YAP/TAZ enhances P-body formation to promote tumorigenesis
eLife assessment
This valuable study advances our understanding that YAP/TAZ, as well as their target genes, plays a prominent role in the formation of processing bodies (P-bodies). The evidence supporting the conclusions is convincing. The article could be improved through further analysis to elucidate the mechanistic link between P-body formation and oncogenesis. The work will be of broad interest to scientists working in the field of Hippo signaling and cancer biology.
https://doi.org/10.7554/eLife.88573.3.sa0Valuable: Findings that have theoretical or practical implications for a subfield
- Landmark
- Fundamental
- Important
- Valuable
- Useful
Convincing: Appropriate and validated methodology in line with current state-of-the-art
- Exceptional
- Compelling
- Convincing
- Solid
- Incomplete
- Inadequate
During the peer-review process the editor and reviewers write an eLife Assessment that summarises the significance of the findings reported in the article (on a scale ranging from landmark to useful) and the strength of the evidence (on a scale ranging from exceptional to inadequate). Learn more about eLife Assessments
Abstract
The role of processing bodies (P-bodies) in tumorigenesis and tumor progression is not well understood. Here, we showed that the oncogenes YAP/TAZ promote P-body formation in a series of cancer cell lines. Mechanistically, both transcriptional activation of the P-body-related genes SAMD4A, AJUBA, and WTIP and transcriptional suppression of the tumor suppressor gene PNRC1 are involved in enhancing the effects of YAP/TAZ on P-body formation in colorectal cancer (CRC) cells. By reexpression of PNRC1 or knockdown of P-body core genes (DDX6, DCP1A, and LSM14A), we determined that disruption of P-bodies attenuates cell proliferation, cell migration, and tumor growth induced by overexpression of YAP5SA in CRC. Analysis of a pancancer CRISPR screen database (DepMap) revealed co-dependencies between YAP/TEAD and the P-body core genes and correlations between the mRNA levels of SAMD4A, AJUBA, WTIP, PNRC1, and YAP target genes. Our study suggests that the P-body is a new downstream effector of YAP/TAZ, which implies that reexpression of PNRC1 or disruption of P-bodies is a potential therapeutic strategy for tumors with active YAP.
Introduction
The Hippo pathway is an evolutionally conserved signaling pathway that regulates organ size and plays vital roles in development and tissue homeostasis (Driskill and Pan, 2021; Ma et al., 2019; Russell and Camargo, 2022). The transcriptional output of the Hippo pathway is mainly mediated by the YAP/TAZ-TEAD transcription complex. In response to various extracellular or intracellular signals, including cell–cell contact, mechanical force, serum stimulation, cellular stress, and cellular energy status, the YAP/TAZ-TEAD complex modulates target gene expression to respond to environmental cues (Calvo et al., 2013; Misra and Irvine, 2018; Yu et al., 2012; Zhao et al., 2007). Although initially identified as transcriptional coactivators, YAP/TAZ can also function as corepressors to inhibit target gene transcription by recruiting the nucleosome remodeling and histone deacetylase (NuRD) complex (Kim et al., 2015b). The evidence of Hippo pathway dysregulation in a variety of cancers and the list of YAP/TAZ target genes continue to increase (Calses et al., 2019; Kulkarni et al., 2020; Nguyen and Yi, 2019; Wang et al., 2018; Zanconato et al., 2016). Dysregulation of YAP/TAZ-TEAD transcriptional output endows tumor cells with every hallmark of cancer, including sustained proliferation, resistance to apoptosis, tumor-promoting inflammation, tumor immune escape, dysregulated tumor metabolism, etc. (Calses et al., 2019; Hanahan, 2022; Kulkarni et al., 2020; Nguyen and Yi, 2019; Zanconato et al., 2016).
At the cellular organization level, the YAP/TAZ-TEAD transcription complex modulates mitochondrial fusion; cytoskeleton, primary cilium, and focal adhesion assembly; and caveolae formation (Kim et al., 2015a; Mason et al., 2019; Nagaraj et al., 2012; Qiao et al., 2017; Rausch et al., 2019). Processing bodies (P-bodies) are cytoplasmic membraneless organelles that consist of ribonucleoprotein complexes (RNPs) and are formed by phase separation (Luo et al., 2018; Riggs et al., 2020). Although initial studies hypothesized that mRNAs in P-bodies are targeted for decay and translational repression, it was subsequently suggested that P-bodies are not required for mRNA decay and that repressed mRNAs can be recycled from P-bodies to reenter translation; thus, the primary function of P-bodies is controlling the storage of untranslated mRNAs (Decker and Parker, 2012; Hubstenberger et al., 2017; Luo et al., 2018). The role of P-bodies in tumorigenesis and tumor progression is not well studied (Anderson et al., 2015; Lavalée et al., 2021; Riggs et al., 2020). The formation of P-bodies is correlated with epithelial–mesenchymal transition (EMT) in breast cancer (Hardy et al., 2017). In contrast, there is also evidence that an increase in P-bodies leads to attenuated growth, migration, and invasion of prostate cancer cells (Bearss et al., 2021). Recently, YAP was reported to be a negative regulator of P-bodies and to be involved in Kaposi sarcoma-associated herpesvirus (KHSV)-induced P-body disassembly in human umbilical vein endothelial cells (HUVECs) (Castle et al., 2021). However, this regulatory axis and the potential function of P-bodies in YAP-induced tumorigenesis remain unclear.
In this study, we discovered that YAP/TAZ are enhancers but not negative regulators of P-body formation in a series of cancer cell lines. YAP/TAZ modulates the transcription of multiple P-body-related genes, especially repressing the transcription of the tumor suppressor proline-rich nuclear receptor coactivator 1 (PNRC1) through cooperation with the NuRD complex. As a direct YAP/TAZ target gene, PNRC1 functions as a critical effector in YAP-induced biogenesis of P-bodies and tumorigenesis in colorectal cancer (CRC). Furthermore, disruption of P-bodies by knockdown of core component genes of P-bodies attenuated the protumorigenic effects of YAP in CRC. Thus, our study reveals a YAP–P-body positive regulatory axis in CRC, which exposes the vital role of YAP/TAZ in the biogenesis of P-bodies in tumors and implies that reexpression of PNRC1 or disruption of P-bodies is a potential therapeutic strategy for cancers with active YAP.
Results
YAP/TAZ regulates the transcription of P-body-related genes
Previously, to identify the new target genes and molecular signatures of YAP/TAZ in CRC, we performed RNA sequencing analysis of HCT116 CRC cells with simultaneous knockdown of YAP and TAZ (GSE176475) (Guo et al., 2022). Gene Ontology enrichment analysis of the 674 differentially expressed genes upon knockdown of YAP/TAZ (fold change [FC] > 2, p<0.05) revealed that the genes downregulated by YAP/TAZ knockdown were enriched in the term P-body in the cellular component category (Figure 1A). We further expanded our analysis to the moderately differentially expressed genes (FC < 0.66 or >1.5) that were annotated as related to P-bodies (Figure 1—figure supplement 1A, Supplementary file 1). Through integration with the public ChIP-seq data for TEAD4 in HCT116 cells from the ENCODE database, AJUBA, WTIP, NOCT, SAMD4A, and PNRC1 were selected for in-depth investigation (Figure 1B, Figure 1—figure supplement 1A). Intriguingly, the public TEAD4 ChIP-seq datasets for the other three cancer cell lines (A549, MCF7, and MDA-MB-231), not just HCT116 cells, also showed strong TEAD4 binding peaks in the genomic loci of these five P-body-related genes (Figure 1—figure supplement 1B; Mei et al., 2017). HCT116, A549, MCF7, and MDA-MB-231 are well-established cell models for exploring YAP/TAZ function and the cell proliferation of these four cell lines is dependent on YAP/TAZ activity (Rosenbluh et al., 2012; Shreberk-Shaked et al., 2020; Zanconato et al., 2015; Zhu et al., 2019). It is worth noting that cell contact inhibition was observed in HCT116 and MDA-MB-231 and YAP remains in the nucleus regardless of cell–cell contact in A549 and MCF7 cells (Fan et al., 2013; Kim et al., 2011; Lee et al., 2018; Wu et al., 2019). The ChIP–qPCR results in HCT116 cells further confirmed that TEAD4 bound to the promoter regions of AJUBA, WTIP, NOCT, SAMD4A, and PNRC1 and to the intronic region of PNRC1 (Figure 1B). Next, we confirmed the significantly downregulated mRNA expression of AJUBA, WTIP, SAMD4A, and NOCT and moderately increased expression of PNRC1 in YAP/TAZ knockdown HCT116 cells by qPCR analysis; this pattern was also observed in A549 lung cancer cells and MDA-MB-231 breast cancer cells (Figure 1C). Consistent with these findings, overexpression of the constitutively active YAP5SA mutant but not the TEAD binding-defective YAP5SA-S94A mutant significantly decreased the mRNA level of PNRC1 and increased the mRNA level of SAMD4A in HCT116, MCF7 and A549 cells (Figure 1D). Enhanced expression of AJUBA and WTIP was observed in HCT116 and MCF7 cells but not in A549 cells (Figure 1D). Since NOCT was not affected by overexpression of YAP5SA in either MCF7 or A549 cells, we did not investigate NOCT in subsequent functional experiments (Figure 1D). Finally, we confirmed that the protein level of PNRC1 was increased by knockdown of YAP/TAZ in HCT116 cells (Figure 1—figure supplement 1C). Additionally, overexpression of YAP5SA but not YAP5SA-S94A decreased the protein level of PNRC1 in HCT116, A549, and MDA-MB-231 cells (Figure 1—figure supplement 1D). Overall, these data demonstrate that YAP/TAZ modulates the transcription of P-body-related genes through the TEAD transcription factors.
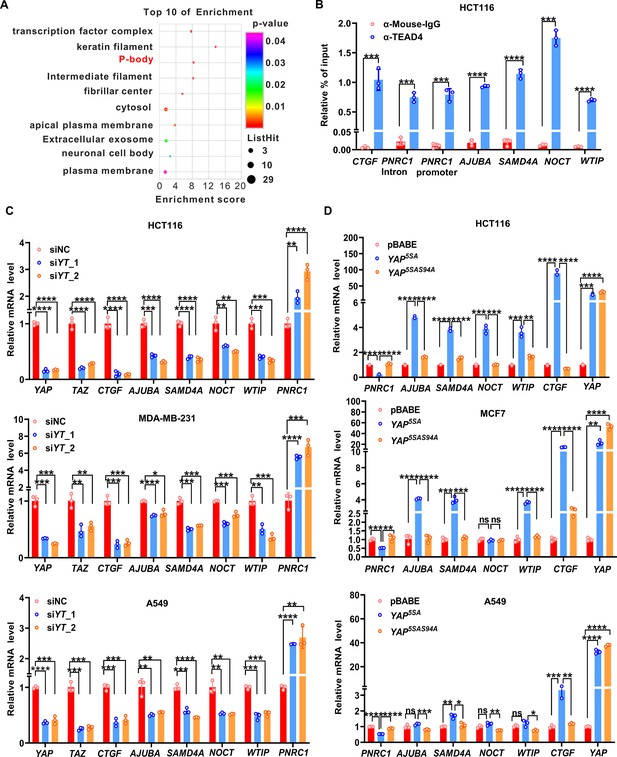
YAP/TAZ transcriptionally regulates genes related to P-bodies.
(A) Gene Ontology (GO) analysis of the downregulated genes upon knockdown of YAP/TAZ in HCT116 cells. The graph shows enrichment in the cellular component category. (B) ChIP–qPCR analysis of endogenous TEAD4 binding to the genomic locus of the indicated P-body-related genes in HCT116 cells. The CTGF promoter was included as the positive control. (C) qPCR analysis of the mRNA levels of the indicated P-body-related genes in YAP/TAZ knockdown HCT116, MDA-MB-231, and A549 cells. Cells were transfected with YAP/TAZ siRNA for 3 d before qPCR analysis. (D) qPCR analysis of the mRNA levels of the indicated P-body-related genes in HCT116, MCF7, and A549 cells stably expressing YAP5SA and YAP5SA-S94A. Cells were infected with YAP5SA- and YAP5SA-S94A-containing retroviruses and selected with puromycin for 1 wk before qPCR analysis. n = 3 biologically independent samples per group. Two-tailed Student’s t-test (B) and one-way ANOVA (C, D) were performed to assess statistical significance in this figure. These data (B–D) are representative of three independent experiments.
-
Figure 1—source data 1
Original data for the statistical analysis in Figure 1B–D.
- https://cdn.elifesciences.org/articles/88573/elife-88573-fig1-data1-v1.xlsx
YAP/TAZ enhances P-body formation
In contrast to stress granule (SG) formation, P-body formation is constitutive and independent of the activation of the integrated stress response (ISR) (Luo et al., 2018; Riggs et al., 2020). DEAD-box ATP-dependent RNA helicase 6 (DDX6) and mRNA-decapping enzyme 1A (DCP1A) are the essential components of P-bodies and are normally used as the biomarkers for P-bodies (James et al., 2010; Lavalée et al., 2021; Luo et al., 2018). To explore whether YAP/TAZ regulates P-body formation, we performed immunofluorescence analysis of DDX6 and DCP1A in YAP/TAZ knockdown cells plated at a low density. We found that knockdown of YAP/TAZ significantly decreased but overexpression of YAP5SA increased the number of DDX6/DCP1A-positive foci in HCT116 cells (Figure 2A and B). HCT116 cells expressing YAP5SA-S94A and control HCT116 cells showed similar numbers of P-bodies, which indicated that the TEAD transcription factors mediate the enhanced effects of YAP/TAZ on P-body formation (Figure 2B). Similar results were observed in YAP/TAZ knockdown MDA-MB-231 cells and YAP5SA/YAP5SA-S94A-expressing A549 cells (Figure 2—figure supplement 1A and B).
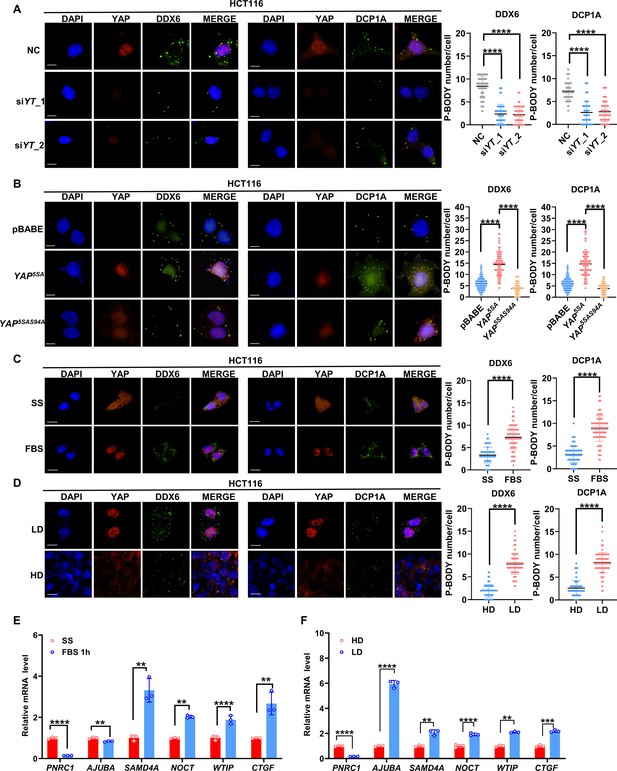
YAP/TAZ promotes P-body formation in colorectal cancer (CRC) cells.
(A) Immunofluorescence analysis of the P-body markers DDX6 and DCP1A in YAP/TAZ knockdown HCT116 cells. Cells were transfected with YAP/TAZ siRNA for 3 d before processing for immunofluorescence staining using anti-DDX6 and anti-DCP1A antibodies. Foci were counted in 100 cells per group. (B) Immunofluorescence analysis of DDX6 and DCP1A in HCT116 cells expressing YAP5SA and YAP5SA-S94A. (C) Immunofluorescence analysis of DDX6 and DCP1A in HCT116 cells. Cells were treated with 10% fetal bovine serum (FBS) for 1 hr after overnight serum starvation (SS). (D) Immunofluorescence analysis of DDX6 and DCP1A in HCT116 cells in sparse or confluent culture. (E, F) qPCR analysis of the indicated genes in HCT116 cells. HCT116 cells were treated with 10% FBS for 1 hr after overnight SD (E) or culture under sparse or confluent conditions in standard culture medium (F). Kruskal–Wallis test (A, B), Mann–Whitney U test (C, D), and two-tailed Student’s t-test (E, F) were performed to assess statistical significance. These data (A–F) are representative of three independent experiments.
-
Figure 2—source data 1
Original data for the statistical analysis in Figure 2A–F.
- https://cdn.elifesciences.org/articles/88573/elife-88573-fig2-data1-v1.xlsx
YAP/TAZ are well known to be activated by serum stimulation and suppressed by high cell densities (Yu et al., 2012; Zhao et al., 2007). Of note, cytoplasmic translocation of YAP at high cell density was first observed in the untransformed NIH3T3 cells (Zhao et al., 2007). Thus, in addition to a series of cancer cell lines, NIH3T3 cells were further included in this study. Consistently, overexpression of YAP5SA but not the YAP5SA-S94A increased the number of DDX6/LSM14A-positive foci in NIH3T3 cells (Figure 2—figure supplement 2A). Upregulation of Ajuba, Samd4 (mouse ortholog of human SAMD4A) and Noct and downregulation of Pnrc1 was also observed in NIH3T3 cells overexpressed with YAP5SA but not cells with YAP5SA-S94A overexpression (Figure 2—figure supplement 2E). Moreover, despite their constitutive formation in cells, the size and number of P-bodies are altered in response to stress (Luo et al., 2018; Riggs et al., 2020). Next, we evaluated P-bodies under exposure to different stimuli. We observed that serum stimulation led to rapid induction of P-body formation in HCT116 and NIH3T3 cells (Figure 2C, Figure 2—figure supplement 2B). Knockdown of YAP/TAZ attenuated the enhancement of P-body formation induced by serum stimulation (Figure 2—figure supplement 1C). Conversely, at a high cell density, the number of P-bodies was significantly decreased in HCT116 and NIH3T3 cells (Figure 2D, Figure 2—figure supplement 2C). Consistent with this finding, the expression of SAMD4A, NOCT, and WTIP in HCT116 cells was induced by serum stimulation and suppressed by culture at a high cell density (Figure 2E and F). Similar results were also observed in NIH3T3 cells (Figure 2—figure supplement 2F and G). Intriguingly, both serum starvation and culture at a high cell density dramatically increased the expression of PNRC1, consistent with the tumor suppressor function of PNRC1 (Figure 2E and F, Figure 2—figure supplement 2F and G). Recent studies have revealed that mechanical cues as an important signal modulating YAP/TAZ activity (Aragona et al., 2013; Dupont et al., 2011). Diverse mechanical forces, such as increased extracellular matrix (ECM) rigidity, cell stretching, shear stress, or the increased area of cell adhesion, can all activate YAP, which is dominant over Hippo signaling (Dasgupta and McCollum, 2019; Piccolo et al., 2014). Next, we examined whether ECM stiffness affected P-body formation. When NIH3T3 cells were shifted from soft (1 kPa) to stiff (40 kPa) matrices, YAP was translocated into nucleus and activated (Figure 2—figure supplement 2D). Furthermore, the P-body formation was enhanced, which was associated with decreased mRNA level of Pnrc1 and increased mRNA levels of Ajuba, Samd4, and Noct (Figure 2—figure supplement 2D and H). Collectively, our data indicate that YAP/TAZ could be positive regulators of P-body formation in response to various stimuli, probably by modulating the expression of P-body-related genes.
SAMD4A, AJUBA, and PNRC1 mediate the functions of YAP/TAZ in regulating P-body formation
Next, we investigated whether the P-body-related genes transcriptionally regulated by YAP/TAZ mediate the biological functions of YAP/TAZ in regulating P-body formation. The LIM-domain proteins AJUBA, WTIP, and LIMD1 are known as negative regulators of LATS1 (Das Thakur et al., 2010). They are also components of P-bodies and are required for miRNA-mediated silencing (James et al., 2010). SAMD4A is the mammalian homolog of Drosophila Smaug, which is involved in translational repression and localized in P-bodies (Baez and Boccaccio, 2005). First, we knocked down AJUBA and SAMD4A in HCT116 cells overexpressing YAP5SA (Figure 3—figure supplement 1A and B). As expected, knockdown of both AJUBA and SAMD4A significantly diminished the promoting effect of YAP5SA overexpression on P-body formation in HCT116 cells (Figure 3A). Unlike AJUBA and SAMD4A, PNRC1 is a tumor suppressor that inhibits P-body formation by recruiting cytoplasmic DCP1A/DCP2 into the nucleolus, thus loss of cytoplasmic DCP1A/DCP2 results in disruption of P-body (Gaviraghi et al., 2018). Overexpression of YAP5SA suppressed PNRC1 expression; thus, WT PNRC1 and PNRC1 with the W300A mutation, which disrupts the interaction between PNRC1 and DCP1A/DCP2, were overexpressed in YAP5SA-expressing HCT116 cells (Figure 3—figure supplement 1C and D; Gaviraghi et al., 2018). We observed that overexpression of WT PNRC1 but not the W300A mutant dramatically decreased the number of P-bodies in YAP5SA-expressing HCT116 cells (Figure 3B). We also examined whether the attenuation of P-body formation by knockdown of YAP/TAZ can be restored by knockdown of PNRC1 (Figure 3—figure supplement 1E). Consistent with the above findings, the reduction in the P-body number was reversed by knockdown of PNRC1 in YAP/TAZ knockdown HCT116 cells (Figure 3—figure supplement 1F). Collectively, these findings indicate that YAP/TAZ enhances P-body formation through modulation of a series of P-body-related genes. Both activation of SAMD4A and AJUBA expression and downregulation of PNRC1 are involved in YAP/TAZ-induced P-body formation.
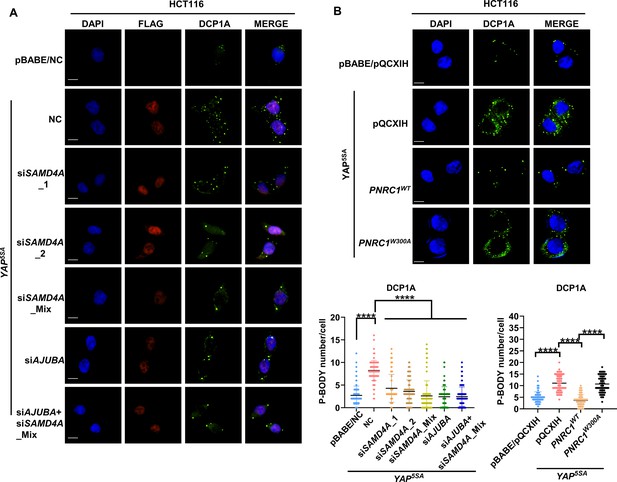
SAMD4A, AJUBA, and PNRC1 mediate the regulatory functions of YAP/TAZ in P-body formation.
(A) Immunofluorescence analysis of DDX6 and DCP1A in HCT116 cells stably expressing YAP5SA and YAP5SA-expressing cells transiently transfected with SMAD4A and AJUBA siRNA. Foci were counted in 100 cells per group. (B) Immunofluorescence analysis of DDX6 and DCP1A in HCT116 cells expressing YAP5SA alone or in combination with PNRC1WT or PNRC1W300A. Kruskal–Wallis test was performed to assess statistical significance. These data (A–B) are representative of three independent experiments.
-
Figure 3—source data 1
Original data for the statistical analysis in Figure 3A and B.
- https://cdn.elifesciences.org/articles/88573/elife-88573-fig3-data1-v1.xlsx
YAP/TAZ inhibit PNRC1 gene transcription by recruiting the NuRD complex
PNRC1 is a newly identified tumor suppressor gene whose expression is frequently downregulated in cancer (Gaviraghi et al., 2018). Thus, we further explored the molecular mechanism of YAP/TAZ in inhibiting the PNRC1 gene transcription. The ChIP-seq data for TEAD4 at the PNRC1 gene locus in multiple cancer cells implicated PNRC1 as a potential direct target gene of YAP/TAZ-TEAD transcription complexes (Figure 1—figure supplement 1B). As ChIP–qPCR analysis of TEAD4 in HCT116 cells revealed one TEAD4 binding site at the PNRC1 promoter and another in the PNRC1 intron, we constructed PNRC1 promoter and PNRC1 intron luciferase reporter plasmids. We observed that overexpression of YAP5SA significantly decreased the luciferase activity of both the PNRC1 promoter and intron reporters (Figure 4A). Compared with the 5SA mutation in YAP, the S94A mutation resulted in a decreased suppressive effect on PNRC1 promoter and intron luciferase reporter activity (Figure 4A). In contrast, the luciferase activity of both the PNRC1 promoter and intron reporters was significantly enhanced in YAP/TAZ knockdown HCT116 cells (Figure 4B, Figure 4—figure supplement 1A). Bioinformatic analysis of TEAD4 ChIP peaks in the PNRC1 promoter and intronic regions with JASPAR revealed the existence of one TEAD binding motif in each peak region; thus, we further constructed PNRC1 luciferase reporter plasmids with mutated TEAD binding sites. Consistent with the above results, mutation of the TEAD binding sites abolished the inhibitory effect of YAP5SAon the PNRC1 promoter and intron luciferase reporters (Figure 4C). Similarly, mutation of the TEAD binding sites escaped the derepression of PNRC1 promoter and intron luciferase reporters by YAP/TAZ knockdown (Figure 4D). Furthermore, the ChIP–qPCR results confirmed that YAP bound to the promoter and intronic regions of PNRC1, which required its interaction with TEADs (Figure 4—figure supplement 1B and C).
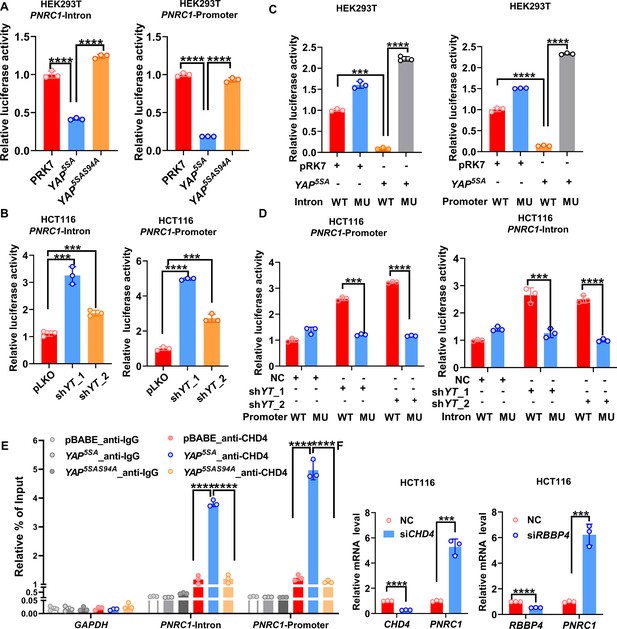
YAP suppresses PNRC1 gene transcription by recruiting the NuRD complex.
(A) Overexpression of YAP5SA but not YAP5SA-S94A decreased the luciferase activity of the PNRC1 promoter and intron reporters. HEK-293T cells were transfected with the indicated FLAG-YAP5SA and YAP5SA-S94A expression plasmids and the PNRC1 promoter or intron luciferase reporter. (B) Knockdown of YAP/TAZ stimulated the luciferase activity of the PNRC1 promoter and intron reporters. The PNRC1 promoter or intron luciferase reporter plasmid and the Renilla luciferase reporter plasmid were co-transfected into HCT116 cells stably expressing pLKO-vec, shYAP/TAZ-1, or shYAP/TAZ-2. (C, D) Luciferase assay of the PNRC1 promoter/intron WT reporters and mutant reporters with TEAD binding motif mutations in HEK-293T cells (C) and HCT116 cells (D). (E) ChIP–qPCR analysis of CHD4 binding to the PNRC1 promoter and intronic regions in control and HCT116 cells stably expressing FLAG-YAP5SA or YAP5SA-S94A. (F) qPCR analysis of PNRC1, CHD4, and RBBP4 in HCT116 cells transfected with the indicated siRNAs. n = 3 biologically independent samples per group. One-way ANOVA (A–E) and two-tailed Student’s t-test (F) were performed to assess statistical significance in this figure. These data (A–F) are representative of two independent experiments.
-
Figure 4—source data 1
Original data for the statistical analysis in Figure 4A–F.
- https://cdn.elifesciences.org/articles/88573/elife-88573-fig4-data1-v1.xlsx
In addition to functioning as transcriptional coactivators, YAP/TAZ can also act as transcriptional corepressors by recruiting the NuRD complex (Kim et al., 2015b). The ChIP–qPCR results showed that the NuRD complex component CHD4 was recruited to the promoter and intronic regions of the PNRC1 gene by overexpressed YAP5SA but not by the TEAD binding-defective YAP5SA-S94A mutant (Figure 4E). Compared to the genomic locus of PNRC1, the binding enrichment of CHD4 at the YAP target genes activated by YAP/TAZ was relatively lower and not affected by overexpression of YAP (Figure 4—figure supplement 1D). Moreover, knockdown of the NuRD complex components CHD4 and RBBP4 significantly upregulated the mRNA expression of PNRC1 in HCT116 cells (Figure 4F). Consistently, knockdown of CHD4 significantly decreased the number of DDX6/DCP1A-positive foci in HCT116 cells (Figure 4—figure supplement 1E). Taken together, these data demonstrate that YAP/TAZ inhibits PNRC1 gene transcription through direct binding of TEADs to the PNRC1 gene locus and that the NuRD complex is required for the transcriptional repression of PNRC1 by YAP/TAZ.
PNRC1 suppresses the oncogenic function of YAP in CRC
Analysis of colorectal (COAD) and rectal (READ) TCGA datasets revealed that the mRNA level of PNRC1 was significantly decreased in CRC (Figure 5—figure supplement 1A). We further confirmed the decreased mRNA level of PNRC1 in CRC by qPCR analysis of 16 CRC tissues with paired normal mucosal tissues; this finding implies that PNRC1 is a potential tumor suppressor also in CRC (Figure 5—figure supplement 1B). Thus, we sought to explore whether downregulation of PNRC1 mediates the oncogenic function of YAP in CRC. To this end, we examined whether the YAP overexpression-induced oncogenic phenotype can be attenuated by coexpression of YAP5SA with WT PNRC1 or the W300A mutant in HCT116 cells. We observed that reexpression of WT PNRC1 almost completely abolished the increases in cell proliferation and colony formation induced by YAP5SA overexpression in HCT116 cells (Figure 5A and B). Re-expression of the PNRC1 W300A mutant did not affect the proliferation and colony formation of YAP5SA-expressing HCT116 cells, which implied that the suppressive effect of PNRC1 on YAP relies on the recruitment of cytoplasmic DCP1A/DCP2 into the nucleolus by PNRC1 (Figure 5A and B). Similarly, overexpression of PNRC1 WT but not PNRC1 W300A suppressed the increase in migration induced by YAP5SA in HCT116 cells (Figure 5C). To verify the tumor-suppressive effect of PNRC1 on YAP in CRC in vivo, we performed a xenograft assay by subcutaneously injecting HCT116 cells into nude mice. Consistent with the above findings, reexpression of WT PNRC1 but not the W300A mutant dramatically inhibited the growth of YAP5SA-expressing HCT116 xenografts, and xenograft tumors formed from HCT116 cells coexpressing YAP5SA and PNRC1 were significantly smaller than the tumors formed from HCT116 cells expressing YAP5SA alone or in combination with the PNRC1 W300A mutant (Figure 5D). Ki67 staining of xenograft tumors further showed fewer Ki67-positive cells in xenograft tumors formed from HCT116 cells coexpressing YAP5SA and PNRC1 (Figure 5E, Figure 5—figure supplement 1C). Next, we examined whether the YAP/TAZ knockdown-induced attenuation of the oncogenic phenotype can be restored by knockdown of PNRC1 in HCT116 cells. Intriguingly, the decrease in proliferation and attenuation of migration induced by YAP/TAZ knockdown were reversed by knockdown of PNRC1 in HCT116 cells (Figure 5—figure supplement 1D and E). Overall, these results indicate that YAP promotes tumorigenesis by downregulating PNRC1 expression and that reexpression of PNRC1 suppresses YAP-driven tumor growth.
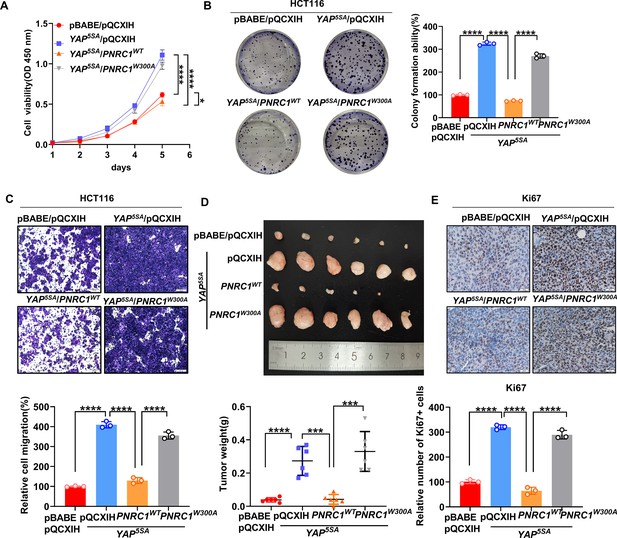
PNRC1 attenuates the oncogenic function of YAP in colorectal cancer (CRC).
(A) CCK8 proliferation assays of HCT116 cells stably expressing YAP5SA alone or in combination with of PNRC1WT or PNRC1W300A. n = 4 biologically independent samples per group. (B, C) Colony formation assay (B) and Transwell assay (C) of HCT116 cells stably expressing YAP5SA alone or in combination with PNRC1WT or PNRC1W300A. n = 3 biologically independent samples per group. (D) Representative images of xenograft tumors formed from HCT116 cells stably expressing YAP5SA alone or in combination with of PNRC1WT or PNRC1W300A (n = 6). (E) Representative images of IHC staining of the proliferation marker Ki67 in xenograft tumors formed from HCT-116 cells stably expressing YAP5SA alone or in combination with PNRC1WT or PNRC1W300A (n = 3). Two-way ANOVA (A) and one-way ANOVA (B–E) were performed to assess statistical significance in this figure. These data (A–C) are representative of two independent experiments.
-
Figure 5—source data 1
Original data for the statistical analysis in Figure 5A–E.
- https://cdn.elifesciences.org/articles/88573/elife-88573-fig5-data1-v1.xlsx
P-body disassembly attenuates YAP-driven cell proliferation and migration in CRC
Due to the inhibitory effects of PNRC1 on P-body formation and the YAP-induced oncogenic phenotype, we evaluated whether enhanced P-body formation plays a vital role in YAP-driven cancer cell proliferation and migration. The proteins LSM14 homolog A (LSM14A) and DDX6 are essential nucleating proteins for P-body assembly, and DCP1A plays a vital role in further RNP aggregation, which is required for stress-dependent P-body aggregation (Lavalée et al., 2021; Luo et al., 2018; Riggs et al., 2020). To explore the requirement of P-body formation for the YAP-induced oncogenic phenotype, we generated YAP5SA-expressing HCT116 cell lines with stable knockdown of DCP1A, LSM14A, and DDX6 (Figure 6—figure supplement 1A, Figure 6—figure supplement 2A–C). By immunofluorescence analysis of DDX6 and DCP1A, we further confirmed the knockdown of DCP1A and DDX6 and observed a reduced number of P-bodies upon knockdown of DCP1A, LSM14A or DDX6 in YAP5SA-expressing HCT116 cells (Figure 6—figure supplement 1B). Next, by using a CCK8 assay, we found that knockdown of DCP1A, LSM14A, and DDX6 suppressed the proliferation of YAP5SA-expressing and control ‘parental’ HCT116 cells, consistent with the results of the colony formation assay (Figure 6A and B). As an oncogene, YAP is known to promote cell division and inhibit cell apoptosis of cancer cells (He et al., 2020; Jang et al., 2017). By analyzing the cell cycle and cell apoptosis, we further found that knockdown of DCP1A, LSM14A, and DDX6 all led to downregulation of cell mitosis and increased cell apoptosis, which was opposite to the effect of YAP5SA overexpression in HCT116 cells (Figure 6—figure supplement 3A and B). Furthermore, knockdown of either DCP1A or LSM14A significantly attenuated the enhancement of cell migration induced by overexpression of YAP5SA in HCT116 cells (Figure 6C). In contrast, knockdown of DDX6 showed stimulative effect on the migration of both control and YAP5SA-expressing HCT116 cells, possibly due to the diverse functions of DDX6 (Figure 6—figure supplement 4A; Di Stefano et al., 2019). To further demonstrate the potential role of P-body mediating the function of YAP/TAZ in CRC, we established YAP5SA-expressing HCT116 cell lines with stable knockdown of AJUBA and SAMD4A (Figure 6—figure supplement 4B and C). Indeed, both knockdown of AJUBA and SAMD4A suppressed the proliferation and cell migration of YAP5SA-expressing and control ‘parental’ HCT116 cells (Figure 6—figure supplement 4D and E). Collectively, our data demonstrate that P-body formation is required for the oncogenic function of YAP in CRC.
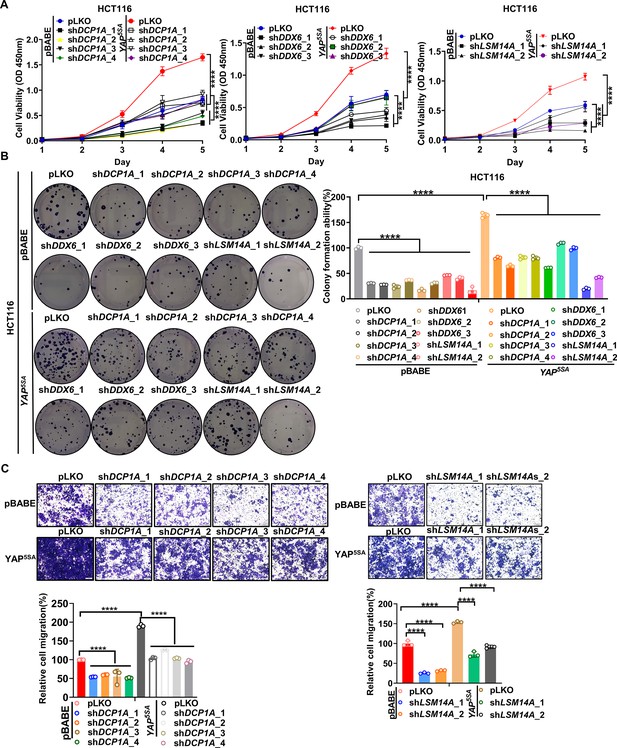
Knockdown of P-body-related core genes suppresses the oncogenic function of YAP in colorectal cancer (CRC).
(A) CCK8 proliferation assays of control HCT116 cells with or without knockdown of DCP1A, LSM14A or DDX6 and HCT116 cells stably expressing YAP5SA with or without knockdown of DCP1A, LSM14A, or DDX6. n = 5 biologically independent samples per group. (B, C) Colony formation assay (B) and Transwell assay (C) of control HCT116 cells with or without knockdown of DCP1A, LSM14A, or DDX6 and HCT116 cells stably expressing YAP5SA with or without knockdown of DCP1A, LSM14A, or DDX6. n = 3 biologically independent samples per group. Two-way ANOVA (A) and one-way ANOVA (B, C) were performed to assess statistical significance in this figure. These data (A–C) are representative of three independent experiments.
-
Figure 6—source data 1
Original data for the statistical analysis in Figure 6A–C.
- https://cdn.elifesciences.org/articles/88573/elife-88573-fig6-data1-v1.xlsx
Codependency of YAP/TEAD and essential P-body-related genes in pancancer CRISPR screens
Based on the observation that P-body disassembly attenuates YAP-driven cell proliferation in CRC cells, we speculated that cancer cells whose proliferation is dependent on YAP should also be vulnerable to knockout of essential P-body genes. To this end, we analyzed the Cancer Dependency Map (DepMap), which aims to systematically assess the effect of single-gene inactivation on cell proliferation by CRISPR and shRNA screens and define genetic dependencies in hundreds of cancer cell lines by integrating data pertaining to multiple molecular characteristics, such as Cancer Cell Line Encyclopedia (CCLE) data (Dempster et al., 2021; Tsherniak et al., 2017). As expected, by analyzing gene expression data from the CCLE, we observed a strong positive correlation between YAP-regulated P-body-related genes (SAMD4A, AJUBA, and WTIP) and canonical target genes of YAP (CTGF, CYR61, AXL, and AMOTL2) in cell lines across cancers or in cell lines of colorectal, breast and lung lineages (Figure 7A, Figure 7—figure supplement 1A and B). IHC analysis of 294 CRC tissues further showed the positive correlation between the expression of AJUBA/SAMD4A and YAP (Figure 7—figure supplement 1D). Although there were no correlations between PNRC1 and YAP target genes in cell lines across cancers, we found that the mRNA level of PNRC1 was negatively correlated with that of YAP target genes in cancer cells of thyroid and central nervous system (CNS) lineages (Figure 7B, Figure 7—figure supplement 1C). Next, we analyzed the effect of P-body core gene knockout in 1070 cancer cell lines (DepMap 22Q1 Public+Score, Chronos). Strikingly, knockout of the P-body-nucleation-determining genes DDX6 and LSM14A inhibited proliferation in multiple cancer cell lines (negative Chronos score) (Figure 7C). Similar results were observed for EDC4, which is required for P-body aggregation (Figure 7C). Logically, correlations between dependency profiles suggest functionality in the same pathway or regulatory axis; thus, EDC4 is strongly associated with multiple known P-body genes (DDX6, DCP2, EIF4ENIF1, etc.) (Supplementary file 2). Furthermore, we found that YAP ranked 14th among genes correlating with EDC4 (Figure 7C, Supplementary file 2). In addition, the YAP dependency score was positively correlated with the DDX6 and LSM14A scores (Figure 7C). Similar relationships were observed between EDC4/DDX6/LSM14A and TEAD1/3 (Figure 7—figure supplement 2). Last, we examined whether cell proliferation and cell migration are affected by the knockdown of DCP1A or LSM14A and overexpression of PNRC1 in MCF7, MDA-MB-231, and A549 cell lines, whose proliferation is dependent on YAP/TAZ activity. Consistent with the observation in HCT116 cells, the knockdown of DCP1A/LSM14A and overexpression of PNRC1 attenuated both cell proliferation and cell migration in these three YAP-dependent cancer cells (Figure 7—figure supplements 3–5). Collectively, the co-dependencies of YAP/TEAD and essential P-body genes further suggest that enhanced P-body formation plays a vital role in YAP-induced tumorigenesis.
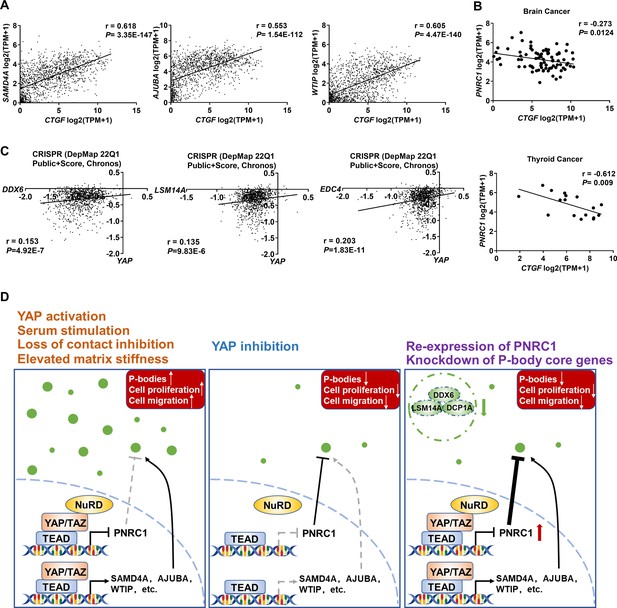
DepMap analysis reveals the co-dependencies of YAP/TEAD and P-body core genes in pancancer CRISPR screens.
(A) Positive correlations between the mRNA levels of CTGF and SAMD4A/AJUBA/WTIP in 1393 cancer cell lines. (B) Negative correlation between the mRNA levels of CTGF and PNRC1 in brain cancer cell lines (n = 83) and thyroid cancer cell lines (n = 17). (C) Positive correlations between the dependency scores of YAP and DDX6/LSM14A/EDC4 in 1070 cancer cell lines. The Chronos dependency scores were extracted from the DepMap database. The negative Chronos score indicates decreased cell proliferation upon gene knockout. Pearson correlation analysis was used to assess statistical significance. (D) In response to serum stimulation or under loss of contact inhibition or reduced ECM stiffness, activation of YAP enhances the P-body formation to promote colorectal cancer (CRC) cell proliferation and migration. Disruption of P-bodies by overexpression of the tumor suppressor gene PNRC1 or knockdown of P-body core genes could attenuate the cell proliferation and migration induced by activation of YAP in CRC cells.
-
Figure 7—source data 1
Original data for the statistical analysis in Figure 7A–C.
- https://cdn.elifesciences.org/articles/88573/elife-88573-fig7-data1-v1.xlsx
Discussion
Dysregulation of the Hippo pathway occurs in a variety of cancers, leading to cell transformation and diverse changes in tumor cells through activation of the YAP/TEAD transcriptional program (Calses et al., 2019; Kulkarni et al., 2020; Nguyen and Yi, 2019; Wang et al., 2018; Zanconato et al., 2016). Here, we demonstrated the crucial role of YAP/TEAD in regulating P-body formation in multiple cancer cell lines. Through transcriptional stimulation of positive regulators of P-body formation (AJUBA, WTIP, and SAMD4A) and suppression of negative regulators of P-body formation (PNRC1), YAP enhances P-body formation and increases the number of P-bodies in cancer cells, which suggests that YAP is a positive regulator of P-body formation (Figure 7D). Studies of P-bodies in yeast have shown that the size and number of P-bodies increase upon exogenous and endogenous stress (Luo et al., 2018). In addition, our study revealed that the number of P-bodies decreases under serum starvation, contact inhibition, and decreased ECM rigidity, possibly due to inactivation of YAP/TEAD. In contrast, a recent study, which provided the first link between YAP and P-bodies, implicated YAP as a negative regulator of P-bodies in KHSV-infected HUVECs (Castle et al., 2021). Elizabeth L. Castle et al. reported that virus-encoded Kaposin B (KapB) induces actin stress fiber formation and disassembly of P-bodies, which requires RhoA activity and the YAP transcriptional program (Castle et al., 2021). YAP-enhanced autophagic flux was proposed to participate in KapB-induced P-body disassembly, consistent with the concept that SGs and P-bodies are cleared by autophagy (Buchan et al., 2013; Castle et al., 2021). However, an increasing number of studies have reported the contradictory role of YAP in autophagy regulation, which suggests that YAP-mediated autophagy regulation is cell type- and context-dependent (Jin et al., 2021; Pei et al., 2022; Totaro et al., 2019; Wang et al., 2020). Furthermore, though YAP is required for the cell proliferation in HUVEC, transformed cell lines often display elevated baseline YAP/TAZ activity compared to normal cells and possess many alterations in growth signaling pathways including autophagy signaling (Nguyen and Yi, 2019; Shen and Stanger, 2015; Zanconato et al., 2016). Thus, the contradictory observations regarding the role of YAP in modulating P-body formation between Elizabeth L. Castle et al.’s study and our study could be due to the different cell contexts and different cell conditions (baseline vs. KHSV infection).
In addition to the transcriptional regulation, P-body dynamics can also be modulated by post-translational modifications (Luo et al., 2018). P-body constituent proteins, such as DCP1A and DCP2, are phosphorylated, which affects the protein interaction between DCP1A and DCP2 and subsequent P-body assembly (Chiang et al., 2013; Yoon et al., 2010). Previous studies have shown that DCP1A is hyperphosphorylated during mitosis and P-body assembly is dynamically changed across the cell cycle (Aizer et al., 2013; Yang et al., 2004). Meanwhile, Hippo signaling is intrinsically regulated and YAP can also be directly phosphorylated by CDK1 during cell cycle progression (Kim et al., 2019; Yang et al., 2013). Besides, as a direct regulator of P-body formation, AJUBA is also phosphorylated by CDK1 and mitotic phosphorylation of AJUBA promotes cancer cell proliferation (Chen et al., 2016). Thus, we speculated that mitotic phosphorylation of YAP and AJUBA might also play a potential role in modulating P-body dynamics during cell cycle.
Compared with the role of SGs, the role of P-bodies in tumorigenesis and tumor progression is not well studied and is considered to be cancer type- or context-dependent (Lavalée et al., 2021). TGF-β induces P-body formation and EMT in mammary epithelial cells, while inhibition of P-body formation by knockdown of DDX6 reverses EMT and suppresses breast cancer metastasis, implying a prometastatic function of P-bodies during the progression of breast cancer (Hardy et al., 2017). In prostate cancer cells, dephosphorylation of EDC3 promotes the localization of EDC3-containing P-bodies and increases the P-body number (Bearss et al., 2021). The increase in EDC3-containing P-bodies leads to sequestration or decay of a subset of mRNAs related to cell attachment and cell growth, such as ITGB1, ITGA6, and KLF4, which ultimately inhibits cell proliferation and cell migration (Bearss et al., 2021). Of note, EDC3 and LSM14A compete for binding to the P-body core protein DDX6, and P-body formation still occurs constitutively in EDC3 KO prostate cancer cells (Bearss et al., 2021). Therefore, it can be speculated that there might be different types of P-bodies that contain different RNAs and exert protumorigenic or tumor-suppressive functions in different cell contexts. In CRC, DCP1A expression is elevated, which is associated with advanced TNM stages, lymph node metastasis and poor prognosis, and overexpression of DCP1A enhances P-body formation (Wu et al., 2018a; Wu et al., 2018b). These studies imply the potential protumorigenic function of P-bodies in CRC. Furthermore, our study showed that disruption or attenuation of P-body formation by knockdown of YAP-regulated P-body-related genes or the P-body core genes (DDX6, DCP1A, LSM14A) suppressed YAP-induced oncogenic phenotypes in CRC cells, such as cell proliferation and cell migration, further indicating the protumorigenic function of P-bodies in CRC or at least in CRC with active YAP. Numerous studies have demonstrated the YAP/TAZ promotes cancer cell growth through direct transcriptional regulation of genes related to cell cycle and cell apoptosis (He et al., 2020; Jang et al., 2017). Since P-bodies control the storage of untranslated mRNAs, YAP/TAZ might modulate gene expression by indirectly promoting P-body formation and the storage of untranslated target mRNAs. Future work is needed to explore P-body-enriched RNAs in CRC cells, which will further uncover the underlying mechanism by which P-bodies mediate the oncogenic function of YAP.
Recently, a study exploring new TSGs based on hemizygous deletions in multiple cancers revealed that PNRC1 is a novel tumor suppressor gene (Gaviraghi et al., 2018). PNRC1 translocates the cytoplasmic DCP1A/DCP2 decapping complex into the nucleolus, which subsequently impedes rRNA transcription and ribosome biogenesis (Gaviraghi et al., 2018). This translocation of DCP1A/DCP2 also leads to disassembly of P-bodies; thus, PNRC1 could also inhibit tumor cell proliferation by disrupting P-body formation. Moreover, hemizygous deletion of the 6q15 locus, where PNRC1 is located, occurs in multiple cancers, including prostate, pancreatic, breast, and liver cancers (Gaviraghi et al., 2018). Our findings suggest that transcriptional downregulation of PNRC1 by YAP activation could be a new mechanism of PNRC1 dysregulation during tumorigenesis. In addition, multiple oncogenes, such as MYC, RAS, and PI3K, can activate rRNA transcription and boost ribosome biogenesis to support cancer cell proliferation (Pelletier et al., 2018). The presence of the YAP-PNRC1 regulatory axis implies a potential role of YAP in ribosome biogenesis, which warrants further investigation in follow-up studies.
It has been shown that PNRC1 inhibits RAS- and MYC-driven tumor cell proliferation (Gaviraghi et al., 2018). In addition, YAP acts downstream of mutant KRAS, and activation of YAP was found to drive KRAS-independent tumor relapse in preclinical models of pancreatic cancer (Kapoor et al., 2014; Shao et al., 2014; Zhang et al., 2014). Strikingly, reexpression of PNRC1 also dramatically diminished the cell proliferation induced by YAP overexpression in CRC cells in our study. These data indicate that PNRC1 is a tumor suppressor gene with a strong antitumor effect on various oncogenes; thus, reexpression of PNRC1 could be a promising anticancer therapeutic strategy. In addition, as an alternative to targeting the YAP/TEAD complex, drugs that inhibit downstream effectors of YAP/TAZ have shown efficacy in the clinic (Gay et al., 2017; Neesse et al., 2013; Nguyen and Yi, 2019). The identification of the P-body as a new downstream effector of YAP/TAZ suggests that disruption of P-bodies might be a potential therapeutic strategy for tumors with active YAP. Although each P-body core gene performs multiple biological functions, unbiased functional CRISPR screening across cancer cell lines (DepMap) revealed that loss of function of a series of P-body core genes significantly suppresses proliferation in various tumor cell lines. The functional overlap in P-body assembly and the positive correlation between the dependency profiles of these P-body core genes imply the important role of P-bodies in tumor cell proliferation and cell survival. Several compounds, including translation inhibitors, have been reported to inhibit P-body formation (Martínez et al., 2013; Stribinskis and Ramos, 2007). Notably, actin polymerization can activate YAP (Sun and Irvine, 2016). Methyl-chivosazol, an actin polymerization inhibitor, was found to be a strong inhibitor of P-body formation by screening of a library of compounds derived from myxobacteria (Martínez et al., 2013). However, these small molecules indirectly target P-bodies and show extensive effects on cells (Martínez et al., 2013; Stribinskis and Ramos, 2007). Thus, the development of inhibitors directly targeting P-body core proteins will provide a chemical tool for exploring the function of P-bodies in tumors and assess the therapeutic efficacy of P-body disassembly in cancer. Overall, our study reveals the P-body as a new downstream effector of YAP/TAZ, which opens a new possibility of targeting P-body assembly to combat tumors (Figure 7D).
Materials and methods
Cell culture and transfection
Request a detailed protocolHEK293T, NIH3T3, HCT116, MCF7, MDA-MB-231, and A549 cells were purchased from the American Type Culture Collection (ATCC) and authenticated by short tandem repeat analysis. HEK293T, NIH3T3, HCT116, and MDA-MB-231 cells were cultured in Dulbecco's Modified Eagle Medium (DMEM)/high-glucose (HyClone) supplemented with 10% fetal bovine serum (FBS), 100 units/ml penicillin, and 100 μg/ml streptomycin (Sangon Biotech) at 37°C in 5% CO2. MCF7 cells were cultured in Minimum Essential Medium (MEM)/high-glucose (HyClone), A549 cells were cultured in RPMI-1640 high-glucose medium (HyClone), and the other culture conditions were the same as those used for HCT116 cells. Corning TC-treated Culture Dish was used for routine cell culture. For the cell culture with 2D polyacrylamide-based hydrogels, hydrogels of high (40.40 ± 2.39 kPa) or low (1.00 ± 0.31 kPa) stiffness were generated according to the published protocol (Tse and Engler, 2010). Fibronectin solution (3 μg/ml) and Sigmacote were used to coat the surface of the hydrogels. PEI (Polysciences) and Lipofectamine 2000 (Invitrogen) were used for plasmid transfection. Lipofectamine RNAiMAX (Invitrogen) was used for siRNA transfection.
Plasmids and reagents
Request a detailed protocolFull-length PNRC1 cDNA was inserted into the pQCXIH vector, and the mutant pQCXIH-FLAG-PNRC1W300A plasmid was constructed by using a KOD mutagenesis kit (Toyobo, Osaka, Japan) according to the manufacturer’s instructions. The pBABE-FLAG-YAP5SA/YAP5SA-S94A and pRK7-FLAG-YAP5SA/YAP5SA-S94A plasmids were obtained from laboratory storage. To generate the shRNA constructs targeting human TAZ, the targeting sequences were inserted into the pLKO.1-puro vector. The shRNA constructs targeting human YAP, SAMD4A, AJUBA, DDX6, DCP1A, and LSM14A were generated by using the pLKO.1-hygro vector. The PNRC1 promoter and intron reporter plasmids and TEAD binding site mutant reporter plasmids were constructed by using the pGL3-Basic vector. siRNA/shRNA was used for all RNA silencing experiments in the study. The siRNA oligos targeting CHD4, RBBP4, PNRC1, SAMD4A, AJUBA, YAP, and TAZ were synthesized by Shanghai Genepharma Co., Ltd. The shRNA and siRNA targeting sequences and the primers used for plasmid construction are listed in Supplementary file 3. The following antibodies were used in this study: anti-FLAG (D6W5B, CST), anti-FLAG (M2, Sigma), anti-YAP/TAZ (D24E4, CST), anti-YAP (sc-101199, Santa Cruz), anti-TEAD4 (ab58310, Abcam), anti-CHD4 (14173-1-AP, Proteintech), anti-DDX6 (A9634, ABclonal), anti-DCP1A (A6824, ABclonal), anti-LSM14A (18336-1-AP, Proteintech), anti-AJUBA (A22039, ABclonal), anti-SAMD4A (17387-1-AP, Proteintech), and anti-PNRC1 (51052-1-AP, Proteintech).
Cell proliferation, colony formation, and cell migration assays
Request a detailed protocolFor the proliferation assay, cells (1 × 103 per well) were seeded into a 96-well plate and cultured for 5 d. Cell viability was measured every day with a Cell Counting Kit 8 (CCK8) (Vazyme) according to the manufacturer’s instructions. Briefly, 10 μl of CCK8 reagent was added to each well and incubated for 2 hr. The absorbance at 450 nm was measured with a microplate reader to determine the relative numbers of viable cells. For the colony formation assay, cells (1 × 103 per well) were seeded and cultured in six-well plates for 2 wk. Then, the cells were stained with 1% crystal violet, and the number of colonies in each well was counted. For the cell migration assay, cells (1.5 × 105 per well) in DMEM/high-glucose containing 0.1% FBS were seeded in the upper compartment of a Transwell chamber, while DMEM/high-glucose containing 10% FBS was placed in the lower compartment. After 60 hr, migrated cells were stained with 1% crystal violet and counted.
Cell cycle and cell apoptosis assays
Request a detailed protocolCell cycle and cell apoptosis assays were performed according to the manufacturer’s instruction (FITC Annexin V Apoptosis Detection Kit I, BD, 556547; PI/RNase staining buffer, BD, 550825).
qPCR, ChIP, and luciferase reporter assays
Request a detailed protocolqPCR and ChIP were performed as previously described (Zhu et al., 2020). For the luciferase assay, HEK293T cells were seeded in 24-well plates, incubated overnight to 50% confluence, and then co-transfected with the PNRC1 luciferase reporter plasmid and the FLAG-YAP5SA or FLAG-YAP5SA-S94A plasmid. A Renilla luciferase plasmid was used as the control. After 24–36 hr, luciferase activity was measured by using a dual-luciferase reporter assay (Promega) and normalized to Renilla luciferase activity. For the luciferase assay using the YAP/TAZ knockdown of HCT116 cells, stable HCT116 cells were seeded in 24-well plates and co-transfected with the PNRC1 luciferase reporter and Renilla luciferase plasmids for 6 hr. Then, cells were replated to six-well plates and cultured for 24–36 hr at a low cell density before measuring the luciferase activity.
Immunofluorescence staining
Request a detailed protocolCells were seeded in glass-bottom cell culture dishes one night before IF staining. The cells were washed with phosphate-buffered saline (PBS) and fixed with 4% paraformaldehyde. After the cells were permeabilized with 0.1% Triton X-100 at room temperature (RT) for 5 min, they were blocked with 3% Bovine Serum Albumin (BSA) at RT for 1 hr. Then, the cells were incubated overnight with a rabbit anti-DDX6/DCP1A/LSM14A antibody (1:250) or a mouse anti-FLAG (M2) antibody (1:150) or mouse anti-YAP antibody (1:100). After a 1 hr incubation with Cy3-conjugated mouse and Alexa Fluor 488-conjugated rabbit secondary antibodies followed by a 1 min incubation with 0.1 μg/ml DAPI, the cells were visualized with an Olympus IX81 microscope.
Xenograft assay and immunohistochemistry
Request a detailed protocolNude mice (4–6 weeks old, male) were obtained from SLAC Laboratory Animals LLC, Shanghai, China. All mouse procedures were approved by the Xinhua Hospital Animal Care and Use Committee. Male nude mice (4–6 weeks old) were randomly divided into four groups (n = 6 mice per group) and injected in the right flank with 2 × 106 of the indicated stable HCT116 cells resuspended in 100 μl of PBS. Mice were sacrificed on day 21, and the xenograft tumors were removed, photographed, and paraffin embedded for sectioning. The xenograft tumors were sectioned for H&E staining and immunohistochemical staining with anti-ki67 and anti-PNRC1 antibodies as described previously (Zhu et al., 2020).
Colorectal cancer specimen
Request a detailed protocolPatients with CRC who underwent curative surgery without prior treatments at the Department of Colorectal and Anal Surgery, Xinhua Hospital, Shanghai Jiao Tong University School of Medicine, between January 2008 and December 2018 were enrolled. Institutional review board approval and informed consent were obtained for all sample collections. None of the patients had any history of other tumors. Tumors and paired paracancerous normal tissues were collected during surgery. The generation of the CRC tissue array has been described, and the IHC analysis of the CRC tissue array was performed according to our previous study (Zhu et al., 2020).
Statistical analysis
Request a detailed protocolStatistical analysis was performed by using GraphPad Prism 8.0.2 and SPSS 22.0. Typically, differences between two groups were evaluated using two-tailed Student’s t-test or the chi-square test as indicated in the figure legends. One-way ANOVA was used for the experiments with more than two groups. Mann–Whitney U test and Kruskal–Wallis test were used for statistical analysis of the foci number in IF staining assay. Two-way ANOVA was used for statistical analysis of CCK8 assay. Paired Student’s t-test was performed to assess the statistical significance of differential PNRC1 mRNA expression in 16 pairs of CRC and adjacent normal tissues. Pearson correlation analysis was used to statistically assess correlations of mRNA levels or co-dependencies from the DepMap database. The results are shown as averages; the error bars indicate the SDs. p-Values less than 0.05 were considered significant (*p<0.05; **p<0.01; ***p<0.001; ****p<0.0001).
Data availability
All WB source data generated during this study are included in the manuscript and supporting files. Source data files have been provided for figures.
-
NCBI Gene Expression OmnibusID GSE176475. Identification of differential expressed genes after HHEX/YAP/TAZ/TEAD knockdown in colorectal cancer cells.
References
-
Stress granules, P-bodies and cancerBiochimica et Biophysica Acta 1849:861–870.https://doi.org/10.1016/j.bbagrm.2014.11.009
-
Mammalian Smaug is a translational repressor that forms cytoplasmic foci similar to stress granulesThe Journal of Biological Chemistry 280:43131–43140.https://doi.org/10.1074/jbc.M508374200
-
Viral manipulation of a mechanoresponsive signaling axis disassembles processing bodiesMolecular and Cellular Biology 41:e0039921.https://doi.org/10.1128/MCB.00399-21
-
Ajuba phosphorylation by CDK1 promotes cell proliferation and tumorigenesisJournal of Biological Chemistry 291:14761–14772.https://doi.org/10.1074/jbc.M116.722751
-
Control of cellular responses to mechanical cues through YAP/TAZ regulationThe Journal of Biological Chemistry 294:17693–17706.https://doi.org/10.1074/jbc.REV119.007963
-
P-bodies and stress granules: possible roles in the control of translation and mRNA degradationCold Spring Harbor Perspectives in Biology 4:a012286.https://doi.org/10.1101/cshperspect.a012286
-
The hippo pathway in liver homeostasis and pathophysiologyAnnual Review of Pathology 16:299–322.https://doi.org/10.1146/annurev-pathol-030420-105050
-
Giving AXL the axe: targeting AXL in human malignancyBritish Journal of Cancer 116:415–423.https://doi.org/10.1038/bjc.2016.428
-
Hallmarks of cancer: new dimensionsCancer Discovery 12:31–46.https://doi.org/10.1158/2159-8290.CD-21-1059
-
The hippo pathway as a driver of select human cancersTrends in Cancer 6:781–796.https://doi.org/10.1016/j.trecan.2020.04.004
-
P-bodies: composition, properties, and functionsBiochemistry 57:2424–2431.https://doi.org/10.1021/acs.biochem.7b01162
-
The hippo pathway: biology and pathophysiologyAnnual Review of Biochemistry 88:577–604.https://doi.org/10.1146/annurev-biochem-013118-111829
-
YAP and TAZ limit cytoskeletal and focal adhesion maturation to enable persistent cell motilityThe Journal of Cell Biology 218:1369–1389.https://doi.org/10.1083/jcb.201806065
-
Cistrome Data Browser: a data portal for ChIP-Seq and chromatin accessibility data in human and mouseNucleic Acids Research 45:D658–D662.https://doi.org/10.1093/nar/gkw983
-
The hippo signaling network and its biological functionsAnnual Review of Genetics 52:65–87.https://doi.org/10.1146/annurev-genet-120417-031621
-
Control of mitochondrial structure and function by the Yorkie/YAP oncogenic pathwayGenes & Development 26:2027–2037.https://doi.org/10.1101/gad.183061.111
-
YAP/TAZ signaling and resistance to cancer therapyTrends in Cancer 5:283–296.https://doi.org/10.1016/j.trecan.2019.02.010
-
Ribosome biogenesis in cancer: new players and therapeutic avenuesNature Reviews Cancer 18:51–63.https://doi.org/10.1038/nrc.2017.104
-
The biology of YAP/TAZ: hippo signaling and beyondPhysiological Reviews 94:1287–1312.https://doi.org/10.1152/physrev.00005.2014
-
Mammalian stress granules and P bodies at a glanceJournal of Cell Science 133:science.https://doi.org/10.1242/jcs.242487
-
Hippo signalling in the liver: role in development, regeneration and diseaseNature Reviews. Gastroenterology & Hepatology 19:297–312.https://doi.org/10.1038/s41575-021-00571-w
-
Cellular organization and cytoskeletal regulation of the hippo signaling networkTrends in Cell Biology 26:694–704.https://doi.org/10.1016/j.tcb.2016.05.003
-
Preparation of hydrogel substrates with tunable mechanical propertiesCurrent Protocols in Cell Biology 47:cb1016s47.https://doi.org/10.1002/0471143030.cb1016s47
-
Emerging role of the Hippo pathway in autophagyCell Death & Disease 11:880.https://doi.org/10.1038/s41419-020-03069-6
-
MALAT1 promotes the colorectal cancer malignancy by increasing DCP1A expression and miR203 downregulationMolecular Carcinogenesis 57:1421–1431.https://doi.org/10.1002/mc.22868
-
GW182 is critical for the stability of GW bodies expressed during the cell cycle and cell proliferationJournal of Cell Science 117:5567–5578.https://doi.org/10.1242/jcs.01477
-
Dcp2 phosphorylation by Ste20 modulates stress granule assembly and mRNA decay in Saccharomyces cerevisiaeThe Journal of Cell Biology 189:813–827.https://doi.org/10.1083/jcb.200912019
-
Genome-wide association between YAP/TAZ/TEAD and AP-1 at enhancers drives oncogenic growthNature Cell Biology 17:1218–1227.https://doi.org/10.1038/ncb3216
Article and author information
Author details
Funding
National Natural Science Foundation of China (82172916)
- Chen-Ying Liu
National Natural Science Foundation of China (82073056)
- Yun Liu
National Natural Science Foundation of China (82273022)
- Long Cui
Program for Professor of Special Appointment (Eastern Scholar) at Shanghai Institutions of Higher Learning
- Chen-Ying Liu
National Natural Science Foundation of China (82372648)
- Chen-Ying Liu
The funders had no role in study design, data collection and interpretation, or the decision to submit the work for publication.
Acknowledgements
This work was sponsored by the National Key R&D Program of China (2019YFC1316002), the National Natural Science Foundation of China (82172916, 82372648, 82073056, 82273022), and the Program for Professor of Special Appointment (Eastern Scholar) at Shanghai Institutions of Higher Learning (to C-YL).
Ethics
Patients with colorectal cancer who underwent curative surgery without prior treatments at the Department of Colorectal and Anal Surgery, Xinhua Hospital, Shanghai Jiao Tong University School of Medicine, between January 2008 and December 2018 were enrolled.
All mouse studies were approved, and all animals were manipulated according to the protocols approved by the Animal Care and Use Committees of Xinhua Hospital and animal care was conducted in accordance with institutional guidelines. According to the criteria of the Animal Care and Use Committee of Xinhua Hospital, the maximal tumor burden permitted was <10% body weight, at no point did any mice exceed maximal tumor burden. Mice were housed in pathogen-free and ventilated cages, and allowed free access to irradiated food and autoclaved water ad libitum in a 12 h light/dark cycle, with room temperature at 21 °C and humidity between 45 and 65%. Male BALB/c nude mice of ~4-6 weeks of age were purchased from GemPharmatech, Shanghai, China.
Version history
- Sent for peer review:
- Preprint posted:
- Reviewed Preprint version 1:
- Reviewed Preprint version 2:
- Version of Record published:
Cite all versions
You can cite all versions using the DOI https://doi.org/10.7554/eLife.88573. This DOI represents all versions, and will always resolve to the latest one.
Copyright
© 2023, Shen, Peng, Guo et al.
This article is distributed under the terms of the Creative Commons Attribution License, which permits unrestricted use and redistribution provided that the original author and source are credited.
Metrics
-
- 1,834
- views
-
- 192
- downloads
-
- 6
- citations
Views, downloads and citations are aggregated across all versions of this paper published by eLife.
Citations by DOI
-
- 6
- citations for umbrella DOI https://doi.org/10.7554/eLife.88573