reagent has had time to react, as illustrated in the right panel of B and D. Data shown in E and F are mean ± SEM, some error bars are smaller than symbols. *p<0.05 by unpaired t-test.
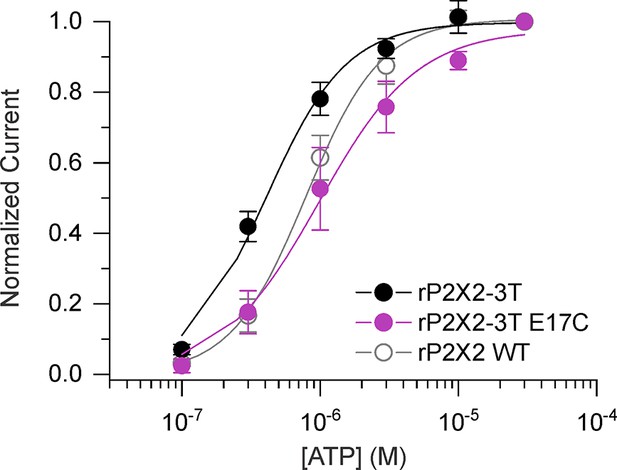
Concentration-dependence for activation of rP2X2 constructs.
Normalized concentration-dependence for ATP activation of rP2X2–3 T (black filled circles, n=7), E17C rP2X2–3 T (purple filled circles, n=3), and WT rP2X2 (gray open circles, n=4) in solutions without divalent ions with a holding voltage of -60 mV. Smooth curves are fits of the Hill equation to the data with EC50 and nH values of 0.4 ± 0.1 µM and 1.5 ± 0.2 for rP2X2–3 T, 1.0 ± 0.1 µM and 1.2 ± 0.2 for E17C rP2X2–3 T and 0.8 ± 0.1 µM and 1.6 ± 0.1 for WT rP2X2. Error bars are SEM.
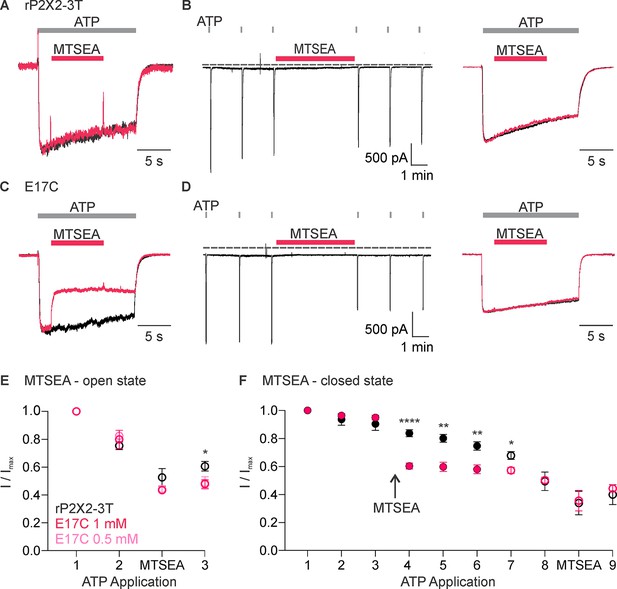
Accessibility of E17C in rP2X2–3 T to extracellular 2-aminoethyl methanethiosulfonate (MTSEA).
(A) Testing for modification of rP2X2–3 T by extracellular MTSEA after opening channels with extracellular ATP. Consecutive current traces elicited by extracellular ATP application at -60 mV without (black trace) or with subsequent application of 1 mM extracellular MTSEA (red trace). ATP (1 µM) was applied for 15 s at 2 min intervals three times with MTSEA application only during the second ATP application. Superimposed and scaled current traces are shown for the first and second applications. MTSEA (1 mM) inhibited ATP-activated currents by 28 ± 6% (n=5). (B) Testing for modification of rP2X2–3 T by extracellular MTSEA when channels are closed. ATP (1 µM) was applied six times for 2 s at 2 min intervals and MTSEA (1 mM) was applied in the absence of ATP after the third ATP application. Holding voltage was -60 mV. Following the application of MTSEA in the closed state, MTSEA was applied again in the presence of ATP to the same cell to serve as a control. MTSEA (1 mM) inhibited ATP-activated currents by 7 ± 2% (n=7) when applied in the closed state and by 34 ± 9% (n=3) when subsequently applied in the presence of ATP. (C) Testing for modification of E17C rP2X2–3 T by extracellular MTSEA after opening channels with extracellular ATP. Current traces elicited by extracellular ATP application at -60 mV without (black trace) or with subsequent application of 1 mM extracellular MTSEA (red trace). Same protocol as in A. This protocol was conducted in MTSEA concentrations of both 0.5 mM and 1 mM MTSEA. MTSEA (0.5 mM) inhibited ATP-activated currents by 46 ± 2% (n=4) and at 1 mM inhibited ATP-activated currents by 45 ± 4% (n=4). (D) Testing for modification of E17C rP2X2–3 T by extracellular MTSEA when channels are closed. Same protocol as in B. MTSEA (1 mM) inhibited ATP-activated currents by 31 ± 4% (n=6). when applied in the closed state and by 30 ± 13% (n=3) when subsequently applied in the presence of ATP. (E) Normalized ATP current amplitudes for MTSEA application in the presence of ATP for rP2X2–3 T (black symbols; n=5) and E17C rP2X2–3 T with 1 mM MTSEA (red symbols; n=4) or 0.5 mM MTSEA (pink symbols; n=4) using the protocols illustrated in panels A and C. ATP application one corresponds to the peak current amplitude for the first control ATP application, two corresponds to the peak current amplitude immediately before methanethiosulfonate (MTS) application for the second ATP application, measured within 1 s of ATP application. The ATP application labeled MTSEA corresponds to the current amplitude 10 s into the ATP application and at the end of the MTSEA application during the second ATP application. Three corresponds to peak current amplitude 2 min after MTS application during the third ATP application, measured within 1 s of ATP application. Statistical significance of *p<0.05 by unpaired t-test for 1 mM MTSEA E17C rP2X2–3 T and rP2X2–3 T background construct. (F) Normalized ATP current amplitudes during MTSEA application in the absence of ATP. Filled black circles (n=6) are from experiments with rP2X2–3 T and filled red circles (n=4) are from experiments with E17C rP2X2–3 T using the protocols illustrated in panels B and D. MTSEA was applied in the absence of ATP between ATP applications three and four as indicated. Open symbols are ATP current amplitudes when testing for open-state modification by MTSEA after testing for closed state modification. Open black circles (n=3) for rP2X2–3 T and open red circles (n=3) for E17C rP2X2–3 T using the protocols illustrated in panels B and D. For open state modification following closed state modification, application seven is a control ATP application, and MTSEA was applied during application eight along with ATP. Data point eight is the initial peak current before MTSEA was applied and the subsequent data point is at the end of the MTSEA application in the presence of ATP after the reagent has had time to react, as illustrated in the right panel of B and D. Data shown in E and F are mean ± SEM, some error bars are smaller than symbols. *p<0.05, **p<0.01, and ****p<0.0001 by unpaired t-test.

Rates of methanethiosulfonate (MTS) modification in rP2X2 for E17C and comparison with rates for the pore-lining TM2 helix.
(A) Fit of a single exponential function (purple relation) to the time course of current inhibition by 2-trimethylaminoethyl methanethiosulfonate (MTSET) (1 mM) for E17C rP2X2–3 T in the presence of ATP (1 µM) at -60 mV. (B) Fit of a single exponential function (red relation) to the time course of current inhibition by 2-aminoethyl methanethiosulfonate (MTSEA) (0.5 mM) for E17C rP2X2–3 T in the presence of ATP (1 µM) at -60 mV. (C) Side view of the transmembrane helices and cytoplasmic cap from the structure of hP2X3Slow with ATP bound (PDB ID: 6ah4). MTS-accessible residues along TM2 are labeled and each residue alpha carbon is represented as a gray sphere. (D) Comparison of MTS modification rates measured in the presence of ATP for E17C rP2X2–3 T (purple open circles for MTSET and red open circles for MTSEA) with MTSET reactive residues in TM2 (gray open circles) from Li et al., 2008. Data is mean ± SEM with n=5 for MTSET (1 mM) and n=4 for MTSEA (0.5 mM). Some error bars are smaller than symbols.

Ion selectivity of rP2X2 and influence of the E17K mutation.
(A) Representative I-V relationships for WT rP2X2 obtained from the same cell using 0.5 s voltage ramps from –60 mV to +60 mV from a holding potential of –60 mV in symmetric 140 mM NaCl solution (black) and two asymmetric NaCl solutions (internal: 140 mM NaCl; external:40 mM NaCl with glucose in green, or 40 mM NaCl with sucrose in blue). Voltage ramps were applied in the absence and presence of 30 µM ATP in each of the three external solutions and currents from ramps recorded in the absence of ATP were subtracted from currents in the presence of ATP to obtain the ATP-activated currents shown. (B) Representative current traces from a cell expressing WT rP2X2 elicited using voltage step protocols in symmetrical 140 mM NaCl solution and two asymmetrical solutions as in A. Voltage steps were elicited from a holding potential of 0 mV to –60 mV for 100 ms before stepping to a series of voltages in increments of 5 mV. The range of voltages tested for symmetrical solutions was –20 mV to +20 mV and for asymmetrical solutions was –40 mV to 0 mV. ATP-activated currents were obtained by subtracting control currents recorded in the absence of ATP from currents collected in the presence of 30 µM ATP. The gray dotted line represents 0 pA. (C) Normalized instantaneous I-V relationships for WT rP2X2 were obtained using voltage step protocols illustrated in panel B in symmetrical 140 mM NaCl and two asymmetrical solutions. Instantaneous current was measured at 0.5–1 ms following the step from –60 mV to the range of voltages indicated. Data points represent the mean steady-state current ± SEM (n=6 for each condition). (D) Representative I-V relationships for the E17K mutant of rP2X2 obtained from the same cell in symmetrical 140 mM NaCl and two asymmetric solutions using the voltage ramp protocol from panel A. (E) Representative current traces from a cell expressing the E17K mutant of rP2X2 elicited using the same voltage step protocols from panel B in symmetrical 140 mM NaCl and two external solutions containing 40 mM NaCl. (F) Normalized instantaneous I-V relationships for the E17K mutant of rP2X2 were obtained using voltage step protocols illustrated in panel E in symmetrical 140 mM NaCl and two external solutions containing 40 mM NaCl. Protocols were identical to those in C and data points represent the mean steady-state current ± SEM (n=7). (G) Reversal potential measurements for WT and E17K rP2X2 in symmetric and asymmetric NaCl solutions using either ramp or step protocols. Mean and SEM are indicated with bars and individual measurements are indicated with filled-colored circles using the same color code as in A. WT rP2X2 ramp protocol reversal potentials were –0.7 ± 0.4 mV in symmetric solution, –31.6 ± 0.7 mV in asymmetric solution with sucrose, and –32.8 ± 0.4 mV in asymmetric solution with glucose. E17K rP2X2 ramp protocol reversal potentials were 0 ± 0.4 mV in symmetric solution, –21.1 ± 1.0 mV in asymmetric solution with sucrose, and –21.9 ± 1.2 mV in asymmetric solution with glucose. WT rP2X2 step protocol reversal potentials were –0.2 ± 0.6 mV in symmetric solution, –30.7 ± 0.5 mV in asymmetric solution with sucrose, and –31.2 ± 0.8 mV in asymmetric solution with glucose. E17K rP2X2 step protocol reversal potentials were –0.4 ± 0.4 mV in symmetric solution, –23.3 ± 1.3 mV in asymmetric solution with sucrose, and –23.5 ± 1.5 mV in asymmetric solution with glucose. For WT, ramp protocols n=6 and step protocols n=5. For E17K, ramp protocols n=5 and step protocols n=7. (H) Normalized concentration-dependence for ATP activation of WT rP2X2 (open circles and dashed line, n=4) and the E17K mutant of rP2X2 (black circles, n=5). Smooth curves are fits of the Hill equation to the data with EC50 and nH values of 0.8 ± 0.1 µM and 1.7 ± 0.1 for WT and 0.9 ± 0.1 µM and 1.6 ± 0.1 for E17K.
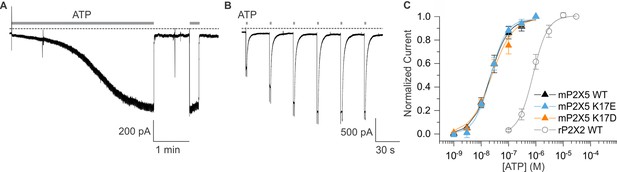
Expression and characterization of mP2X5.
(A) mP2X5 sensitization upon initial exposure to a sustained external application of ATP (3 µM). Subsequent short ATP applications activate slowly desensitizing currents of similar amplitude compared to that achieved during sensitization. Holding voltage was –60 mV. Gray dotted line represents zero current. (B) Sensitization was observed while delivering short pulses of saturating ATP (100 µM) given in 30 s intervals. Holding voltage was –60 mV. Gray dotted line represents zero current. (C) Concentration-dependence for ATP activation of WT mP2X5 (black filled triangles, n=3), the K17E mutant of mP2X5 (light blue triangles, n=4), and the K17D mutant of mP2X5 (orange triangles, n=3). Smooth curves are fits of the Hill equation to the data with EC50 and nH values of 22.5 ± 1.6 nM and 1.3 ± 0.1 for WT, 21.9 ± 1.7 nM and 1.5 ± 0.1 for K17E, and 24.1 ± 4.3 nM and 1.1 ± 0.2 for K17D. Data for WT rP2X2 are shown for comparison from Figure 6H.
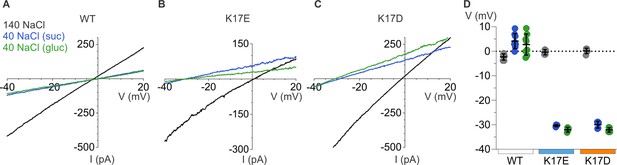
Ion selectivity of mP2X5 and influence of K17E and K17D mutations.
(A) Representative I-V relationships for WT mP2X5 obtained from the same cell using 0.5 s voltage ramps from –60 mV to +60 mV from a holding potential of –60 mV in symmetric 140 mM NaCl solution (black) and two asymmetric NaCl solutions (internal: 140 mM NaCl; external:40 mM NaCl with glucose in green, or 40 mM NaCl with sucrose in blue). Voltage ramps were applied in the absence and presence of 3 µM ATP in each of the three conditions and currents from ramps recorded in the absence of ATP were subtracted from currents in the presence of ATP to obtain the ATP-activated currents shown. (B) Representative I-V relationships for the K17E mutant of mP2X5 were obtained from the same cell using conditions as in A. (C) Representative I-V relationships for the K17D mutant of mP2X5 were obtained from the same cell using conditions as in A and B. (D) Reversal potential measurements for WT, K17E, and K17D mP2X5 in symmetrical and asymmetrical NaCl solutions using ramp protocols. Mean and SEM are indicated with bars and individual measurements are indicated with filled-colored circles using the same color code as in A. WT mP2X5 reversal potentials were –2.3±0.4 mV in symmetric solution, 4.1 ± 1.1 mV in asymmetric solution containing sucrose, and 2.8 ± 1.5 mV in asymmetric solution containing glucose. K17E mP2X5 reversal potentials were –0.4 ± 0.5 mV in symmetric solution, –30.4 ± 0.2 mV in asymmetric solution containing sucrose, and –32 ± 0.5 mV in asymmetric solution containing glucose. K17D mP2X5 reversal potentials were 0.2 ± 0.5 mV in symmetric solution, –30 ± 0.6 mV in asymmetric solution containing sucrose, and –32.1 ± 0.4 mV in asymmetric solution containing glucose. n=8 for WT, n=4 for K17E, and n=5 for K17D.