Genetic requirement of dact1/2 to regulate noncanonical Wnt signaling and calpain 8 during embryonic convergent extension and craniofacial morphogenesis
eLife Assessment
This in several parts valuable study confirms the roles of Dact1 and Dact2, two factors involved in Wnt signaling, during zebrafish gastrulation and demonstrates their genetic interactions with other Wnt components to modulate craniofacial morphologies. Unfortunately, there are several limitations associated with the study, making it challenging to distinguish the primary and secondary effects of each factor, and their roles in craniofacial morphogenesis. The findings of a new potential target of dact1/2-mediated Wnt signaling are potentially of value; however, experimental evidence supporting their functional significance remains incomplete due to inconsistent results and limitations inherent to the overexpression approach.
https://doi.org/10.7554/eLife.91648.4.sa0Valuable: Findings that have theoretical or practical implications for a subfield
- Landmark
- Fundamental
- Important
- Valuable
- Useful
Incomplete: Main claims are only partially supported
- Exceptional
- Compelling
- Convincing
- Solid
- Incomplete
- Inadequate
During the peer-review process the editor and reviewers write an eLife Assessment that summarises the significance of the findings reported in the article (on a scale ranging from landmark to useful) and the strength of the evidence (on a scale ranging from exceptional to inadequate). Learn more about eLife Assessments
Abstract
Wnt signaling plays crucial roles in embryonic patterning including the regulation of convergent extension (CE) during gastrulation, the establishment of the dorsal axis, and later, craniofacial morphogenesis. Further, Wnt signaling is a crucial regulator of craniofacial morphogenesis. The adapter proteins Dact1 and Dact2 modulate the Wnt signaling pathway through binding to Disheveled. However, the distinct relative functions of Dact1 and Dact2 during embryogenesis remain unclear. We found that dact1 and dact2 genes have dynamic spatiotemporal expression domains that are reciprocal to one another suggesting distinct functions during zebrafish embryogenesis. Both dact1 and dact2 contribute to axis extension, with compound mutants exhibiting a similar CE defect and craniofacial phenotype to the wnt11f2 mutant. Utilizing single-cell RNAseq and an established noncanonical Wnt pathway mutant with a shortened axis (gpc4), we identified dact1/2-specific roles during early development. Comparative whole transcriptome analysis between wildtype and gpc4 and wildtype and dact1/2 compound mutants revealed a novel role for dact1/2 in regulating the mRNA expression of the classical calpain capn8. Overexpression of capn8 phenocopies dact1/2 craniofacial dysmorphology. These results identify a previously unappreciated role of capn8 and calcium-dependent proteolysis during embryogenesis. Taken together, our findings highlight the distinct and overlapping roles of dact1 and dact2 in embryonic craniofacial development, providing new insights into the multifaceted regulation of Wnt signaling.
Introduction
Wnt signaling is a crucial regulator of embryogenesis through its regulation of body axis patterning, cell fate determination, cell migration, and cell proliferation (Logan and Nusse, 2004; Steinhart and Angers, 2018; Mehta et al., 2021). Current mechanistic understanding of Wnt signaling during embryogenesis includes an extensive catalog of ligands, receptors, co-receptors, adaptors, and effector molecules (Clevers and Nusse, 2012; Niehrs, 2012; Loh et al., 2016; Mehta et al., 2021). The intricate spatiotemporal integration of Wnt signaling combinations is an important focus of developmental biology and tissue morphogenesis (Petersen and Reddien, 2009; Clevers and Nusse, 2012; Loh et al., 2016; Wiese et al., 2018). Disruptions of Wnt signaling-associated genes lead to several congenital malformations which often affect multiple organ systems given their pleotropic developmental functions (Hashimoto et al., 2014; Shi, 2022). Craniofacial anomalies are among the most common structural congenital malformations and genes in the Wnt signaling pathway are frequently implicated (Ji et al., 2019; Reynolds et al., 2019; Huybrechts et al., 2020).
Genetic approaches in zebrafish have identified a number of key Wnt regulators of early development, with gastrulation and craniofacial phenotypes (Brand et al., 1996; Hammerschmidt et al., 1996; Heisenberg et al., 1996; Piotrowski et al., 1996; Solnica-Krezel et al., 1996; Schilling and Le Pabic, 2009). The silberblick (slb) mutant, later identified as a wnt11f2 mutant allele, exhibits gastrulation and midline craniofacial phenotypes that encompassed aspects of multiple mutant classes. During early segmentation in the somite stage, the wnt11f2 mutant developed a shortened anterior–posterior axis and partially fused eyes (Heisenberg et al., 1996). Subsequently, as the cranial prominences converge and the ethmoid plate (EP) formed, instead of a fan-shaped structure observed in wildtype embryos, the wnt11f2 mutant formed a rod-like EP with a significant deficiency of the medio-lateral dimension (Heisenberg et al., 1996; Heisenberg and Nüsslein-Volhard, 1997). Another mutant knypek (kny), identified as having a nonsense mutation in gpc4, an extracellular Wnt co-receptor, was identified as a gastrulation mutant that also exhibited a shortened body axis due to a defect in embryonic convergent extension (CE) (Solnica-Krezel et al., 1996). In contrast to the slb/wnt11f2 mutant, the gpc4 mutant formed an EP that is wider in the medio-lateral dimension than the wildtype, in the opposite end of the EP phenotypic spectrum compared to wnt11f2 (Topczewski et al., 2001; Rochard et al., 2016). These observations beg the question of how defects in early patterning and CE of the embryo may be associated with later craniofacial morphogenesis. The observation that wnt11f2 and gpc4 mutant share similar CE dysfunction and axis extension phenotypes but contrasting craniofacial morphologies (Heisenberg and Nüsslein-Volhard, 1997) supports a hypothesis that CE mechanisms regulated by these Wnt pathway genes are specific to the temporal and spatial contexts during embryogenesis.
Dact (aka Frodo, Dapper) are scaffolding proteins that regulate Dishevelled (Dvl)-mediated Wnt signaling, both positively and negatively (Cheyette et al., 2002; Gloy et al., 2002; Waxman et al., 2004; Gao et al., 2008; Wen et al., 2010; Ma et al., 2015; Lee et al., 2018). Dact proteins bind directly to Dvl (Gloy et al., 2002; Brott and Sokol, 2005; Lee et al., 2018) and interact with and inhibit members of transforming growth factor beta (TGF-β) and Nodal signaling pathways (Zhang et al., 2004; Su et al., 2007; Meng et al., 2008; Kivimäe et al., 2011). In chick and Xenopus, Dact2 and Dact1 (respectively) are expressed in the neural folds during neural crest delamination and are important in epithelial–mesenchymal transition (EMT), Wnt signaling, and TGF-β signaling (Hikasa and Sokol, 2004; Schubert et al., 2014; Rabadán et al., 2016). In mouse embryos, Dact1 is expressed predominantly in mesodermal tissues, as well as ectodermal-derived tissues (Hunter et al., 2006), and ablation of Dact1 results in defective EMT and primitive streak morphogenesis, with subsequent posterior defects (Wen et al., 2010). Mouse embryonic Dact2 expression has been described in the oral epithelium and ablation of Dact2 causes increased cell proliferation (Li et al., 2013) and re-epithelialization in mice (Meng et al., 2008) and zebrafish (Kim et al., 2020).
Previous experiments using morpholinos to disrupt dact1 and dact2 in zebrafish found dact1 morphants to be slightly smaller and to develop a normal body. In contrast, dact2 morphants were found to phenocopy described zebrafish gastrulation mutants, with impaired CE, shortened body axis, and medially displaced eyes. Importantly, prior work using morpholino-mediated gene disruption of dact1 and dact2 did not examine craniofacial morphogenesis except to analyze dact1 and dact2 morphants head and eye shapes under light microscopy (Waxman et al., 2004). These experiments were carried out at a time when morpholino was the accessible tool of gene disruption (Nasevicius and Ekker, 2000; Corey and Abrams, 2001; Heasman, 2002). Since CRISPR/Cas9 targeted gene mutagenesis became popularized, many reports of germline mutant phenotypes being discrepant from prior morpholino studies warranted revisiting many of the prior work (Kok et al., 2015) and careful interpretation given the caveats of each technology (Morcos et al., 2015; Rossi et al., 2015). More recently, a zebrafish CRISPR/Cas9 genetic dact2 mutant has been generated and studied, but unlike in the dact2 morphant, no developmental phenotypes were described (Kim et al., 2020).
Here, we investigated the genetic requirement of dact1 and dact2 during embryogenesis and craniofacial development using germline mutant alleles. We found an early developmental role for dact1 and dact2 during gastrulation and body axis elongation. We also characterized the abnormal craniofacial development of the dact1/2 compound mutants. We identified distinct transcriptomic profiles of wildtype, dact1/2, and gpc4 mutants during early development, including finding calpain 8 (capn8) calcium-dependent protease to be ectopically expressed in the dact1/2 mutants. These results elaborate on the cellular roles of dact1/2 and identify capn8 as a novel regulatory candidate of embryogenesis.
Results
dact1 and dact2 have distinct expression patterns throughout embryogenesis
To determine the spatiotemporal gene expression of dact1 and dact2 during embryogenesis we performed wholemount RNA in situ hybridization (ISH) across key time points (Figure 1). Given that the described craniofacial phenotypes of the dact2 morphant and the wnt11f2 mutant are similar (Heisenberg and Nüsslein-Volhard, 1997; Waxman et al., 2004), we also performed wnt11f2 ISH to compare to dact1 and dact2 expression patterns.
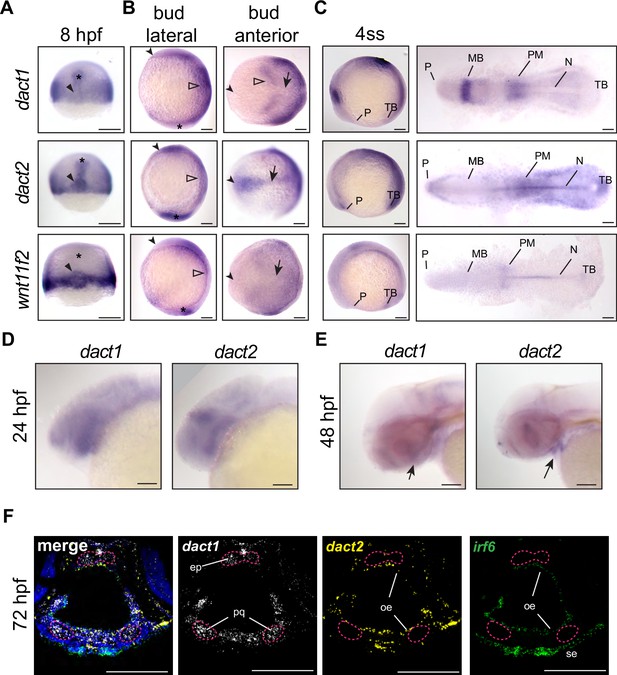
Unique and shared dact1 and dact2 gene expression domains during zebrafish development.
(A–C) Representative images of wholemount in situ hybridization showing dact1, dact2, and wnt11f2 gene expression patterns. (A) At 8 hpf, dact2 and wnt11f2 are highly expressed in the dorsal margin and presumptive Nieuwkoop center of the gastrulating embryo, with dact1 being weakly detected (arrowhead). In contrast to wnt11f2, dact1, and dact2 are expressed in the presumptive dorsal mesoderm (asterisk). (B) Lateral (anterior to the left of page) and anterior (dorsal side toward top of page) views of bud-stage embryos. dact2 and wnt11f2 transcripts are both detected in the tailbud (asterisk) while dact2 is additionally expressed in the axial mesoderm (arrow). dact1 gene expression is concentrated to the paraxial mesoderm and the neuroectoderm (open arrowheads). (C) Lateral and flat-mount views of 4 ss embryos. dact2 is expressed in the anterior neural plate and polster (P), notochord (N), paraxial and presomitic mesoderm (PM) and tailbud (TB). In contrast, dact1 is expressed in the midbrain (MB) and the paraxial and presomitic mesoderm. (D, E) Representative lateral (anterior to left of page) images of wholemount in situ hybridization showing dact1 and dact2 expression patterns. (D) At 24 hpf expression is detected in the developing head. (E) At 48 hpf expression is detected in the developing craniofacial structures (arrow). (F) Representative images of RNAscope in situ hybridization analysis of dact1 (white) and dact2 (yellow) and irf6 (green) expression in transverse section of 72 hpf embryos. dact1 is expressed in the ethmoid plate (ep) and palatoquadrate (pq) orofacial cartilage, while dact2 is expressed in the oral epithelium (oe). The epithelial marker irf6 is expressed in the oe and surface epithelium (se). Dapi (blue). Scale bar: 100 μm.
During gastrulation at 8 hours post-fertilization (hpf; 75% epiboly), some regions of dact1 and dact2 gene expression were shared and some areas are distinct to each dact gene (Figure 1A). Further, dact gene expression was distinct from wnt11f2 in that wnt11f2 expression was not detected in the presumptive dorsal mesoderm. Transcripts of dact1, dact2, and wnt11f2 were all detected in the blastoderm margin, as previously described (Makita et al., 1998; Heisenberg et al., 2000; Gillhouse et al., 2004). Transcripts of dact2, and to a lesser extent dact1, were also detected in the prechordal plate and chordamesoderm (Figure 1A). Additionally, dact2 gene expression was concentrated in the shield and presumptive organizer or Nieuwkoop center along with wnt11f2. This finding is consistent with previously described expression patterns in zebrafish and supports a role for dact1 and dact2 in mesoderm induction and dact2 in embryo dorsalization (Thisse et al., 2001; Gillhouse et al., 2004; Muyskens and Kimmel, 2007; Oteiza et al., 2010). At the end of gastrulation and during somitogenesis the differences in the domains of dact1 and dact2 gene expressions became more distinct (Figure 1B, C). At tailbud stage, dact1 transcripts were detected in the neuroectoderm and the posterior paraxial mesoderm, whereas dact2 transcripts were detected in the anterior neural plate, notochord, and tailbud. Anterior notochord and tailbud expression overlapped with wnt11f2 gene expression (Makita et al., 1998; Heisenberg et al., 2000). The expression of dact2 was unique in that its expression demarcated the anterior border of the neural plate. As dact2 morphants exhibited a craniofacial defect with medially displaced eyes and midfacial hypoplasia (Waxman et al., 2004), we examined dact1 and dact2 expression in the orofacial tissues. At 24 hpf we found some overlap but predominantly distinct expression patterns of dact1 and dact2 with dact1 being more highly expressed in the pharyngeal arches and dact2 being expressed in the midbrain/hindbrain boundary. Both dact1 and dact2 appeared to be expressed in the developing oral cavity. At 48 hpf dact1 expression is consistent with expression in the developing craniofacial cartilage elements, while dact2 expression appears within the developing mouth. The distinct cellular expression profiles of dact1 and dact2 were more clear in histological sections through the craniofacial region at 72 hpf. Utilizing RNAscope ISH, we found that dact2 and the epithelial gene irf6 were co-expressed in the surface and oral epithelium that surround the cartilaginous structures (Figure 1F). This is in contrast to dact1 which was expressed in the developing cartilage of the anterior neurocranium (ANC)/EP and palatoquadrate of the zebrafish larvae (Figure 1F).
We examined the overall expression patterns of dact1, dact2, gpc4, and wnt11f2 using Daniocell single-cell sequencing data (Farrell et al., 2018). In general, we found dact1 spatiotemporal gene expression to be more similar to gpc4 while dact2 gene expression was more similar to wnt11f2 (Figure 1—figure supplement 1). These results of shared but also distinct domains of spatiotemporal gene expression of dact1 and dact2 suggest that the dact paralogs may have some overlapping developmental functions while other roles are paralog-specific. dact1 and dact2 contribute to axis extension and dact1/2 compound mutants exhibit a CE defect dact1 and dact2 are known to interact with disheveled and regulate noncanonical Wnt signaling (Gloy et al., 2002; Waxman et al., 2004; Gao et al., 2008; Wen et al., 2010; Ma et al., 2015; Lee et al., 2018) and we have previously described the craniofacial anomalies of several zebrafish Wnt mutants (Dougherty et al., 2012; Kamel et al., 2013; Rochard et al., 2016; Ling et al., 2017; Alhazmi et al., 2021). Previous work investigated the effect of dact1 and dact2 disruption during zebrafish embryogenesis using morpholinos and reported morphant phenotypes in embryonic axis extension and eye fusion (Waxman et al., 2004). However, the limitations associated with morpholino-induced gene disruption (Kok et al., 2015; Morcos et al., 2015; Rossi et al., 2015) and the fact that craniofacial morphogenesis was not detailed for the dact1 and dact2 morphants, warranted the generation of mutant germline alleles (Figure 2—figure supplement 1). We created a dact1 mutant allele (22 bp deletion, hereafter dact1−/−) and a dact2 mutant allele (7 bp deletion, hereafter dact2−/−), both resulting in a premature stop codon and presumed protein truncation (Figure 2—figure supplement 1A, B). Gene expression of dact1 and dact2 was measured in pooled dact1−/−, dact2−/−, and dact1/2−/− embryos (Figure 2—figure supplement 1C). We found a decrease in dact1 mRNA and an increase in dact2 mRNA levels in the respective CRISPR single mutants. We hypothesize that dact2 mRNA levels are maintained or elevated in the dact2−/− mutant due to the relative 3′ position of the deletion. In the dact2−/− embryos we found a slight increase in dact1 mRNA levels, suggesting a possible compensatory effect of dact2 disruption. The specificity of the gene disruption was demonstrated by phenotypic rescue of the rod-like EP with the injection of dact1 or dact2 mRNA. Injection of dact1 mRNA or dact2 mRNA or in combination decreased the percentage of rod-like EP phenotype from near the expected 25% (35% actual) to 2–7% (Figure 2—figure supplement 1D and E).
Analysis of compound dact1 and dact2 heterozygote and homozygote alleles during late gastrulation and early segmentation time points identified embryonic axis extension anomalies (Figure 2A, B). dact1−/− or dact2−/− homozygotes develop to be phenotypically normal and viable. However, at 12 hpf, dact2−/− single mutants have a significantly shorter body axis relative to wildtype. In contrast, body length shortening phenotype was not observed in dact1−/− homozygotes. Compound heterozygotes of dact1+/-; dact2+/- also developed normally but exhibited shorter body axis relative to wildtype. The most significant axis shortening occurred in dact1−/−; dact2−/− double homozygotes with a less severe truncation phenotype in the compound heterozygote dact1+/-; dact2−/− (Figure 2A, B). Interestingly, these changes in body axis extension do not preclude the compound heterozygous larvae from reaching adulthood, except in the dact1−/−; dact2−/− double homozygotes which did not survive from larval to juvenile stages.
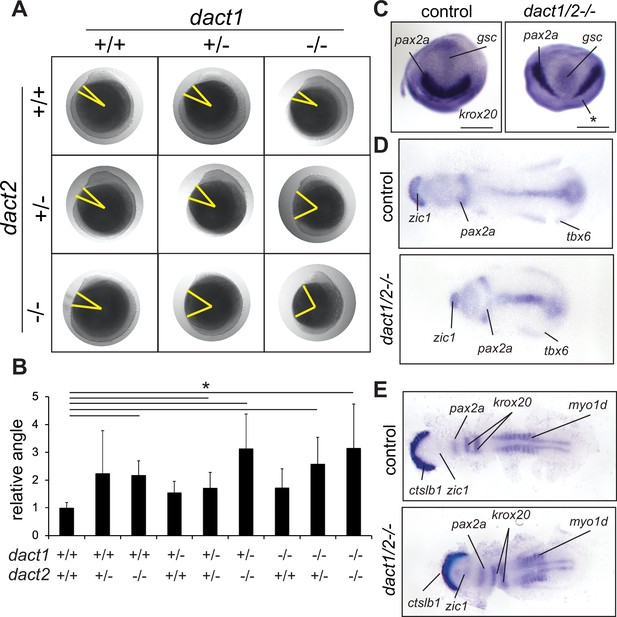
Impaired convergent extension in dact1/2 compound mutants.
(A) Inter-cross of compound heterozygotes yield embryos with different degrees of axis extension that correspond to the dact1 and dact2 genotypes. Representative lateral images of embryos at 12 hpf. The yellow line indicates body axis angle measured from the anterior point of the head, the center of the yolk, to the end of the tail. (B) Quantification of body axis angle. Numbers represent the difference in angle relative to the average wildtype embryo. Asterisk indicates genotypes with angles significantly different from wildtype. ANOVA p < 0.5 n = 3–21 embryos. Error bars: ± SEM. (C) Representative bud stage wildtype and dact1/2−/− mutant embryos stained for gsc (prechordal plate), pax2a (midbrain/hindbrain boundary), and krox20 (rhombomere 3). Asterisk indicates lack of krox20 expression in dact1/2−/− mutant. Scale bar = 200 μm (D) Representative flat mounts of 1–2 ss wildtype and dact1/2 mutant embryos stained for zic1 (telencephalon), pax2a and tbx6 (ventrolateral mesoderm). (E) Representative flat mounts of 10 ss wildtype and dact1/2−/− mutant embryos stained for ctsl1b (hatching gland), zic1, pax2a, krox20, and myo1d (somites).
Body axis truncation has been attributed to impaired CE during gastrulation (Tada and Heisenberg, 2012). To delineate CE hallmarks in the dact1−/−;dact2−/− mutants, we performed wholemount RNA ISH detecting genes that are expressed in key domains along the body axis. At bud stage, dact1−/−; dact2−/− embryos demonstrate bifurcated expression of pax2a and decreased anterior extension of gsc expression, suggesting impaired midline convergence and anterior extension of the mesoderm (Figure 2C). At the 1–2 somite stage, zic1, pax2a, and tbx6 are expressed in neural plate, prospective midbrain and the tailbud, respectively, in both the wildtype and dact1−/−; dact2−/− embryos. However, the spacing of these genes clearly revealed the shortening of the antero-posterior body axis in the dact1−/−; dact2−/− embryos. Midline convergence is decreased and the anterior border of the neural plate (marked by zic1 expression) was narrower in the dact1−/−; dact2−/− embryos (Figure 2D). At the 10-somite stage (ss), dact1−/−; dact2−/− embryos demonstrated decreased spacing between ctslb1 and pax2a gene expression, suggesting impaired lengthening of the anterior portion of the embryo. Detection of muscle marker myo1d in the dact1−/−; dact2−/− embryos delineated impaired posterior lengthening as well as reduced somitogenesis, evidenced by the decreased number of somites (Figure 2E). These data point to impaired CE of the mesoderm in dact1/2−/− double mutants, which resulted in a shorter body axis. The aberrant CE and axis extension in the dact1/2−/− phenotypes were similar to findings in other Wnt mutants, such as slb and kyn (Heisenberg et al., 2000; Topczewski et al., 2001) in that the body axis is truncated upon segmentation.
dact1/dact2 compound mutants exhibit axis shortening and craniofacial dysmorphology
Given the defective converge phenotype and shortened axis in the dact mutants during gastrulation, we examined the fish at 4 dpf for axis defects and for evidence of defective morphogenesis in the craniofacial cartilages. Craniofacial morphology is an excellent model for studying CE morphogenesis as many craniofacial cartilage elements develop through this cellular mechanism (Kamel et al., 2013; Mork and Crump, 2015; Sisson et al., 2015; Rochard et al., 2016). No craniofacial phenotype was observed in dact1 or dact2 single mutants (data not shown). However, in-crossing to generate dact1/2−/− compound homozygotes resulted in dramatic craniofacial malformation (Figure 3). Specificity of this phenotype to dact1/2 was confirmed via rescue with dact1 or dact2 mRNA injection (Figure 2—figure supplement 1D, E). The dact1/2−/− mutant embryos exhibited fully penetrant midfacial hypoplasia (Figure 3A); however, the degree of eye field convergence in the midline varied between individuals. The forebrain protruded dorsally and the mouth opening and ventral cartilage structures were displaced ventrally (Figure 3A). Alcian blue staining of cartilage elements revealed severe narrowing of the EP into a rod-like structure in 100% of double mutants, while the ventral cartilage elements were largely unaffected (Figure 3B). Notably the trabeculae extending posteriorly from the EP and the rest of the posterior neurocranium exhibit wildtype morphology in dact1/2. This dact1/2−/− double mutant phenotype is highly similar to that described for wnt11f2 (slb) mutants, a key regulator of noncanonical Wnt signaling and CE (Kimmel et al., 2001).
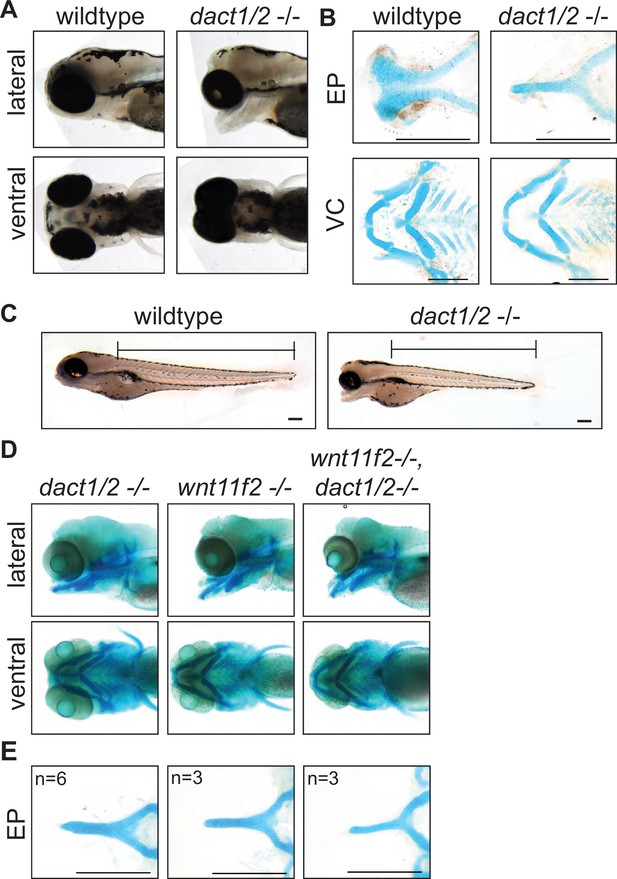
Midface development requires dact1 and dact2.
(A) Representative brightfield images of wildtype and dact1/2−/− compound mutants at 4 dpf. 100 individuals were analyzed from a dact1/2+/- double het cross. Lateral and ventral views show dact1/2−/− compound mutants have a hypoplastic midface, medially displaced eyes, and a displaced lower jaw. (B) Representative flat-mount images of Alcian blue stained ethmoid plate (EP) and visceral cartilage (VC) elements from 4 dpf wildtype and dact1/2−/− compound mutants. dact1/2−/− mutants have a rod-shaped EP with no distinct lateral and medial elements. No obvious differences were found in dact1/2 mutant VC. (C) Representative brightfield image of 4 dpf wildtype and dact1/2−/− mutant. Bar indicates vertebral spine length. Scale bar: 100 μm. (D) Representative images of Alcian blue stained dact1/2−/−, wnt11f2−/−, and wnt11f2−/−,dact1/2−/− compound mutants. Embryos resulted from a dact1+/-,dact2+/-,wnt11f2+/-in-cross. Lateral and ventral views show similar craniofacial phenotypes in each mutant. (E) Representative flat-mount images of Alcian blue stained EP show a similar phenotype between dact1/2−/−, wnt11f2−/−, and wnt11f2−/−,dact1/2−/− compound mutants. Scale bar: 200 μm.
As axis lengthening was found to be affected by loss of dact1 and dact2 (Figure 2A) we measured body length in 5 dpf dact1/2 compound mutants. Using the length of the vertebral spine as a measure of body length we found a trend (p = 0.06) toward an effect of dact1 and dact2 on shortening of the body length. Similar to axis length during gastrulation/segmentation, the shortening was most pronounced in dact1/2−/− double homozygous mutants versus wildtype clutch-mates (Figure 3C, Figure 2—figure supplement 2A, B).
As wnt11f2 signals via disheveled and since dact proteins are known to interact with disheveled (Wong et al., 2003; Zhang et al., 2006; Kivimäe et al., 2011), it is suspected that dact has a role in wnt11f2 signaling. Combinatorial gene disruption with morpholinos showed that dact2 morpholino exasperated the wnt11 morpholino midfacial/eye fusion defect (Waxman et al., 2004). We hypothesized that the shared phenotypes between wnt11f2 and dact1/2 mutants point to these genes acting in the same signaling pathway. To test for genetic epistasis between wnt11f2, dact1, and dact2 genes we generated wnt11f2/dact1/2−/− triple homozygous mutants. If wnt11f2 and dact1/2 had independent developmental requirements, the wnt11f2/dact1/2−/− mutant may exhibit a phenotype distinct from wnt11f2−/− or dact1/2−/− mutants. We found that the wnt11f2/dact1/2−/− triple homozygous mutant phenotype of the linear rod-like EP was the same as the wnt11f2−/− mutant or dact1/2−/− double mutant, without exhibiting additional or neo-phenotypes in the craniofacial cartilages or body axis (Figure 3D, E). This result supports dact1 and dact2 acting downstream of wnt11f2 signaling during ANC morphogenesis, where loss of dact1/2 function recapitulates a loss of wnt11f2 signaling.
Lineage tracing of dact1/2 mutant neural crest cell movements reveals their ANC composition
The EP forms from the convergence of a central frontal prominence-derived structure with bilateral maxillary prominence-derived elements (Wada et al., 2005; Swartz et al., 2011; Dougherty et al., 2012; Mork and Crump, 2015; Rochard et al., 2016).The stereotypic convergent migration of cranial neural crest cells (NCCs) and their derivatives presents an excellent model to examine CE movements and their effects on tissue morphogenesis. The zebrafish EP is formed from the joining of a midline frontal prominence derived from the anteromost cranial NCC population that migrate over the eyes and turn caudally, to join paired lateral maxillary prominences derived from the second stream of cranial NCC population that migrate rostrally (Kimmel et al., 2001; Wada et al., 2005; Schilling and Le Pabic, 2009; Dougherty et al., 2012; Mork and Crump, 2015). The EP that forms is a planar fan-shaped structure where we and others have shown that the morphology is governed by Wnt signaling (Kimmel et al., 2001; Rochard et al., 2016).
Given the rod-like EP we observed in the dact1/2−/− double mutants, we hypothesized that the dysmorphology could be due to aberrant migration of the anteromost midline stream of cranial NCCs resulting in fusion of the lateral maxillary components. Conversely, an abrogated contribution from the second paired stream of maxillary NCCs could lead to an EP composed entirely of the medial component. To distinguish between these possibilities, we carried out lineage tracing of the cranial NCC populations in wildtype and dact1/2 mutants. The dact1/2 compound mutants were bred onto a sox10:kaede transgenic background, where we and others have shown that the sox10 reporter is a reliable driver of cranial neural crest labeling (Wada et al., 2005; Dutton et al., 2008; Swartz et al., 2011; Dougherty et al., 2012; Kague et al., 2012; Mork and Crump, 2015). Cranial NCC populations in wildtype and dact1/2 mutants were targeted at 19 hpf to photoconvert Kaede reporter protein in either the anterior cranial NCCs that contribute to the frontal prominence, or the second stream of NCCs that contribute to the maxillary prominence, where the labeled cells were followed longitudinally over 4.5 days of development (Figure 4). We found that the anterior NCCs of wildtype embryos migrated antero-dorsally to the eye and populated the medial EP. To our surprise, the anterior cranial NCC also migrated to contribute to the median element of the rod-like EP, suggesting the complex anterior then caudal migration of the anterior NCC is not disrupted by dact1/2 mutation (Figure 4A, arrows). This finding is in contrast to lineage tracing in another midline mutant with a similarly shaped rod-like EP, the syu (sonic hedgehog null) mutant, where the anterior NCCs failed to populate the ANC (Wada et al., 2005).
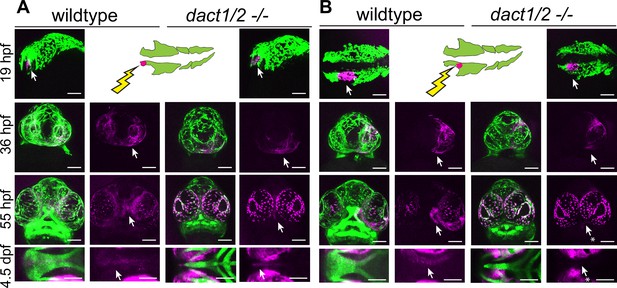
Anterior neural crest cells of the dact1/2−/− mutant migrate to the midline and populate the dysmorphic ethmoid plate.
Lineage tracing of wildtype and dact1/2−/− double mutant zebrafish embryos using Tg(sox10:kaede) line. sox10:kaede fluorescence is shown in green and photo-converted kaede is shown in magenta and highlighted with an arrow. Asterisks indicate that the cell population is absent. (A, B) 19 hpf embryo sagittal views showing photoconversion of anterior-most neural crest population. At 36 hpf frontal images show the migration of photoconverted neural crest cells to the frontal prominence in wildtype and dact1/2−/− double mutants. At 55 hpf, frontal images show photoconverted neural crest cells populating the region of the developing anterior neurocranium (ANC) in wildtype and dact1/2−/− mutants. At 4.5 dpf ventral images show photoconverted neural crest cells populating the medial ethmoid plate in wildtype. Similarly, neural crest cells in dact1/2−/− mutants populate the rod-shaped ethmoid plate. Scale bar: 100 μm. Representative images of three individual experiments.
Next, the second stream of NCC population that contribute to the maxillary prominence was labeled, where they migrate and contribute to the lateral element of the EP as expected in the wildtype (Figure 4B). When the second stream of cranial NCCs were labeled and followed in the dact1/2 mutants, the cells were found to migrate normally up to 36 hpf, but did not ultimately populate the EP in the mutant (arrows). These results suggest that NCC migration itself is not regulated by dact1/2 but that loss of dact1/2 hinders the second stream of NCCs’ ability to populate the ANC by an alternative means. Further, we have found that a rod-like EP can be formed from two different NCC origins, where in the dact1/2 mutants the EP is contributed by the anteromost frontonasal NCCs, in contrast to the similar rod-shaped EP of the syu mutants that is formed from the more posterior stream of maxillary NCCs (Wada et al., 2005).
Genetic interaction of dact1/2 with gpc4 and wls to determine facial morphology
Given the role of Dact/dapper as modifiers of Wnt signaling, we hypothesized that genetic interaction of dact1/2 with wls and gpc4 will modify facial morphology. Gpc4 is a glycoprotein that binds Wnt ligands and modulates Wnt signaling. gpc4 zebrafish mutants have impaired CE which leads to a shortened body axis (Topczewski et al., 2001). Wls is a posttranslational modifier of Wnt ligands which promotes their secretion (Bänziger et al., 2006; Bartscherer et al., 2006). We previously described that these components of the Wnt/PCP pathway (gpc4 receptor, wls intracellular ligand chaperon, and Wnt ligands wnt9a and wnt5b) are required for craniofacial morphogenesis, where each gene affects particular morphologic aspects of chondrocytes arrangement in the cardinal axis of the ANC and Meckel’s cartilage (Rochard et al., 2016; Ling et al., 2017). Using the EP as a morphologic readout, we examined the genetic interaction of dact1 and dact2 with wls and gpc4. Compound mutants of dact1, dact2, gpc4, or wls were generated by breeding the single alleles. Compared to wildtype ANC morphology, abrogation of gpc4 led to increased width in the transverse axis, but shorter in the antero-posterior axis (Rochard et al., 2016). Disruption of wls leads to ANC morphology that is also wider in the transverse dimension, but to a lesser degree than observed in gpc4. Additionally, in the wls mutant, chondrocytes stack in greater layers in the sagittal axis (Rochard et al., 2016).
Disruption of gpc4 or wls in addition to dact1/2 generated EP morphology that contained phenotypic attributes from each single mutant, so that the resultant ANC morphology represented a novel ANC form. The EP of a triple homozygous gpc4/dact1/2−/− mutant was triangular, wider in the transverse axis and shorter in the antero-posterior axis compared to the rod-like ANC observed in the dact1/2−/− double mutant (Figure 5A, B). Similarly, the ANC of a triple homozygous wls/dact1/2−/− mutant was in the shape of a rod, shorter in the antero-posterior axis and thicker in the sagittal axis compared to the dact1/2−/− double mutant, reflecting attributes of the wls mutant (Figure 5C, D). In addition to the EP phenotypes, the triple homozygous gpc4/dact1/2−/− mutant also had a short body axis and truncated tail similar but more severe than the gpc4 mutant (Figure 5A). Since compound disruption of dact1, dact2, and gpc4 or wls resulted in a new phenotype we conclude that these genes function in different components of Wnt signaling during craniofacial development.
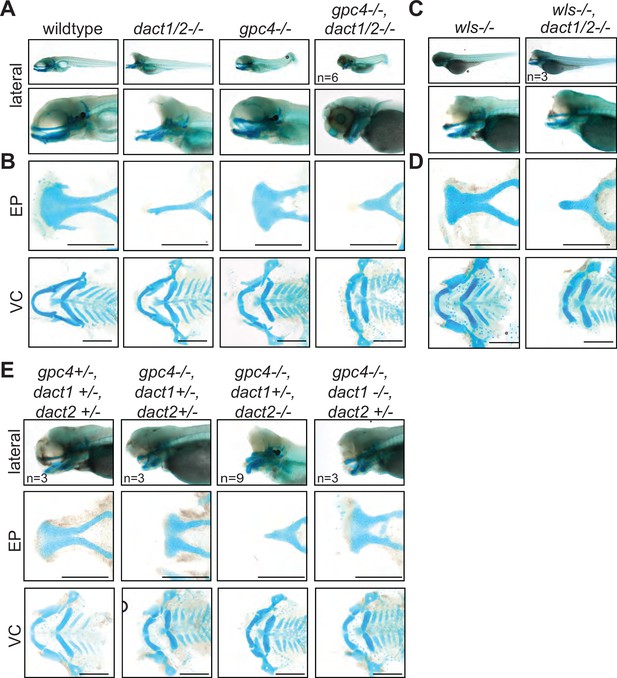
A nonoverlapping functional role for dact1, dact2, and gpc4 and wls.
(A) Representative Alcian blue stained wholemount images of wildtype, dact1/2−/− double mutant, gpc4−/− mutant, and gpc4/dact1/2−/− triple mutants at 4 dpf. Low magnification lateral images of embryos showing tail truncation in dact1/2−/− mutants, shortened and kinked tail in gpc4−/− mutants, and a combinatorial effect in gpc4/dact1/2−/− triple mutants. Higher magnification lateral images show a shortened midface and displaced lower jaw in dact1/2−/− mutants, a shortened midface in gpc4−/− mutant, and a combinatorial effect in gpc4/dact1/2−/− triple mutants. (B) Representative flat-mount images of dissected Alcian blue-stained cartilage elements. dact1/2−/− mutants have a narrow rod-shaped ethmoid plate (EP) while gpc4−/− mutants have a broad and shortened EP. dact1/2/gpc4 triple mutants have a combinatorial effect with a short, broad rod-shaped EP. In ventral cartilages (VC), dact1/2−/− mutants have a relatively normal morphology while Meckel’s cartilage in gpc4−/− mutants and gpc4/dact1/2−/− triple mutants is truncated. (C, D) Same as above except wls−/− mutant and wls/dact1/2−/− triple mutant, with similar findings. (E) Combinatorial genotypes of dact1, dact2, and gpc4. dact2−/− contributed the dact/gpc4 compound phenotype while dact1−/− did not. Scale bar: 200 μm.
As we analyzed the subsequent genotypes of our dact1/dact2/gpc4 triple heterozygote in-cross we gleaned more functional information about dact1 and dact2. We found that dact1 heterozygosity in the context of dact2−/−; gpc4−/− was sufficient to replicate the triple dact1/dact2/gpc4 homozygous phenotype (Figure 5E). In contrast, dact2 heterozygosity in the context of dact1−/−; gpc4−/− double mutant produced ANC in the opposite phenotypic spectrum of ANC morphology, appearing similar to the gpc4−/− mutant phenotype (Figure 5E). These results show that dact1 and dact2 do not have redundant function during craniofacial morphogenesis, and that dact2 function is more indispensable than dact1. These results also suggest that dact1 and gpc4 may have overlapping roles in craniofacial development.
dact1/2 and gpc4 regulate axis extension via overlapping and distinct cellular pathways
Our analyses of axis extension and the hallmarks of a CE defect (namely decreased length and increased width between early tissues) demonstrate that dact1 and/or dact2 are required for CE and anterior–posterior axis lengthening during gastrulation (Figure 3). An axis lengthening and CE defect has also been described in gpc4 (aka kny) mutants (Topczewski et al., 2001). We also observe a defect in axis lengthening in gpc4−/− in our hands (representative image Figure 6A) that is grossly similar to the dact1/2−/− mutants. Interestingly, the midfacial hypoplasia of the wnt11f2 (slb) mutant has been attributed to a defect in axis extension and anterior neural plate patterning (Heisenberg and Nüsslein-Volhard, 1997), whereas defective axis extension does not lead to midfacial hypoplasia in the gpc4−/− mutant. Therefore, we hypothesized that by comparing and contrasting the gene expression changes in dact1/2 versus gpc4 mutants during axis extension we could identify cell programs specifically responsible for the anterior axis defect and subsequent midfacial hypoplasia. We performed single-cell transcriptional analysis to compare dact1/2 mutants, gpc4 mutants, and wildtype embryos during the segmentation stage. Single-cell encapsulation and barcoded cDNA libraries were prepared from individual dissociated 4 ss wildtype, dact1/2−/− compound mutant and gpc4−/− mutant embryos using the 10X Genomics Chromium platform and Illumina next-generation sequencing. Genotyping of the embryos was not possible but quality control analysis by considering the top 2000 most variable genes across the dataset showed good clustering by genotype, indicating the reproducibility of individuals in each group. Twenty clusters were identified using Louvain clustering and identity was assigned by reviewing cluster-specific markers in light of published expression data (Farrell et al., 2018; Farnsworth et al., 2020; Bradford et al., 2022; Figure 6B, C). Qualitatively, we did not observe any significant difference in cluster abundance between genotype groups (Figure 6—figure supplement 1). We found that dact1, dact2, and gpc4 were detected at various levels across clusters, though dact1 expression was lower than dact2 (Figure 6D), consistent with what we observed in RNA wholemount ISH analysis (Figure 1).
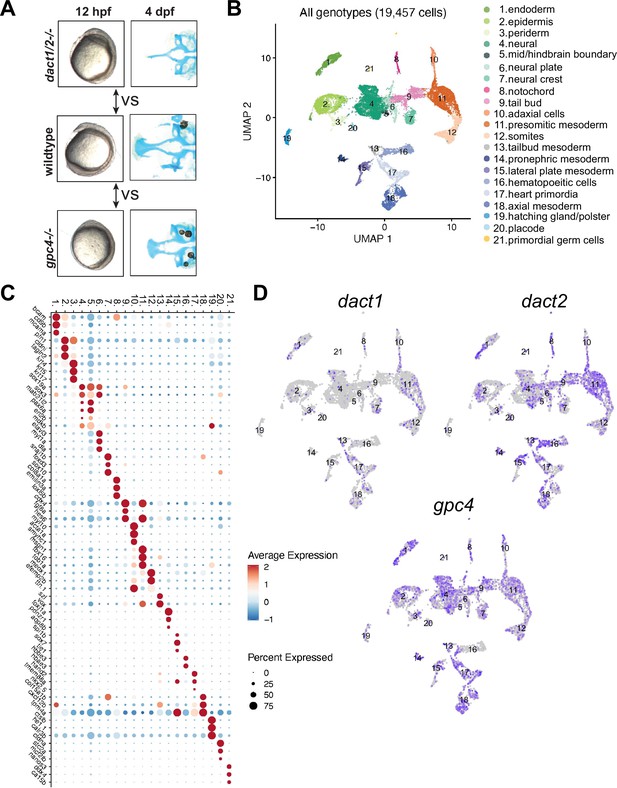
Single-cell RNAseq of 4 ss wildtype, dact1/2−/− mutant, and gpc4−/− mutants.
(A) Summary schematic showing similar phenotypes in dact1/2−/− and gpc4−/− mutants at 12 hpf and divergent phenotypes at 4 dpf. Single-cell RNAseq was performed during axis extension to compare and contrast dact1/2−/− and gpc4−/− transcriptional programs. Uniform manifold approximation and projection (UMAP) showing cluster identification. (B) UMAP of cell clusters identified by single-cell RNAseq. (C) Dot plot showing the most differentially expressed genes between clusters. (D) UMAP showing dact1, dact2, and gpc4 expression in wildtype embryos.
To assess the relative differences in gene expression between genotype groups, we merged clusters into broader cell lineages: ectoderm, axial mesoderm, and paraxial mesoderm (Figure 7A). We focused on these cell types because they contribute significantly to CE processes and axis establishment. For each of these cell lineages, we performed independent pseudo-bulk differential expression analyses (DEA) of wildtype versus dact1/2−/− mutant and wildtype vs. gpc4−/− mutant (Figure 7A). In all three cases, we found differentially expressed genes (DEGs) that were commonly in dact1−/−;dact2−/− and gpc4−/− mutant relative to wildtype (Figure 7B). To address the hypothesis that dact1 and dact2 regulate molecular pathways distinct from those regulated by gpc4 we also identified genes that were differentially expressed only in dact1/2−/− mutants or only in gpc4−/− mutants (Figure 7B). Functional analysis of these DEGs found unique enrichment of intermediate filament genes in gpc4−/− whereas dact1/2−/− mutants had enrichment for pathways associated with proteolysis (Figure 7C, Figure 7—figure supplement 1). Enrichment for pathways associated with calcium-binding were found in both gpc4−/− and dact1/2−/−, although the specific DEGs were distinct (Figure 7—figure supplement 1). We performed functional analyses specifically for genes that were differentially expressed in dact1/2−/− mutants, but not in gpc4−/− mutants, and found enrichment in pathways associated with proteolysis (Figure 7C) suggesting a novel role for Dact in embryogenesis.
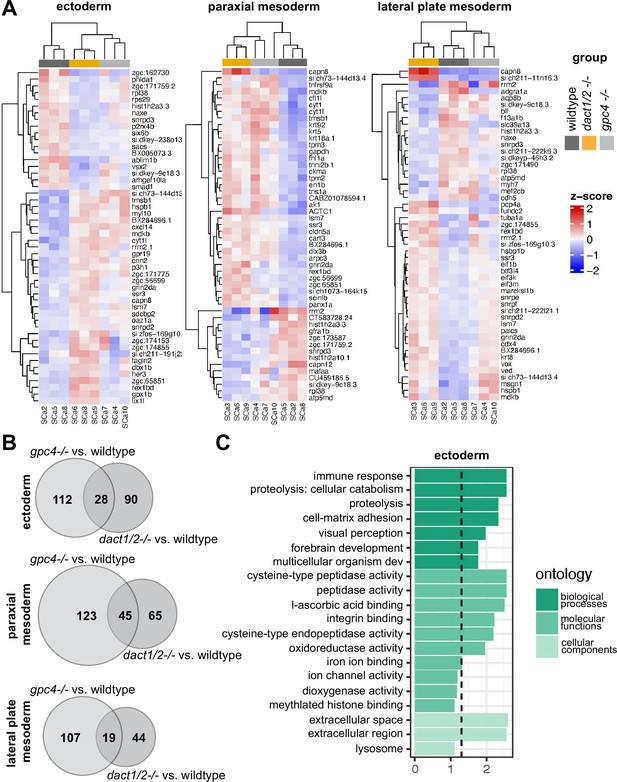
Pseudobulk differential expression analysis of single-cell RNAseq data.
(A) Heatmaps showing the 50 most differentially expressed genes (DEGs) in 3 major cell types; ectoderm (clusters 4, 5, 6, 7), paraxial mesoderm (clusters 10, 11, 12), and lateral plate mesoderm (clusters 15, 16, 17,18) between dact1/2−/− mutants and wildtype and gpc4−/− mutants and wildtype. (B) Venn diagrams showing unique and overlapping DEGs in dact1/2−/− and gpc4−/− mutants. (C) Gene Ontology (GO) analysis of dact1/2−/− mutant-specific DEGs in ectoderm showing enrichment for proteolytic processes.
Interrogation of dact1/2−/− mutant-specific DEGs found that the calcium-dependent cysteine protease calpain 8 (capn8) was significantly overexpressed in dact1/2−/− mutants in paraxial mesoderm (103-fold), axial mesoderm (33-fold), and in ectoderm (3-fold; Figure 7A). We also found that loss of dact1/2 causes significant changes to capn8 expression pattern (Figure 8A). Whereas capn8 gene expression is principally restricted to the epidermis of wildtype embryos, loss of dact1/2 leads to significant expansion of ectopic capn8 gene expression in broader cell types such as in mesodermal tissues (Figure 8A). We corroborated this finding with wholemount RNA ISH for capn8 expression in wildtype versus dact1/2−/− 12 hpf embryos (Figure 8B). The expression of smad1 was found to be decreased uniquely in the ectoderm of dact1/2−/− embryos relative to wildtype (Figure 7A), however this finding was not investigated further.
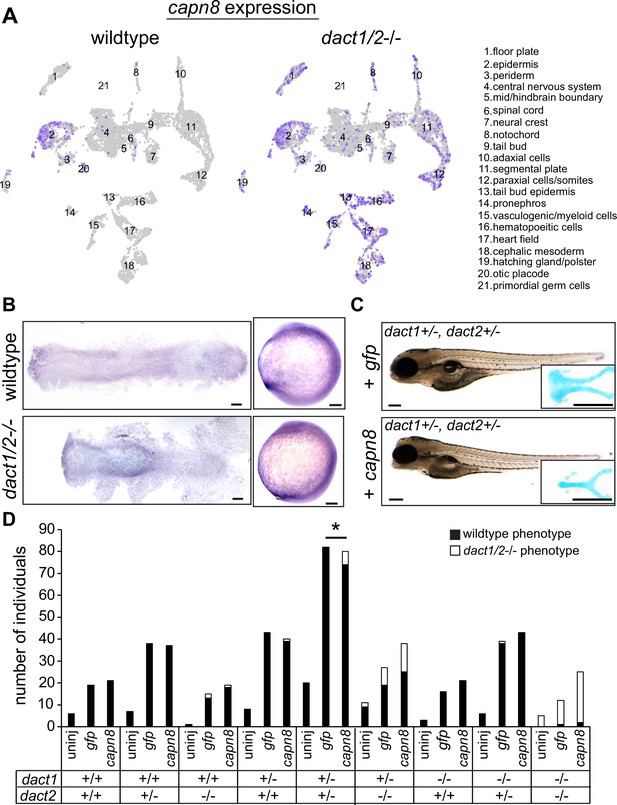
Expression of capn8 is significantly dysregulated in dact1/2−/− mutants.
(A) Single-cell RNAseq gene expression analysis of capn8 in wildtype and dact1/2−/− mutants. In wildtype embryos, capn8 expression is restricted predominantly to the epidermis whereas capn8 is widely expressed throughout the embryo in dact1/2−/− mutants, especially in the mesoderm. (B) Wholemount in situ hybridization of capn8 expression in wildtype and dact1/2−/− mutant embryos at 2 ss. Staining corroborates the single-cell RNAseq data, with expanded ectopic expression of capn8 throughout the embryo. Flat mounts are oriented anterior to the left. Scale bar: 100 μm. (C) Brightfield images and Alcian blue staining of the ethmoid plate show ectopic expression of capn8 mRNA (200 pg) at the 1 cell stage in dact1+/-,dact2+/- embryos recapitulates the dact1/2−/− compound mutant craniofacial phenotype. The mutant craniofacial phenotype did not manifest in gfp mRNA (200 pg) injected 1 cell-stage dact1+/-,dact2+/- embryos. Scale bar: 100 μm (D) Quantification of mutant and normal craniofacial phenotype in 4 dpf larvae after mRNA injection at the 1 cell stage. Larvae were derived from dact1/2+/- interbreeding. Larvae were uninjected or injected with 200 pg gfp control or capn8 mRNA. A Fisher exact test showed a significant effect of capn8 mRNA injecting in the dact1/2 double heterozygotes. Asterisk indicates a significant difference between conditions (p = 0.013).
Capn8 is considered a ‘classical’ calpain, with domain homology similar to Capn1 and Capn2 (Macqueen and Wilcox, 2014). In adult human and mouse tissue, Capn8 expression is largely restricted to the gastrointestinal tract (Sorimachi et al., 1993; Macqueen and Wilcox, 2014), however embryonic expression in mammals has not been characterized. Proteolytic targets of Capn8 have not been identified, however, other classical calpains have been implicated in Wnt and cell–cell/ECM signaling (Konze et al., 2014), including in Wnt/Ca+2 regulation of CE in Xenopus (Zanardelli et al., 2013). To determine whether the dact1/2−/− mutant craniofacial phenotype could be attributed to capn8 overexpression, we performed injection of capn8 or gfp control mRNA into 1 cell-stage zebrafish embryos. In wildtype zebrafish, exogenous capn8 mRNA caused the distinct dact1/2−/− craniofacial phenotype including a rod-like ANC at a very low frequency (1 in 142 injected embryos). This craniofacial phenotype was not observed in wildtype larvae, or when wildtype embryos were injected with an equal concentration of gfp mRNA (0 in 192 injected embryos) (data not shown). When mRNA was injected into 1 cell-stage embryos generated from dact1/2+/- interbreeding, capn8 caused a significant increase in the number of larvae with the mutant craniofacial phenotype when on a dact1/2+/- genetic background (Figure 8D, 0.0% vs. 7.5%). We did not find an effect of exogenous capn8 on any other genotype, including dact1−/−,dact2+/- which we suspect to be due to the smaller number of those individuals in our experimental population. These findings suggest a new contribution of capn8 to embryonic development as well as anterior neural plate patterning and craniofacial development. Further, the regulation of capn8 by dact may be required for normal embryogenesis and craniofacial morphogenesis.
Discussion
In this study, we examined the genetic requirement of dact1 and dact2 during early embryogenesis and craniofacial morphogenesis in zebrafish. Wnt signaling is central to the orchestration of embryogenesis and numerous proteins have been identified as modulators of Wnt signaling, including Dact1 and Dact2 (Cheyette et al., 2002). Several studies across Xenopus, zebrafish, and mouse have ascribed roles to dact1 and dact2, including both promoting and antagonizing Wnt signaling, depending on the developmental context (Cheyette et al., 2002; Gloy et al., 2002; Waxman et al., 2004; Gao et al., 2008; Wen et al., 2010; Ma et al., 2015; Lee et al., 2018). Here, we show that dact1 and dact2 are required for axis extension during gastrulation and show an example of CE defects during gastrulation associated with craniofacial defects. During axis extension, we show that genetic disruption of dact2, but not dact1, resulted in a significantly shortened axis relative to wildtype. This result is similar to what was previously found using morpholinos to disrupt dact1 and dact2. Interestingly, genetically disrupted mutants of dact1 or dact2 developed to be phenotypically normal whereas dact1/2 compound mutants displayed a severe dysmorphic craniofacial phenotype. Again, this is largely similar to the previous morpholino study that found disruption of each gene to cause only a slight and occasional dysmorphic cranial phenotype at 24 hpf (Waxman et al., 2004). Notably, embryos injected with a mixture of dact1 and dact2 morpholino were not characterized after 10 ss, and the singly injected embryos were not characterized after 24 hpf (Waxman et al., 2004). Therefore, by analyzing genetic mutants of dact1 and dact2 our findings have largely validated the previous morpholino literature as well as added new data on later developmental outcomes.
The gene expression and genetic epistasis experiments carried out here support that the dact paralogs are not redundant and have unique functions during different stages of embryonic and larval development. We observed that dact1 and dact2 have distinct spatiotemporal expression patterns throughout embryogenesis, suggesting unique roles for each paralog in developmental processes. Differential expression of Dact1 and Dact2 was also described during odontogenesis in mice (Kettunen et al., 2010). This aligns with previous findings of differential roles of dact1 and dact2 in canonical versus noncanonical Wnt signaling (Waxman et al., 2004) and a specific role for dact2, but not dact1, in TGF-β signaling (Su et al., 2007; Schubert et al., 2014). However, the lack of a resultant phenotype upon genetic ablation of dact1 or dact2 individually suggests the capability of functional compensation. This is puzzling given their distinct expression patterns and needs to be examined further.
We found that dact1 and dact2 contribute to axis extension, and their compound mutants exhibit a shortened and widened body axis that is consistent with a CE defect during gastrulation. This finding aligns with previous studies that have implicated dact1 and dact2 in noncanonical Wnt signaling and regulation of embryonic axis extension (Waxman et al., 2004). Based on our gene expression and combinatorial genetic analyses, we offer the hypothesis that dact1 expression in the paraxial mesoderm is required for dorsal CE during gastrulation through its role in noncanonical Wnt/PCP signaling, similar to the defect observed upon gpc4 disruption. Conversely, we posit that dact2 functions in the prechordal mesoderm to promote anterior migration during gastrulation, a function which has also been ascribed to wnt11f2 (Heisenberg and Nüsslein-Volhard, 1997). It is only upon loss of both dact1 and dact2 functions that the axis is significantly truncated and a craniofacial malformation results. Further experiments with spatially restricted gene ablation or cell transplantation are required to test this hypothesis.
Our results underscore the crucial roles of dact1 and dact2 in embryonic development and suggest a connection between gastrulation movements and subsequent craniofacial morphogenesis. Our finding that in dact1−/−;dact2−/− compound mutants the first stream of cranial NCC migrate and contribute to the ANC, while the second stream fails to contribute suggests the possibility of an anatomical barrier to migration, rather than an autonomous defect of the cranial NCCs. Disruption of the sonic hedgehog signaling pathway in zebrafish results in a similar phenotype to dact1/2−/− and wnt11f2−/− mutants where the eyes converge medially and the EP narrows to a rod shape. Interestingly, lineage tracing analysis in hedgehog-disrupted embryos found the rod-like EP to consist solely of second stream-derived cranial NCCs (Wada et al., 2005). This is in contrast to the dact1/2−/− mutants, demonstrating two different cellular mechanisms that result in a similar anatomical dysmorphology. It will be important to test the generality of this phenomenon and determine if other mutants with craniofacial abnormalities have early patterning differences. Further, a temporally conditional genetic knockout is needed to definitively test the connection between early and later development.
By comparing the transcriptome across different Wnt genetic contexts, that is gpc4−/− with that of the dact1/2−/− compound mutant, we identified a novel role for dact1/2 in the regulation of proteolysis, with significant misexpression of capn8 in the mesoderm of dact1/2−/− mutants. Although at a very low frequency, ectopic expression of capn8 mRNA recapitulated the dact1/2−/− mutant craniofacial phenotype, suggesting that inhibition of capn8 expression in the mesoderm by dact is required for normal morphogenesis. Genes involved in calcium ion binding were also differentially expressed in the dact1/2−/− mutants and we predict that altering intracellular calcium handling in conjunction with capn8 overexpression would increase the frequency of the recapitulated dact1/2−/− mutant phenotype.
Capn8 is described as a stomach-specific calpain and a role during embryogenesis has not been previously described. Calpains are typically calcium-activated proteases and it is feasible that Capn8 is active in response to Wnt/Ca2+ signaling. A close family member, Capn2 has been found to modulate Wnt signaling by degradation of beta-catenin (Zanardelli et al., 2013; Konze et al., 2014). Our findings suggest that dact-dependent suppression of capn8 expression is necessary for normal embryogenesis and craniofacial morphogenesis, further expanding the functional repertoire of dact1/2. Continued research is required to test a direct regulatory role of dacts on capn8 expression. While our data suggests an interaction between dact signaling and capn8 function, we did not find capn8 overexpression to be wholly sufficient to cause the rod-like EP phenotype, Further, we did not test the necessity of capn8 for craniofacial development in this study. This study does however identify capn8 as a novel embryonic gene warranting further investigation into its role during embryogenesis, with possible implications for known craniofacial or other disorders. Recently, Capn8 has been implicated in EMT associated with cancer metastasis (Zhong et al., 2022; Song et al., 2024) and Xenopus capn8 was found to be required for cranial NCC migration (Cousin et al., 2011) which further supports a role of capn8 in cranial NCC migration and craniofacial morphogenesis.
Another gene identified in our single-cell RNA sequencing data to be differentially expressed in the dact1/22−/− but not the gpc4−/− embryos was smad1. Smad1 acts in the TGF-β signaling pathway and dact2 has been described to inhibit TGF-β and Nodal signaling by promoting the degradation of Nodal receptors (Zhang et al., 2004; Su et al., 2007; Meng et al., 2008; Kivimäe et al., 2011). Zebrafish Nodal pathway mutants (cyc/ndr2, oep/tdgf1, sqt/ndr1) exhibit medially displaced eyes (Hatta et al., 1991; Brand et al., 1996; Heisenberg and Nüsslein-Volhard, 1997; Feldman et al., 1998; Zhang et al., 1998) and it is robustly feasible that dysregulation of TGF-β signaling in the dact1/2−/− mutant contributes to the craniofacial phenotype. Future research will examine the role of dact1 and dact2 in the coordination of Wnt and TGF-β signaling and the importance of this coordination in the context of craniofacial development. Of note, Sonic Hedgehog (shh) signaling is a principal regulator to the vertebrate midline (Chiang et al., 1996; Ribes et al., 2010), and important in the development of the zebrafish floorplate (Halpern et al., 1997; Odenthal et al., 2000). Mutants with disrupted shh expression or signaling (cyc/ndr2, smo, oep/tdgf1) exhibit medially displaced eyes similar to the dact1/2 mutants (Brand et al., 1996; Chen et al., 2001). We did not find any genes within the sonic hedgehog pathway to be differentially expressed in dact1/2 mutants, though post-transcriptional interactions cannot be ruled out.
This study has uncovered the genetic requirement of dact1 and dact2 in embryonic CE and craniofacial morphogenesis, delineated the genetic interaction with Wnt genes and identified capn8 as a modifier of this process. Future work will delineate the molecular differences across the different dact1/2 and other Wnt mutants to further identify determinants of craniofacial morphogenesis; and to connect these findings to clinically important Wnt regulators of facial morphology and pathology.
Materials and methods
Reagent type (species) or resource | Designation | Source or reference | Identifiers | Additional information |
---|---|---|---|---|
Strain (Danio rerio) | WT (AB) RRID:ZIRC_ZL1 | Zebrafish International Resource Center | ZDB-GENO-960809-7 | |
Strain (Danio rerio) | WT (Tubingen) RRID:NCBITaxon_7955 | Zebrafish International Resource Center | ZDB-GENO-990623-3 | |
Strain (Danio rerio) | wnt11f2 RRID:ZFIN_ZDB-GENO-200617-11 | Zebrafish International Resource Center | wnt11f2tx226/+ | |
Strain (Danio rerio) | gpc4 RRID:ZFIN_ZDB-GENO-070209-132 | Zebrafish International Resource Center | gpc4hi1688Tg/+ | |
Strain (Danio rerio) | wls | Gift. Rochard et al., 2016 PMID:27287801 | ||
Strain (Danio rerio) | sox10:kaede | Dougherty et al., 2012 PMID:22948622 | ||
Strain (Danio rerio) | dact1 | This paper | Methods: Animals and CRISPR/Cas9 targeted mutagenesis | |
Strain (Danio rerio) | dact2 | This paper | Methods: Animals and CRISPR/Cas9 targeted mutagenesis | |
Commercial kit | RNeasy Plus Mini Kit | QIAGEN | ID_source:identifier 74134 | |
Commercial kit | High Capacity cDNA Reverse Transcription Kit | Thermo Fisher | ID_source:identifier 4368814 | |
Commercial assay or kit | dact1 gene expression assay | Thermo Fisher | Dr03152516_m1 | |
Commercial assay or kit | dact2 gene expression assay | Thermo Fisher | Dr03426298_s1 | |
Commercial assay or kit | 18S rRNA gene expression assay | Thermo Fisher | Hs03003631_g1 | |
Recombinant DNA reagent | pCS2+8 destination plasmid | Addgene Gökirmak et al., 2012 PMID:23124201 | #34931 | |
Commercial assay or kit | ImMessage mMachine | Invitrogen | ID_source:identifier AM1344 | |
Commercial assay or kit | RNAscope probe dact1 | ACDbio | ID_source:identifier 857191-C2 | |
Commercial assay or kit | RNAscope probe dact2 | ACDbio | ID_source:identifier 857201-C3 | |
Commercial assay or kit | RNAscope probe irf6 | ACDbio | ID_source:identifier 555101 | |
Commercial assay or kit | Chromium Single Cell 3′ kit (version 3) | 10X Genomics | ID_source:identifier 1000268 | |
Software, algorithm | Cellranger (version 6.1.0) 10x Genomics Cellranger DNA (RRID:SCR_023221) | Zheng et al., 2017 PMID:28091601 | ||
Software, algorithm | Seurat (version 4.1.0) SEURAT (RRID:SCR_007322) | Hao et al., 2021 PMID:34062119 | ||
Software, algorithm | Harmony (version 0.1.0) Harmony (RRID:SCR_022206) | Korsunsky et al., 2019 PMID:31740819 | ||
Software, algorithm | DESeq2 (v1.34.0) DESeq2 (RRID:SCR_015687) | Love et al., 2014 PMID:25516281 | ||
Software, algorithm | clusterProfiler (version 4.2.2) clusterProfiler (RRID:SCR_016884) | Wu et al., 2021 | ||
Software, algorithm | ZiFiT Targeter v4.2 | Sander et al., 2007 PMID:17526515 | ||
Software, algorithm | ChopChop CHOPCHOP (RRID:SCR_015723) | Montague et al., 2014 PMID:24861617 |
Animals and CRISPR/Cas9 targeted mutagenesis
Request a detailed protocolAll animal husbandry and experiments were performed in accordance with and approval from the Massachusetts General Hospital Institutional Animal Care and Use Committee (protocol number 2010N000106) and the Children’s Hospital of Philadelphia Animal Care and Use Committee (protocol number IAC22001475). Zebrafish (Danio rerio) embryos and adults were maintained in accordance with institutional protocols. Embryos were raised at 28.5°C in E3 medium (Carroll et al., 2020) and staged visually and according to standardized developmental time points (Westerfield, 1993). All zebrafish lines used for experiments and gene editing were generated from the Tubingen or AB strain. The wnt11f2 mutant line and gpc4−/− mutant line were obtained from Zebrafish International Resource Center (wnt11f2tx226/+ and gpc4hi1688Tg/+, respectively). The wls−/− mutant line was originally gifted to the lab and independently generated, as previously described (Rochard et al., 2016). The sox10:kaede transgenic line was previously generated and described by our lab (Dougherty et al., 2012).
CRISPR sgRNA guides were designed using computational programs ZiFiT Targeter v4.2 (https://zifit.partners.org/ZiFit) (Sander et al., 2007), crispr.mit.edu (https://zlab.bio/guide-design-resouces) (Ran et al., 2013), and ChopChop (https://chopchop.cbu.uib.no) (Montague et al., 2014) with traditional sequence constraints. Guides were chosen that were predicted to give high efficiency and specificity. Guides best meeting these parameters were selected in exon 2 of dact1 and exon 4 of dact2. No suitable gRNA with sufficient efficiency were identified for dact2 5′ of exon 4 and the resulting phenotype was reassuring compared to previous morpholino published results. Guides for dact1 and dact2 and Cas9 protein were prepared and microinjected into 1 cell-stage zebrafish embryos and founders were identified as previously described (Carroll et al., 2020). Primers flanking the sgRNA guide site were designed for genotyping and fragment analysis was performed on genomic DNA to detect base pair insertion/deletion. Sanger sequencing was performed to verify targeted gene mutation and confirm the inclusion of a premature stop codon. dact1 forward primer: TACAGAAGCTGCTGAAGTACCG, dact1 reverse primer: CCCTCTCTCAAAGTGTTTTGGT, dact2 forward primer: TGAAGAGCTCCACTCCCCTGT, dact2 reverse primer: GCAGTTGAGGTCCATTCAGC.
RT-qPCR analysis
Request a detailed protocolPooled wildtype, dact1−/−, dact2−/−, and dact1/2−/− fish were collected and RNA extractions were performed using RNeasy Mini Kit (QIAGEN). cDNA was generated using High Capacity cDNA Reverse Transcription Kit (ThermoFisher). Quantitative PCR was performed using dact1 (Dr03152516_m1) and dact2 (Dr03426298_s1) TaqMan Gene Expression Assays. Expression was normalized to 18S rRNA expression (Hs03003631_g1). TaqMan Fast Advanced master mix (Thermo Fisher) and a StepOnePlus Real-Time PCR system (Applied Biosystems) were used to measure relative mRNA levels, which were calculated using the ddCT method.
Microinjection of mRNA
Request a detailed protocolTemplate DNA for in vivo mRNA transcription was generated by PCR amplification of the gene of interest from a zebrafish embryo cDNA library and cloning into pCS2+8 destination plasmid. mRNA for injection was generated using an in vitro transcription kit (Invitrogen mMessage mMachine). One-cell-stage zebrafish embryos were injected with 2 nl of mRNA in solution. To test genetic knockout specificity, 150 or 300 pg or dact1, dact2, or dact1 and dact2 mRNA was injected. For capn8 overexpression analysis 200 pg or GFP or capn8 mRNA was injected. Following phenotyping analysis, genotypes were determined by fragment analysis of dact1 and dact2 genotyping PCR products.
Wholemount and RNAscope ISH
Request a detailed protocolWholemount ISH was performed as previously described (Carroll et al., 2020). Zebrafish embryonic cDNA was used as a template to generate riboprobes. Primers were designed to PCR amplify the specific riboprobe sequence with a T7 promoter sequence linked to the reverse primer. In vitro transcription was performed using a T7 polymerase and DIG-labeled nucleotides (Roche). Probe primer sequences are; dact1 fwd; 5′AGCGCGATTCTCAGATGCAC3′, rev 5′gaaatTAATACGACTCACTATAggCCTGCTCGGGTTTCTGTTCA3′, dact2 fwd: 5′CAGTCGCATAGCGGATCTCAT3′, rev: 5′gaaatTAATACGACTCACTATAggGTGGACTGGGGTAACGGTAA3′, wnt11f2 fwd: 5′TCCGTGGTGTATCTTGACCG3′, rev: 5′gaaatTAATACGACTCACTATAggCTTGGTGGCCGACAGGTATT3′, pax2a fwd: 5′CCAAACCAAAAGTGGCGACG3′, rev: 5′gaaatTAATACGACTCACTATAggGTTGCTGAACCGCCAAGC3′, gsc fwd: 5′CCAGCGCCGAACTTACAATC3′, rev: 5′gaaatTAATACGACTCACTATAggTCTTCAGCTACAGCCCATTCC3′, zic1 fwd: 5′TAGGGGATCGGAGTTTGCCT3′, rev: 5′gaaatTAATACGACTCACTATAggTTCGTCAGCTGCTCTGGTTC3′, tbx6 fwd: 5′ACAGAGATCGAGATGTGCCG3′, rev: 5′gaaatTAATACGACTCACTATAggTGGAAGGGCGGTGTTCATAA3′, myo1d fwd: 5′TCTACGACGACCCTTGCTTC3′, rev: 5′gaaatTAATACGACTCACTATAggGGTCGGATTCGCCTTTTTCTG3′, ctslb1 fwd: 5′AGACCGCCTCTATGTTCGGA3′, rev: 5′gaaatTAATACGACTCACTATAggAGCGACATTAAAACGGGGGT3′, capn8 fwd: 5′AAGGGCTGGGGACAAATGAG3′, rev: 5′gaaatTAATACGACTCACTATAggCACTAGGAATGTGCAGCCGT3′.
RNAscope was performed on sectioned zebrafish larvae as previously described (Carroll et al., 2020). Probes were designed by and purchased from ACD Bio. Hybridization and detection were performed according to the manufacturer’s protocol. Sections were imaged using a confocal microscope (Leica SP8) and z-stack maximum projections were generated using Fiji software.
Alcian blue staining and imaging
Request a detailed protocolAlcian blue staining and imaging were performed at 4 dpf as previously described (Carroll et al., 2020). Briefly, larvae were fixed in 4% vol/vol formaldehyde overnight at 4°C. Larvae were dehydrated in 50% vol/vol ethanol and stained with Alcian blue as described (Walker and Kimmel, 2007). Whole and dissected larvae were imaged in 3% wt/vol methylcellulose using a Nikon Eclipse 80i compound microscope with a Nikon DS Ri1 camera. Z-stacked images were taken and extended depth of field was calculated using NIS Element BR 3.2 software. Images were processed using Fiji software. After image capture embryos were genotyped by PCR and fragment analysis.
Axis measurements
Request a detailed protocolCompound dact1+/-; dact2+/- zebrafish were in-crossed and progeny were collected from two separate clutches and fixed in 4% formaldehyde at approximately 8 ss. Embryos were individually imaged using a Zeiss Axiozoom stereoscope and processed for DNA extraction and genotyping (Westerfield, 1993). Images were analyzed using Fiji. A circle was drawn to overlay the yolk and the geometric center was determined using the function on Fiji. Using the Fiji angle tool, lines were drawn from the center point to the anterior-most point of the embryo and from the center to the posterior-most point of the embryo. The resulting inner angle of these lines was determined. Each angle measurement was then calculated as a ratio to the average angle of the wildtype embryos. All measurements were performed on images blinded for genotype. ANOVA was performed to determine statistical significance, p < 0.05.
Lineage analysis of cranial NCCs
Request a detailed protocolLive embryos were mounted in 1% wt/vol low melt agarose and covered with E3 medium containing 0.013% wt/vol tricaine. Wildtype control and dact1/2−/− compound mutants on a Tg(soxI0:kaede) background were imaged on a Leica DMi8 confocal microscope and photoconverted using the UV laser (404 nm) until the green kaede fluorescence disappeared. For each embryo, one side was photoconverted and the contralateral side served as an internal control. After photoconversion, embryos were removed from the agarose and raised in E3 medium at 28.5°C until the required developmental time point, at which time they were similarly re-mounted and re-imaged. Z-stacked images were processed as maximum-intensity projections using Fiji software.
Single-cell RNA sequencing
Request a detailed protocolSingle-cell transcriptomic analyses were performed on 10 zebrafish embryos, including 4 wildtype, 3 dact1−/−;dact2−/− compound mutant, and 3 gpc4−/− mutant embryos. Embryos were collected at 4 ss with dact1−/−,dact2−/− and gpc4−/− mutants being identified by their truncated body axis. Embryos were dechorionated with a short (approximately 10 min) incubation in 1 mg/ml Pronase and then washed 3× in embryo medium. Cell dissociation was performed with modifications as previously described (Farrell et al., 2018). Each embryo was transferred to 50 μl DMEM/F12 media on ice. To dissociate cells, media was replaced with 200 μl Dulbecco’s phosphate-buffered saline (DPBS) (without Ca2+ and Mg2+) with 0.1% wt/vol bovine serum albumin (BSA). Embryos were disrupted by pipetting 10× with a P200 pipette tip. 500 μl of DPBS + 0.1% BSA was added and cells were centrifuged at 300 × g for 1 min. Cell pellets were resuspended in 200 μl DPBS + 0.1% BSA and kept on ice. Just prior to encapsulation, cells were passed through a 40 μm cell strainer, and cell counts and viability were measured. After droplet encapsulation, barcoding, and library preparation using the 10X Genomics Chromium Single Cell 3′ kit (version 3), data were sequenced on an Illumina NovaSeq 6000 sequencer.
FASTQ files were demultiplexed and aligned to the GRCz11 build of the zebrafish genome using Cellranger (version 6.1.0) (Zheng et al., 2017). Raw Cellranger count matrices were imported into R (version 4.1.2) using Seurat (version 4.1.0) (Hao et al., 2021). First, we reviewed data for quality and excluded any droplet that did not meet all of the following criteria: (1) have at least 1500 unique molecular identifiers (UMIs); (2) covering at least 750 distinct genes; (3) have <5% of genes mapping to the mitochondrial genome; and (4) have a log10 of detected genes per UMI >80%. After quality control, the dataset was also filtered to exclude genes with a detection rate below 1 in 3000 cells, leaving a total of 20,078 distinct genes expressed across 19,457 cells for analysis.
The quality-controlled count data were normalized using Pearson’s residuals from the regularized negative binomial regression model, as implemented in Seurat::SCTransfrom (Hafemeister and Satija, 2019). When computing the SCT model, the effect of the total number of UMIs and number of detected genes per cell were regressed out. After normalization, the top 3000 most variably expressed genes were used to calculate principal components. Data were then integrated by source sample using Harmony (version 0.1.0) (Korsunsky et al., 2019). A two-dimensional uniform manifold approximation and projection (Becht et al., 2018) was then derived from the first 40 Harmony embeddings for visualization.
Using the integrated Harmony embeddings, clusters were defined with the Louvain clustering method, as implemented within Seurat. A resolution of 0.3 was used for cluster definition. Cluster identities were assigned by manually reviewing the results of Seurat::FindAllMarkers, searching for genes associated to known developmental lineages. Gene expression data for key markers that guided cluster identity assignment were visualized using Seurat::DotPlot.
Following this detailed annotation, some clusters were grouped to focus downstream analyses on three major lineages: ventral mesoderm (grouping cells from the pronephros, vasculogenic/myeloid precursors, hematopoietic cells, heart primordium, and cephalic mesoderm clusters), dorsal mesoderm (adaxial cells, segmental plate, and paraxial mesoderm), and ectoderm (CNS, mid/hindbrain boundary, spinal cord, and neural crest). For those three lineages, single-cell level data were aggregated per sample and cluster to perform pseudobulk DEA contrasting genotypes. Independent pairwise comparisons of dact1−/−;dact2−/− versus wildtype and gpc4−/− versus wildtype were performed using DESeq2 (v1.34.0) (Love et al., 2014). p-values were corrected for multiple testing using the default Benjamini–Hochberg method; log2 fold change values were corrected using the apeglm shrinkage estimator (Zhu et al., 2019). Significance was defined as an adjusted p-value <0.1 and log2 fold change >0.58 in absolute value. Heatmaps of the top most significant DEGs were generated from the regularized log transformed data using pheatmap (version 1.0.12). Overlap in significant genes across pairwise comparisons were determined and visualized in Venn diagrams. Over-representation analyses against the Gene Ontology database were ran using clusterProfiler (version 4.2.2) (Wu et al., 2021), using as input the set of genes found to be differentially expressed in the comparison of dact1−/−;dact−/− versus wildtype but not gpc4−/− versus wildtype.
Statistical analysis
Request a detailed protocolAll sample sizes represent biological replicates. Analyses were performed using Prism Software (GraphPad) unless otherwise specified. An unpaired Student’s t test or one-way ANOVA with multiple comparisons was used as indicated and a p-value <0.05 was considered significant. Graphs represent the mean ± SEM and n represents biological replicates. For categorical data (normal vs. mutant phenotype) a Fisher exact test was performed between gfp and capn8 injected embryos and the odds ratio was determined. The confidence interval was determined by the Baptista–Pike method.
Data availability
Sequencing data have been deposited in GEO under accession code GSE240264.
-
NCBI Gene Expression OmnibusID GSE240264. dact1/2 modifies noncanonical Wnt signaling and calpain 8 expression to regulate convergent extension and craniofacial development.
References
-
Dimensionality reduction for visualizing single-cell data using UMAPNature Biotechnology 01:4314.https://doi.org/10.1038/nbt.4314
-
Frodo proteins: modulators of Wnt signaling in vertebrate developmentDifferentiation; Research in Biological Diversity 73:323–329.https://doi.org/10.1111/j.1432-0436.2005.00032.x
-
An Irf6-Esrp1/2 regulatory axis controls midface morphogenesis in vertebratesDevelopment 147:24.
-
Embryonic fate map of first pharyngeal arch structures in the sox10: kaede zebrafish transgenic modelThe Journal of Craniofacial Surgery 23:1333–1337.https://doi.org/10.1097/SCS.0b013e318260f20b
-
A single-cell transcriptome atlas for zebrafish developmentDevelopmental Biology 459:100–108.https://doi.org/10.1016/j.ydbio.2019.11.008
-
Dapper1 is a nucleocytoplasmic shuttling protein that negatively modulates Wnt signaling in the nucleusThe Journal of Biological Chemistry 283:35679–35688.https://doi.org/10.1074/jbc.M804088200
-
Two Frodo/Dapper homologs are expressed in the developing brain and mesoderm of zebrafishDevelopmental Dynamics 230:403–409.https://doi.org/10.1002/dvdy.20060
-
Frodo interacts with Dishevelled to transduce Wnt signalsNature Cell Biology 4:351–357.https://doi.org/10.1038/ncb784
-
Localization and substrate selectivity of sea urchin multidrug (MDR) efflux transportersThe Journal of Biological Chemistry 287:43876–43883.https://doi.org/10.1074/jbc.M112.424879
-
Genetic interactions in zebrafish midline developmentDevelopmental Biology 187:154–170.https://doi.org/10.1006/dbio.1997.8605
-
Morpholino oligos: making sense of antisense?Developmental Biology 243:209–214.https://doi.org/10.1006/dbio.2001.0565
-
WNT signaling and bone: lessons from skeletal dysplasias and disordersFrontiers in Endocrinology 11:165.https://doi.org/10.3389/fendo.2020.00165
-
Dact1-3 mRNAs exhibit distinct expression domains during tooth developmentGene Expression Patterns 10:140–143.https://doi.org/10.1016/j.gep.2010.02.002
-
Dact2 is involved in the regulation of epithelial-mesenchymal transitionBiochemical and Biophysical Research Communications 524:190–197.https://doi.org/10.1016/j.bbrc.2019.12.090
-
Specification and morphogenesis of the zebrafish larval head skeletonDevelopmental Biology 233:239–257.https://doi.org/10.1006/dbio.2001.0201
-
The Wnt signaling pathway in development and diseaseAnnual Review of Cell and Developmental Biology 20:781–810.https://doi.org/10.1146/annurev.cellbio.20.010403.113126
-
The Wnt signaling antagonist dapper1 accelerates dishevelled2 degradation via promoting its ubiquitination and aggregate-induced autophagyThe Journal of Biological Chemistry 290:12346–12354.https://doi.org/10.1074/jbc.M115.654590
-
Zebrafish wnt11: pattern and regulation of the expression by the yolk cell and No tail activityMechanisms of Development 71:165–176.https://doi.org/10.1016/s0925-4773(98)00013-6
-
The emerging mechanisms of Wnt secretion and signaling in developmentFrontiers in Cell and Developmental Biology 9:714746.https://doi.org/10.3389/fcell.2021.714746
-
Accelerated re-epithelialization in Dpr2-deficient mice is associated with enhanced response to TGFbeta signalingJournal of Cell Science 121:2904–2912.https://doi.org/10.1242/jcs.032417
-
CHOPCHOP: a CRISPR/Cas9 and TALEN web tool for genome editingNucleic Acids Research 42:W401–W407.https://doi.org/10.1093/nar/gku410
-
Zebrafish craniofacial development: a window into early patterningCurrent Topics in Developmental Biology 115:235–269.https://doi.org/10.1016/bs.ctdb.2015.07.001
-
Tbx16 cooperates with Wnt11 in assembling the zebrafish organizerMechanisms of Development 124:35–42.
-
Effective targeted gene “knockdown” in zebrafishNature Genetics 26:216–220.https://doi.org/10.1038/79951
-
The complex world of WNT receptor signallingNature Reviews. Molecular Cell Biology 13:767–779.https://doi.org/10.1038/nrm3470
-
Genome engineering using the CRISPR-Cas9 systemNature Protocols 8:2281–2308.https://doi.org/10.1038/nprot.2013.143
-
Wnt signaling in orofacial clefts: crosstalk, pathogenesis and modelsDisease Models & Mechanisms 12:dmm037051.https://doi.org/10.1242/dmm.037051
-
Zinc Finger Targeter (ZiFiT): an engineered zinc finger/target site design toolNucleic Acids Research 35:W599–W5605.https://doi.org/10.1093/nar/gkm349
-
Fishing for the signals that pattern the faceJournal of Biology 8:101.https://doi.org/10.1186/jbiol205
-
Wnt/planar cell polarity signaling controls morphogenetic movements of gastrulation and neural tube closureCellular and Molecular Life Sciences 79:586.https://doi.org/10.1007/s00018-022-04620-8
-
A role of glypican4 and wnt5b in chondrocyte stacking underlying craniofacial cartilage morphogenesisMechanisms of Development 138 Pt 3:279–290.https://doi.org/10.1016/j.mod.2015.10.001
-
A novel tissue-specific calpain species expressed predominantly in the stomach comprises two alternative splicing products with and without Ca(2+)-binding domainThe Journal of Biological Chemistry 268:19476–19482.
-
Examination of a palatogenic gene program in zebrafishDevelopmental Dynamics 240:2204–2220.https://doi.org/10.1002/dvdy.22713
-
A two-color acid-free cartilage and bone stain for zebrafish larvaeBiotechnic & Histochemistry 82:23–28.https://doi.org/10.1080/10520290701333558
-
Loss of Dact1 disrupts planar cell polarity signaling by altering dishevelled activity and leads to posterior malformation in miceThe Journal of Biological Chemistry 285:11023–11030.https://doi.org/10.1074/jbc.M109.085381
-
BookThe Zebrafish Book: A Guide for the Laboratory Use of Zebrafish (Brachydanio Rerio)Westerfield.
-
Dapper 1 antagonizes Wnt signaling by promoting dishevelled degradationJournal of Biological Chemistry 281:8607–8612.https://doi.org/10.1074/jbc.M600274200
-
Massively parallel digital transcriptional profiling of single cellsNature Communications 8:14049.https://doi.org/10.1038/ncomms14049
Article and author information
Author details
Funding
National Institutes of Health (R01DE027983)
- Eric C Liao
Shriners Hospitals for Children
- Eric C Liao
Harvard Stem Cell Institute
- Amelie M Jule
The funders had no role in study design, data collection, and interpretation, or the decision to submit the work for publication.
Acknowledgements
We thank Christoph Seiler, Adele Donohue, and the Aqautics Facility team at Children’s Hospital of Philadelphia; and Jessica Bethoney at Massachusetts General Hospital (MGH) for their excellent management of our zebrafish colonies and facilities. We thank the MGH Next Generation Sequencing Core for cell encapsulation, cDNA library preparation, and sequencing. Single-cell sequencing analysis was performed by the Harvard Chan Bioinformatics Core. Work by AJ was funded in part by the Harvard Stem Cell Institute. We appreciate and acknowledge the generous funding support from the Shriners Hospitals for Children. This work was supported by the National Institutes of Health grant R01DE027983 to ECL.
Ethics
All animal husbandry and experiments were performed in accordance with and approval from the Massachusetts General Hospital Institutional Animal Care and Use Committee (protocol number 2010N000106) and the Children's Hospital of Philadelphia Animal Care and Use Committee (protocol number IAC22001475). Zebrafish (Danio rerio) embryos and adults were maintained in accordance with institutional protocols.
Version history
- Sent for peer review:
- Preprint posted:
- Reviewed Preprint version 1:
- Reviewed Preprint version 2:
- Reviewed Preprint version 3:
- Version of Record published:
Cite all versions
You can cite all versions using the DOI https://doi.org/10.7554/eLife.91648. This DOI represents all versions, and will always resolve to the latest one.
Copyright
© 2024, Carroll et al.
This article is distributed under the terms of the Creative Commons Attribution License, which permits unrestricted use and redistribution provided that the original author and source are credited.
Metrics
-
- 865
- views
-
- 32
- downloads
-
- 2
- citations
Views, downloads and citations are aggregated across all versions of this paper published by eLife.
Citations by DOI
-
- 1
- citation for umbrella DOI https://doi.org/10.7554/eLife.91648
-
- 1
- citation for Reviewed Preprint v2 https://doi.org/10.7554/eLife.91648.2