Normal and dysregulated crosstalk between iron metabolism and erythropoiesis
Figures
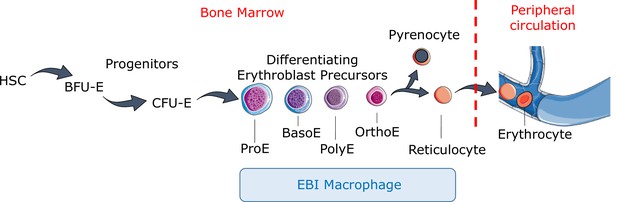
Definitive erythropoiesis.
Definitive erythropoiesis in the adult organism is derived from hematopoietic stem cells (HSCs) with progressive movement of cells through three compartments: progenitors, erythroblast precursors, and erythrocytes. Erythroid progenitors (burst-forming unit-erythroid [BFU-E], colony-forming unit-erythroid [CFU-E]) are defined by their capacity to form colonies of maturing erythroid cells in vitro. Erythroid precursors are defined as pro-erythroblasts (ProE), basophilic erythroblasts (BasoE), polychromatophilic erythroblasts (PolyE), and orthochromatic erythroblasts (OrthoE) based on morphology and progressive change in the expression of surface markers. OrthoE enucleate to form a pyrenocyte, which contains the condensed nucleus, and a reticulocyte, which goes on to mature into an erythrocyte in the peripheral circulation. Erythroblast precursors undergo differentiation in contact with a macrophage within the erythroblastic island (EBI).
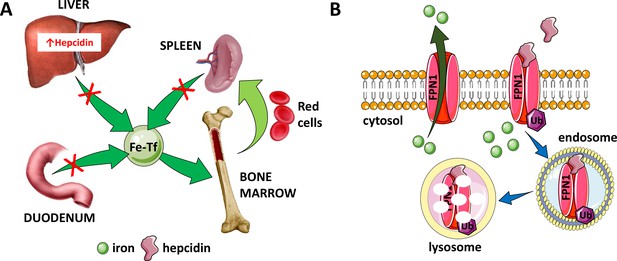
Hepcidin is central to the regulation of iron metabolism.
(A) Systemically, hepcidin is a negative regulator of iron flows such that increased hepcidin synthesis (which mainly occurs in the liver) leads to hypoferremia by decreasing iron absorption in the duodenum, iron recycling from splenic macrophages, and iron release from hepatocyte stores. (B) The mechanism of action of hepcidin involves binding to and occluding ferroportin, induction of ferroportin ubiquitination, followed by endocytosis and lysosomal degradation of the ferroportin:hepcidin complex. Fe-Tf, transferrin-bound iron; FPN1, ferroportin1; Ub, ubiquitination.
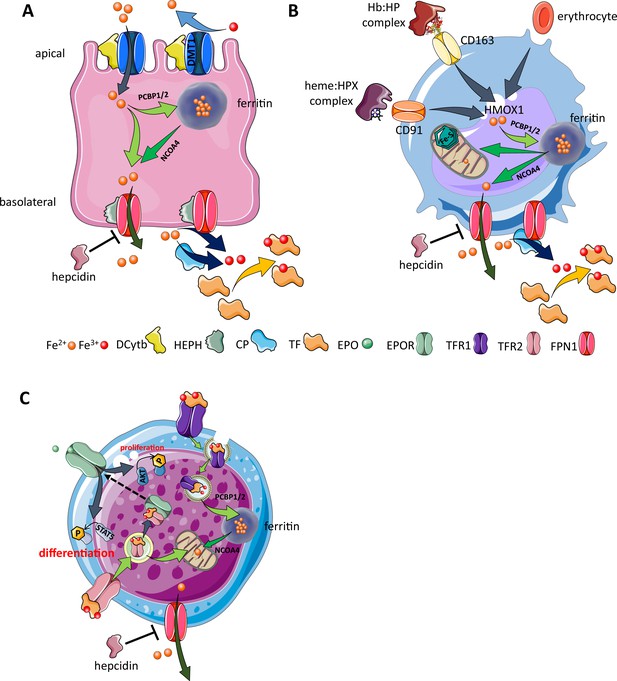
Cellular iron metabolism.
Intracellular iron homeostasis is balanced by coordinated iron uptake, utilization, storage, and export. The three main cells of interest include duodenal enterocytes (involved in systemic iron absorption), reticuloendothelial macrophages (involved in systemic iron recycling), and erythroblasts (main location of systemic iron utilization for hemoglobin synthesis during erythropoiesis). (A) Duodenal enterocyte: absorbed inorganic ferric iron (Fe3+) must be first converted to ferrous iron (Fe2+) via ferrireductase Dcytb and subsequently taken up by iron importer DMT1. Once inside the cell, iron is shuttled to ferritin via iron chaperones PCBP1/2 and stored there or shuttled out of ferritin by NCOA4 for export via FPN1. During iron export, Fe2+ must be oxidized to Fe3+ by HEPH or CP and loaded onto TF for transport in the circulation. Hepcidin prevents iron export at the basolateral cell membrane and results in ferritin iron accumulation within the enterocyte. (B) Macrophage: splenic and liver macrophages are specifically equipped with mechanisms to enable direct erythrophagocytosis, uptake of Hb:HP complexes via CD163, and heme:HPX complexes via CD91. The heme extracted from these pathways is processed by HMOX1 to liberate iron that is then either incorporated into ferritin or exported from the cell via FPN1 and loaded onto TF for delivery to iron-requiring cells. (C) Erythroblast: iron-loaded TF binds to TFR1 on the surface of cells with erythroblasts expressing the highest concentration of TFR1 relative to other cells in light of their high iron requirements. These complexes localize to clathrin-coated pits that invaginate to form specialized endosomes where proton pumps decrease the pH and transported Fe3+ is reduced by STEAP3 for export from the endosome via DMT1. Erythroblasts shuttle much of their iron to the mitochondria by an incompletely understood mechanism where it is incorporated into protoporphyrin. FPN1 is also expressed on erythroblasts but purpose of iron export in erythroblasts is incompletely understood. Finally, iron loaded TF also binds TFR2, which is thought to function as an iron sensor to coordinate iron supply with erythropoietic output by modulating EPOR localization and consequently EPO responsiveness; a detailed mechanistic understanding of TFR2’s role in erythropoiesis (DMT1, divalent metal transporter 1; Dcytb, duodenal cytochrome B reductase; FPN, ferroportin 1; HEPH, hephaestin; CP, ceruloplasmin; TF, transferrin; Fe3+, ferric iron; Fe2+, ferrous iron; Hb, hemoglobin; HP, haptoglobin; HPX, hemopexin; CD91 and 169, cluster of differentiation 91 and 163; HMOX1, heme oxygenase 1; TFR1 and 2, transferrin receptor 1 and 2; EPO, erythropoietin; EPOR, EPO receptor; PCBP1, poly(rC)-binding protein 1; NCOA4, nuclear receptor coactivator 4; pSTAT5, phosphorylated signal transducer and activator of transcription 5; pAKT, phosphorylated protein kinase B).
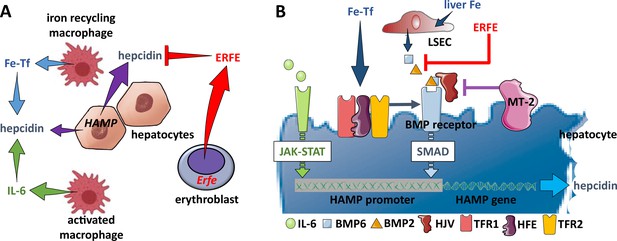
Hepcidin regulation.
(A) Systemically, hepcidin is stimulated by transferrin-bound iron in the circulation and liver iron stores as well as by systemic inflammation and suppressed by enhanced erythroid activity. (B) Regulation of hepcidin expression in the hepatocyte involves JAK-STAT signaling as a consequence of IL-6 receptor stimulation and SMAD signaling as a consequence of a BMP receptor complex stimulation. IL-6 and BMP2/6 binding their receptors, respectively, leads to stimulation of hepcidin expression. Stimulation of erythropoiesis leads to the expression and secretion of erythroferrone that sequesters BMP2/6 to suppress SMAD signaling, decreasing hepcidin. Additional coregulation via matriptase-2 and hemojuvelin as well as systemic iron sensing by TFR1, HFE, and TFR2 enhance BMP receptor stimulation and increase hepcidin expression. Fe-Tf, transferrin-bound iron; IL-6, interleukin 6; HAMP, gene name for hepcidin; ERFE, erythroferrone; LSEC, liver sinusoidal endothelial cell; TFR1/2, transferrin receptor 1 and 2; HFE, homeostatic iron regulator; HJV, hemojuvelin; MT-2, matriptase 2; JAK-STAT, janus kinase and signal transducer and activator of transcription; SMAD, small mothers against decapentaplegic.
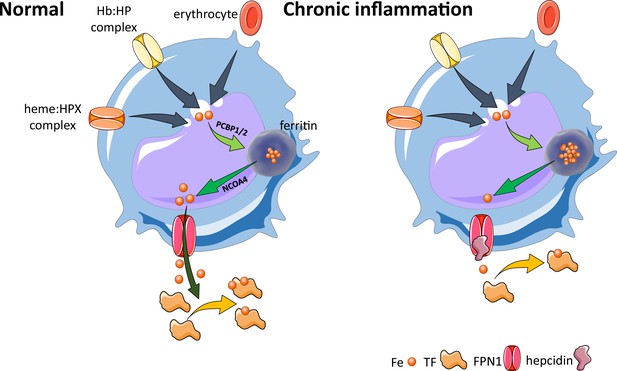
Effects of inflammation on iron recycling.
Under normal conditions, iron recycling from multiple sources within macrophages leads to export of iron via ferroportin back into the circulation where it is loaded onto transferrin and delivered to cells with iron requirements (e.g. for hemoglobin synthesis in erythroblasts during erythropoiesis in the bone marrow). Increased hepcidin in states associated with chronic inflammation lead to binding to and occlusion of the ferroportin channel, preventing iron egress from cells involved in iron recycling (e.g. splenic red pulp macrophages), leading to the accumulation of iron within cellular ferritin core, and causing decreased iron-bound transferrin (low transferrin saturation). This decreased availability of iron for erythropoiesis results in anemia of chronic inflammation. Fe, iron; FPN, ferroportin 1; TF, transferrin; Hb, hemoglobin; HP, haptoglobin; HPX, hemopexin; PCBP1, poly(rC)-binding protein 1; NCOA4, nuclear receptor coactivator 4.
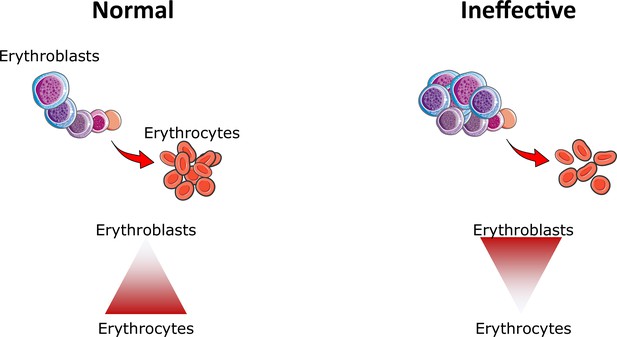
Ineffective erythropoiesis.
Under normal conditions, small numbers of differentiating erythroblasts are needed to efficiently differentiate and enucleate to reticulocytes and ultimately mature red blood cells (erythrocytes). In conditions associated with ineffective erythropoiesis (e.g. β-thalassemia, myelodysplastic syndrome, and others), a block in erythroblast differentiation leads to the accumulation of immature erythroblasts, preventing efficient production of erythrocytes, resulting in anemia.
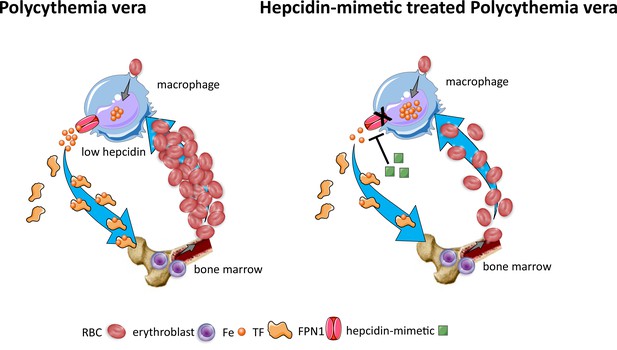
Effects of hepcidin-mimetic on erythropoiesis in polycythemia vera.
Similar to normal erythropoiesis, in polycythemia vera, iron recycling from multiple sources within macrophages leads to export of iron via ferroportin back into the circulation where it is loaded onto transferrin and delivered to cells with iron requirements (e.g. for hemoglobin synthesis in erythroblasts during erythropoiesis in the bone marrow). Unlike normal erythropoiesis, erythropoiesis proceeds despite iron deficiency and hepcidin remains low, enabling continued iron release into the circulation to support continued erythropoiesis. Increased hepcidin leads to binding to and occlusion of the ferroportin channel, preventing iron egress from cells involved in iron recycling (e.g. splenic red pulp macrophages), leading to the accumulation of iron within cellular ferritin core, and causing decreased iron-bound transferrin (low transferrin saturation). This decreased availability of iron for erythropoiesis results in reduction of erythrocytosis in polycythemia Vera. RBC, red blood cell; Fe, iron; TF, transferrin; FPN, ferroportin 1.
Tables
Novel agents in development for β-thalassemia (and other) patients.
Mechanism of action | Agent name | Producer | Stage of development | Reference |
---|---|---|---|---|
Improve RBC quality and production | Luspatercept | BMS | FDA approved for TD β-thalassemia and MDS-RS | Cappellini et al., 2023 |
Mitapivat | Agios | FDA approved for PKD; in phase II clinical trial for NTD α- and β-thalassemia patients | Kuo et al., 2022 | |
TFR2 inhibitors | Preclinical | Di Modica et al., 2022 | ||
Gene therapy to normalize the underlying genetic defect | Gene addition | Eckrich and Frangoul, 2023; Christakopoulos et al., 2023 | ||
Gene editing | ||||
Suppress erythropoiesis and prevent or reverse splenomegaly | JAK2 inhibitors | Novartis | Phase IIa (failed) | Taher et al., 2018 |
Alter iron import | Transferrin | Preclinical | Boshuizen et al., 2017 | |
Limit iron absorption | Hepcidin agonist rusfertide | Protagonist | Currently in phase II and III clinical trials for PV patients | Handa et al., 2023 |
Hepcidin inducer sapablursen | Ionis | Currently in phase II clinical trials for PV patients | Ganz et al., 2023 | |
Ferroportin inhibitor Vamifeport | Vifor | Currently in phase II clinical trials for SCD patients | Nyffenegger et al., 2022 | |
SLN124 | Silence | Currently in phase I clinical trial for β-thalassemia and PV patients | ||
ERFE inhibitors | Preclinical | Arezes et al., 2020 |
-
RBC, red blood cell; BMS, Bristol Myers Squibb; FDA, Food and Drug Administration; TD, transfusion dependent; MDS-RS, myelodysplastic syndrome with ringed sideroblasts; PKD, pyruvate kinase deficiency; NTD, non-transfusion dependent; TFR2, transferrin receptor 2; SCD, sickle cell disease; ERFE, erythroferrone.