Human DCP1 is crucial for mRNA decapping and possesses paralog-specific gene regulating functions
Figures
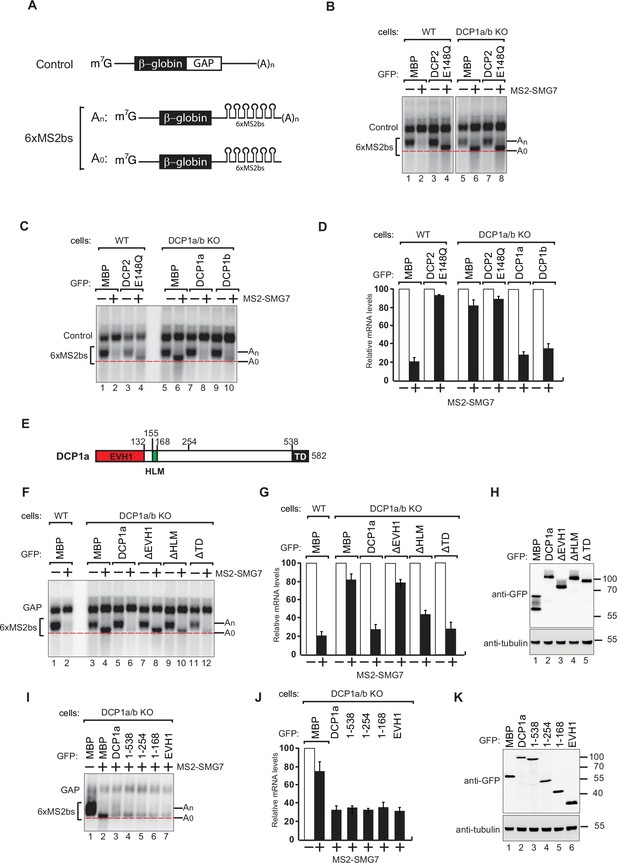
DCP1 is essential for human decapping process.
(A) Schematic of the reporters used in tethering assays. (B) Wild-type or DCP1a/b knockout HEK-293T cells were transfected with a mixture of plasmids. One plasmid expressed the β-globin-6xMS2bs, and another plasmid expressed the transfection control, which contained the β-globin gene fused to the GAPDH 3’ UTR, but it lacked the MS2 binding sites (β-globin-GAP, Control). The third plasmid expressed MS2-HA or MS2-tagged SMG7 proteins, and a fourth plasmid encoding a GFP-tagged protein was included in the transfection mixtures where indicated. A northern blot of representative RNA samples is shown. The positions of the polyadenylated (An) and the deadenylated (A0) forms of the β-globin-6xMS2bs reporters are indicated on the right. A red dotted line additionally marks the fast migrating deadenylated (A0) form. The DCP2 inactive mutant (E148Q) serves as a negative control, highlighting the importance of DCP1 in decapping by showing the absence of decapping activity without functional DCP2. All experimental results were independently repeated at least three times. (C) Complementation assays with GFP-DCP1a or GFP-DCP1b constructs in HEK-293T DCP1a/b-null cells were performed similarly to panel (A). All experimental results were independently repeated at least three times. This experiment demonstrates whether the reintroduction of DCP1a or DCP1b can restore the decapping activity in cells lacking both DCP1 isoforms. (D) The β-globin-6xMS2bs mRNA levels were normalized to those of the control mRNA. These normalized values were set to 100 in cells expressing MS2-HA (white bars). The mean values for relative mRNA levels in cells expressing MS2-SMG7 were estimated with standard deviations (SD) from three independent experiments (black bars). This quantification provides a comparative measure of the mRNA stability under different conditions. (E) The domain organization of human DCP1a. (F) Complementation assays with GFP-DCP1a deletion constructs in HEK-293T DCP1a/b-null cells performed similarly to panel (A). All experimental results were independently repeated at least three times. This experiment helps identify which domains of DCP1a are crucial for its function in the decapping process. (G) The β-globin-6xMS2bs mRNA levels were normalized to those of the control mRNA. These normalized values were set to 100 in cells expressing MS2-HA (white bars). The mean values for relative mRNA levels in cells expressing MS2-SMG7 were estimated with SDs from three independent experiments (black bars). This data provides insight into the functional importance of different DCP1a domains. (H) A western blot demonstrating equivalent expression of the GFP-tagged proteins in panel (F). Tubulin served as a loading control, confirming that the expression levels of the deletion constructs were comparable. (I) Complementation assays with GFP-DCP1a fragment constructs in HEK-293T DCP1a/b-null cells performed similarly to panel (A). All experimental results were independently repeated at least three times. This set of experiments further delineates the specific regions of DCP1a necessary for its decapping activity. (J) The β-globin-6xMS2bs mRNA levels were normalized to those of the control mRNA. These normalized values were set to 100 in cells expressing MS2-HA (white bars). The mean values for relative mRNA levels in cells expressing MS2-SMG7 were estimated with SDs from three independent experiments (black bars). This helps validate the findings regarding the essential regions of DCP1a. (K) A western blot demonstrating equivalent expression of the GFP-tagged proteins in panel (I). Tubulin served as a loading control, ensuring that the variations in mRNA levels were due to functional differences in the DCP1a fragments rather than differences in protein expression.
-
Figure 1—source data 1
Original file for the northern blot analysis in Figure 1B.
- https://cdn.elifesciences.org/articles/94811/elife-94811-fig1-data1-v1.zip
-
Figure 1—source data 2
File containing Figure 1B and original scans of the relevant northern blot analysis with highlighted bands and sample labels.
- https://cdn.elifesciences.org/articles/94811/elife-94811-fig1-data2-v1.pdf
-
Figure 1—source data 3
Original file for the northern blot analysis in Figure 1C.
- https://cdn.elifesciences.org/articles/94811/elife-94811-fig1-data3-v1.zip
-
Figure 1—source data 4
File containing Figure 1C and original scans of the relevant northern blot analysis with highlighted bands and sample labels.
- https://cdn.elifesciences.org/articles/94811/elife-94811-fig1-data4-v1.pdf
-
Figure 1—source data 5
Original file for the northern blot analysis in Figure 1F.
- https://cdn.elifesciences.org/articles/94811/elife-94811-fig1-data5-v1.zip
-
Figure 1—source data 6
File containing Figure 1F and original scans of the relevant northern blot analysis with highlighted bands and sample labels.
- https://cdn.elifesciences.org/articles/94811/elife-94811-fig1-data6-v1.pdf
-
Figure 1—source data 7
Original file for the western blot in Figure 1H.
- https://cdn.elifesciences.org/articles/94811/elife-94811-fig1-data7-v1.zip
-
Figure 1—source data 8
File containing Figure 1H and original scans of the relevant western blot analysis with highlighted bands and sample labels.
- https://cdn.elifesciences.org/articles/94811/elife-94811-fig1-data8-v1.pdf
-
Figure 1—source data 9
Original file for the northern blot analysis in Figure 1I.
- https://cdn.elifesciences.org/articles/94811/elife-94811-fig1-data9-v1.zip
-
Figure 1—source data 10
File containing Figure 1I and original scans of the relevant northern blot analysis with highlighted bands and sample labels.
- https://cdn.elifesciences.org/articles/94811/elife-94811-fig1-data10-v1.pdf
-
Figure 1—source data 11
Original file for the western blot in Figure 1K.
- https://cdn.elifesciences.org/articles/94811/elife-94811-fig1-data11-v1.zip
-
Figure 1—source data 12
File containing Figure 1K and original scans of the relevant western blot analysis with highlighted bands and sample labels.
- https://cdn.elifesciences.org/articles/94811/elife-94811-fig1-data12-v1.pdf
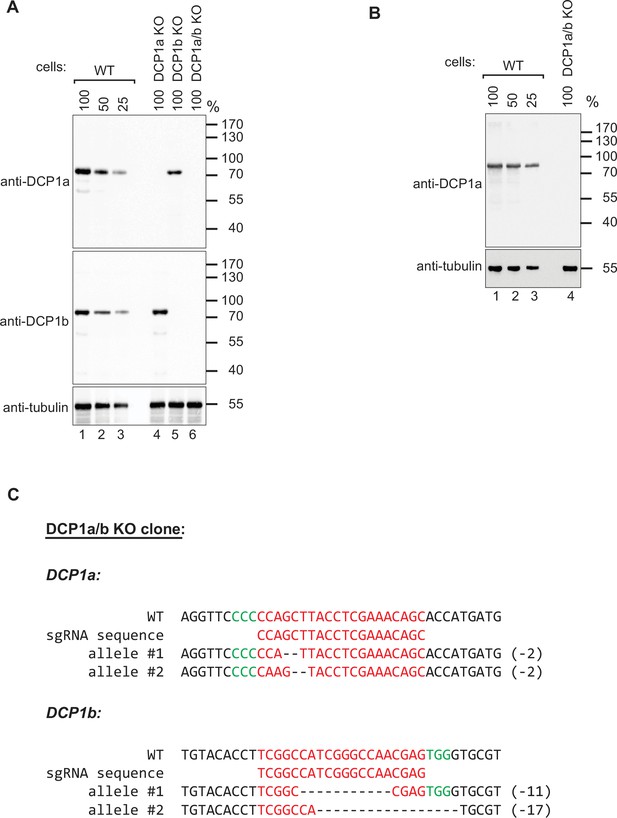
Verification of DCP1a and DCP1b knockout in HEK-293T cells.
(A) The protein levels of DCP1a and DCP1b were quantified in HEK-293T wild-type (WT) cells and DCP1a, DCP1b, and DCP1a/b knockout cell lines (DCP1a KO, DCP1b KO, and DCP1a/b KO). Western blot analysis was performed on the knockout cell clones to evaluate the expression of DCP1a and DCP1b. The Sigma D5444 antibody, which targets the C-terminal region of DCP1a, was used for the initial detection. Tubulin was used as a loading control. The results confirm the absence of full-length DCP1a and DCP1b proteins in the respective knockout cell lines. (B) To further investigate the possibility of truncated DCP1a protein expression in the DCP1a knockout cells, a western blot analysis was performed using an N-terminally targeted DCP1a antibody (aviva ARP39353_T100). This analysis aimed to detect truncated protein fragments that might be produced following the CRISPR-Cas9-mediated frameshift mutation. The results show no detectable truncated DCP1a fragments, supporting the conclusion of successful knockout. (C) Sanger sequencing of the DCP1a/b genomic region targeted by the DCP1a/b sgRNAs in the DCP1a/b knockout clone. The genome targeting led to a 2-nucleotide deletion in both alleles of DCP1a, and in DCP1b, an 11-nucleotide deletion in one allele and a 17-nucleotide deletion in the other allele. These deletions caused a frameshift in the open reading frame (ORF), resulting in the generation of premature stop codons (PTC) in both alleles of DCP1a and DCP1b. Deleted bases are indicated by (−), and the PAM sequence is highlighted in green. This sequencing data provides molecular confirmation of the knockout, demonstrating that the CRISPR/Cas9 editing led to loss-of-function mutations in DCP1a and DCP1b.
-
Figure 1—figure supplement 1—source data 1
Original file for the western blot analysis in Figure 1—figure supplement 1A.
- https://cdn.elifesciences.org/articles/94811/elife-94811-fig1-figsupp1-data1-v1.zip
-
Figure 1—figure supplement 1—source data 2
Figure 1—figure supplement 1A and original scans of the relevant western blot analysis with highlighted bands and sample labels.
- https://cdn.elifesciences.org/articles/94811/elife-94811-fig1-figsupp1-data2-v1.pdf
-
Figure 1—figure supplement 1—source data 3
Original file for the western blot analysis in Figure 1—figure supplement 1B.
- https://cdn.elifesciences.org/articles/94811/elife-94811-fig1-figsupp1-data3-v1.zip
-
Figure 1—figure supplement 1—source data 4
Figure 1—figure supplement 1B and original scans of the relevant Western blot analysis with highlighted bands and sample labels.
- https://cdn.elifesciences.org/articles/94811/elife-94811-fig1-figsupp1-data4-v1.pdf
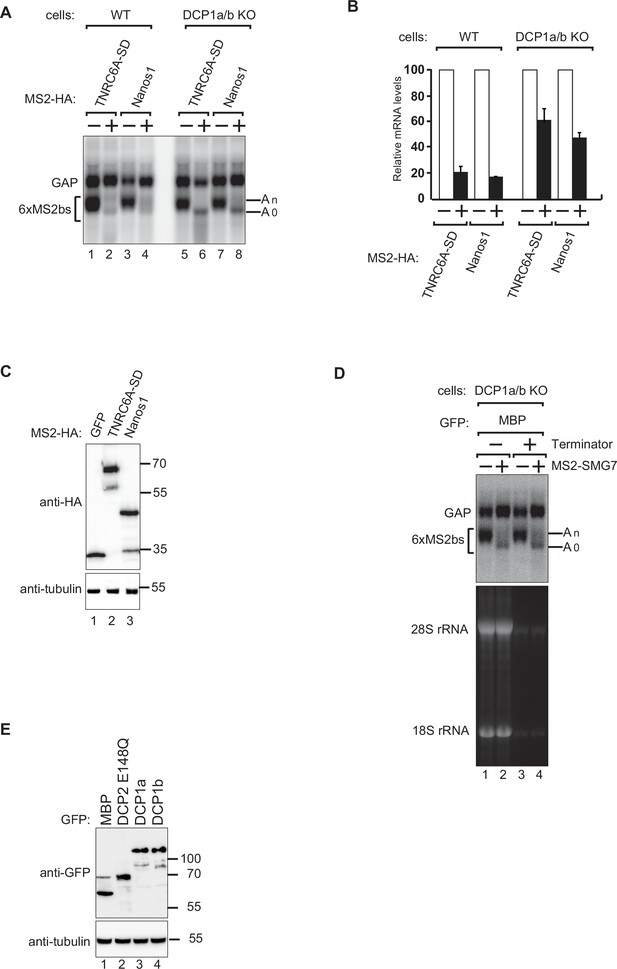
Tethering assays and RNA stability analysis in DCP1a/b knockout cells.
(A) The tethering assays using the β-globin-6xMS2bs reporter were conducted similarly to the procedure described in Figure 1A, with the exception that MS2-SMG7 was replaced by either MS2-HA-TNRC6A silencing domain (TNRC6A-SD) or MS2-HA-Nanos1 (Nanos1), as indicated. MS2-HA-GFP served as a control. All experimental results were independently repeated at least three times. This panel investigates the effect of different silencing domains on the stability of the reporter mRNA. (B) The graph shows the quantification of mRNA levels of the β-globin-6xMS2bs reporter normalized to the levels of the control reporter and set to 100 for MS2-HA-GFP. The mean values ± standard deviations (SD) are shown for three independent experiments. This quantification highlights the impact of different tethered silencing domains on mRNA degradation. (C) A representative western blot depicts the equivalent expression of the MS2-HA-tagged proteins used in (A). Tubulin served as a loading control. This panel ensures that any observed differences in mRNA levels are not due to varying expression levels of the tethered proteins. (D) The RNA samples from Figure 1A were incubated in the absence or presence of Terminator 5’-phosphate-dependent exonuclease and analyzed by northern blotting. 28S and 18S ribosomal RNA served as uncapped controls. All experimental results were independently repeated at least three times. This panel examines the susceptibility of the β-globin-6xMS2bs reporter to exonucleolytic degradation, providing insights into the cap structure and stability of the reporter mRNA under different experimental conditions. (E) A western blot demonstrating equivalent expression of the GFP-tagged proteins in Figure 1 panels (B) and (C). Tubulin served as a loading control, ensuring that any observed differences in mRNA levels were not due to variations in protein expression levels.
-
Figure 1—figure supplement 2—source data 1
Original file for the northern blot analysis in Figure 1—figure supplement 2A.
- https://cdn.elifesciences.org/articles/94811/elife-94811-fig1-figsupp2-data1-v1.zip
-
Figure 1—figure supplement 2—source data 2
File containing Figure 1—figure supplement 2A and original scans of the relevant northern blot analysis with highlighted bands and sample labels.
- https://cdn.elifesciences.org/articles/94811/elife-94811-fig1-figsupp2-data2-v1.pdf
-
Figure 1—figure supplement 2—source data 3
Original file for the western blot analysis in Figure 1—figure supplement 2C.
- https://cdn.elifesciences.org/articles/94811/elife-94811-fig1-figsupp2-data3-v1.zip
-
Figure 1—figure supplement 2—source data 4
Figure 1—figure supplement 2C and original scans of the relevant western blot analysis with highlighted bands and sample labels.
- https://cdn.elifesciences.org/articles/94811/elife-94811-fig1-figsupp2-data4-v1.pdf
-
Figure 1—figure supplement 2—source data 5
Original file for the northern blot analysis in Figure 1—figure supplement 2D.
- https://cdn.elifesciences.org/articles/94811/elife-94811-fig1-figsupp2-data5-v1.zip
-
Figure 1—figure supplement 2—source data 6
Figure 1—figure supplement 2D and original scans of the relevant northern blot analysis with highlighted bands and sample labels.
- https://cdn.elifesciences.org/articles/94811/elife-94811-fig1-figsupp2-data6-v1.pdf
-
Figure 1—figure supplement 2—source data 7
Original file for the western blot analysis in Figure 1—figure supplement 2E.
- https://cdn.elifesciences.org/articles/94811/elife-94811-fig1-figsupp2-data7-v1.zip
-
Figure 1—figure supplement 2—source data 8
Figure 1—figure supplement 2E and original scans of the relevant western blot analysis with highlighted bands and sample labels.
- https://cdn.elifesciences.org/articles/94811/elife-94811-fig1-figsupp2-data8-v1.pdf
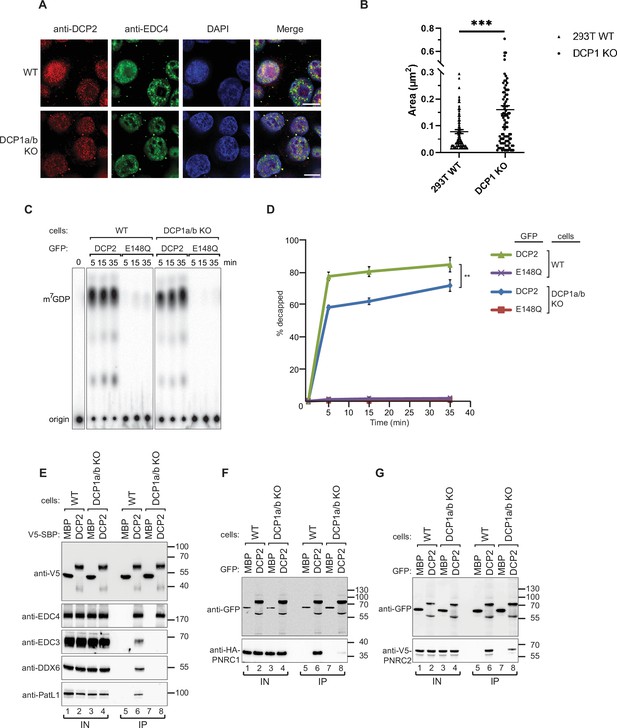
DCP1 serves as a bridging factor facilitating the interaction between multiple decapping factors and DCP2.
(A) HEK-293T wild-type or DCP1a/b-null cells were stained with antibodies detecting DCP2 (red) and a P-body marker, EDC4 (green), and then counterstained with DAPI to visualize the nucleus (blue). In the merged image, colocalization of DCP2 and EDC4 appears yellow, indicating their interaction in P-bodies. This staining helps to visualize the cellular localization of DCP2 in the presence or absence of DCP1a/b. Scale bar = 10 μm. (B) Quantification of P-body size in wild-type and DCP1a/b-null HEK-293T cells. The average granule size was measured across at least three different fields of view. The middle line represents the mean of these measurements, and p-values were calculated using unpaired t-tests (ns: not significant; *p≤0.05; **p≤0.01; ***p≤0.001). (C) GFP-tagged DCP2 proteins were expressed in human HEK-293T wild-type or DCP1a/b-null cells. Following expression, the GFP-tagged DCP2 proteins were purified using GFP antibody and IgG beads. In vitro decapping activity was then tested, with the catalytically inactive DCP2 E148Q mutant serving as a negative control to demonstrate the specificity of the decapping activity. All experimental results were independently repeated at least three times. (D) Decapping assays in vitro were conducted to measure the fraction of decapped mRNA substrate by detecting the release of m7GDP over time. The results are plotted as a function of time, with error bars representing the standard deviations (SD) from three independent experiments. An unpaired t-test was used to evaluate the statistical difference between samples (ns: not significant; *p≤0.05; **p≤0.01; ***p≤0.001). This panel demonstrates the decapping efficiency of DCP2 in the presence or absence of DCP1a/b. (E) V5-Streptavidin-Binding Peptide (SBP)-DCP2 proteins were expressed in human HEK-293T wild-type or DCP1a/b-null cells, followed by purification using Streptavidin beads. This experiment examines the interaction of V5-SBP-tagged DCP2 with endogenous decapping factors in wild-type or DCP1a/b knockout HEK-293T cells. The bound proteins were detected via western blot, with V5-SBP-MBP employed as a negative control. All experimental results were independently repeated at least three times. This panel highlights the role of DCP1a/b in facilitating or stabilizing interactions between DCP2 and other decapping factors. (F, G) The interaction of GFP-tagged DCP2 with HA-tagged PNRC1 (F) or V5-tagged PNRC2 (G) was assessed. The proteins were immunoprecipitated using anti-GFP antibodies and analyzed by western blotting with the indicated antibodies. All experimental results were independently repeated at least three times. These panels demonstrate the interaction between DCP2 and specific decapping co-factors, indicating how DCP1a/b may influence these interactions.
-
Figure 2—source data 1
Original file for the decapping assays in Figure 2C.
- https://cdn.elifesciences.org/articles/94811/elife-94811-fig2-data1-v1.zip
-
Figure 2—source data 2
Figure 2C and original scans of the relevant decapping assays with highlighted bands and sample labels.
- https://cdn.elifesciences.org/articles/94811/elife-94811-fig2-data2-v1.pdf
-
Figure 2—source data 3
Original file for the western blot in Figure 2E.
- https://cdn.elifesciences.org/articles/94811/elife-94811-fig2-data3-v1.zip
-
Figure 2—source data 4
Figure 2E and original scans of the relevant western blot analysis with highlighted bands and sample labels.
- https://cdn.elifesciences.org/articles/94811/elife-94811-fig2-data4-v1.pdf
-
Figure 2—source data 5
Original file for the western blot in Figure 2F.
- https://cdn.elifesciences.org/articles/94811/elife-94811-fig2-data5-v1.zip
-
Figure 2—source data 6
Figure 2F and original scans of the relevant western blot analysis with highlighted bands and sample labels.
- https://cdn.elifesciences.org/articles/94811/elife-94811-fig2-data6-v1.pdf
-
Figure 2—source data 7
Original file for the western blot in Figure 2G.
- https://cdn.elifesciences.org/articles/94811/elife-94811-fig2-data7-v1.zip
-
Figure 2—source data 8
Figure 2G and original scans of the relevant western blot analysis with highlighted bands and sample labels.
- https://cdn.elifesciences.org/articles/94811/elife-94811-fig2-data8-v1.pdf
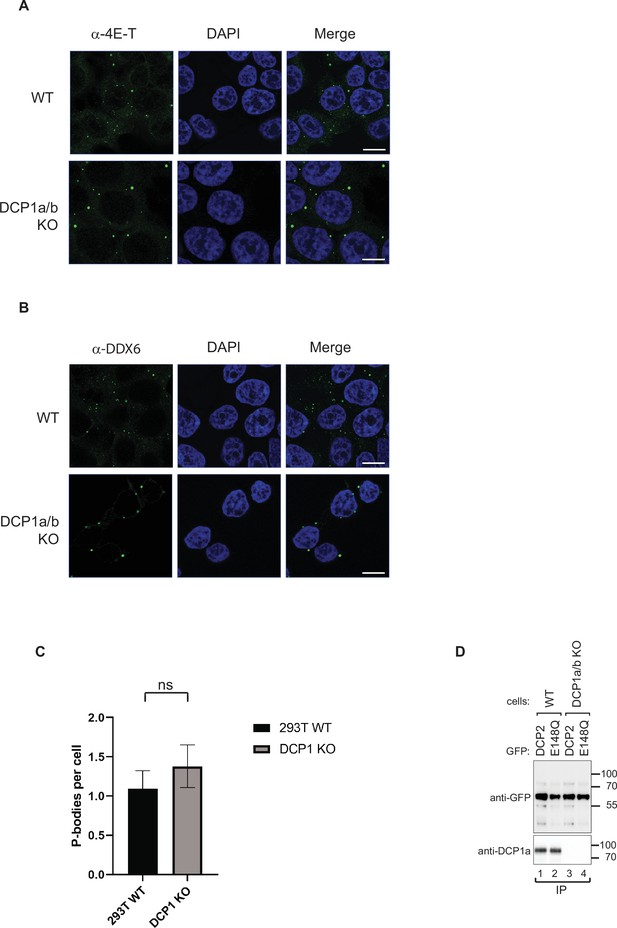
Colocalization of DCP2 with P-body markers in wild-type and DCP1a/b-null HEK-293T cells.
(A) HEK-293T wild-type and DCP1a/b-null cells were stained using antibodies that detect DCP2 (red) and the P-body marker 4E-T (green). Cells were counterstained with DAPI to visualize the nucleus (blue). The merged images show colocalization of DCP2 and 4E-T, indicated by yellow regions. Scale bar = 10 μm. This panel illustrates the distribution and colocalization of DCP2 with the P-body marker 4E-T in both wild-type and knockout cells. (B) HEK-293T wild-type and DCP1a/b-null cells were stained using antibodies that detect DCP2 (red) and the P-body marker DDX6 (green). Cells were counterstained with DAPI to visualize the nucleus (blue). The merged images show colocalization of DCP2 and DDX6, indicated by yellow regions. Scale bar = 10 μm. This panel demonstrates the distribution and colocalization of DCP2 with the P-body marker DDX6 in both wild-type and knockout cells. (C) Quantification of P-body granules in wild-type and DCP1a/b-null cells. The average number of foci per cell was measured across at least three different fields of view. The solid bar indicates the mean values ± standard deviations (SD) of the replicates. An unpaired t-test was used to evaluate the statistical difference between samples (ns: not significant; *p≤0.05; **p≤0.01; ***p≤0.001). (D) The GFP-DCP2 WT or E148Q immunoprecipitated samples corresponding to Figure 2C were analyzed by western blotting using the indicated antibodies. This analysis confirms the presence and proper expression of the GFP-DCP2 proteins and their catalytic activity.
-
Figure 2—figure supplement 1—source data 1
Original file for the western blot analysis in Figure 2—figure supplement 1D.
- https://cdn.elifesciences.org/articles/94811/elife-94811-fig2-figsupp1-data1-v1.zip
-
Figure 2—figure supplement 1—source data 2
Figure 2—figure supplement 1D and original scans of the relevant western blot analysis with highlighted bands and sample labels.
- https://cdn.elifesciences.org/articles/94811/elife-94811-fig2-figsupp1-data2-v1.pdf
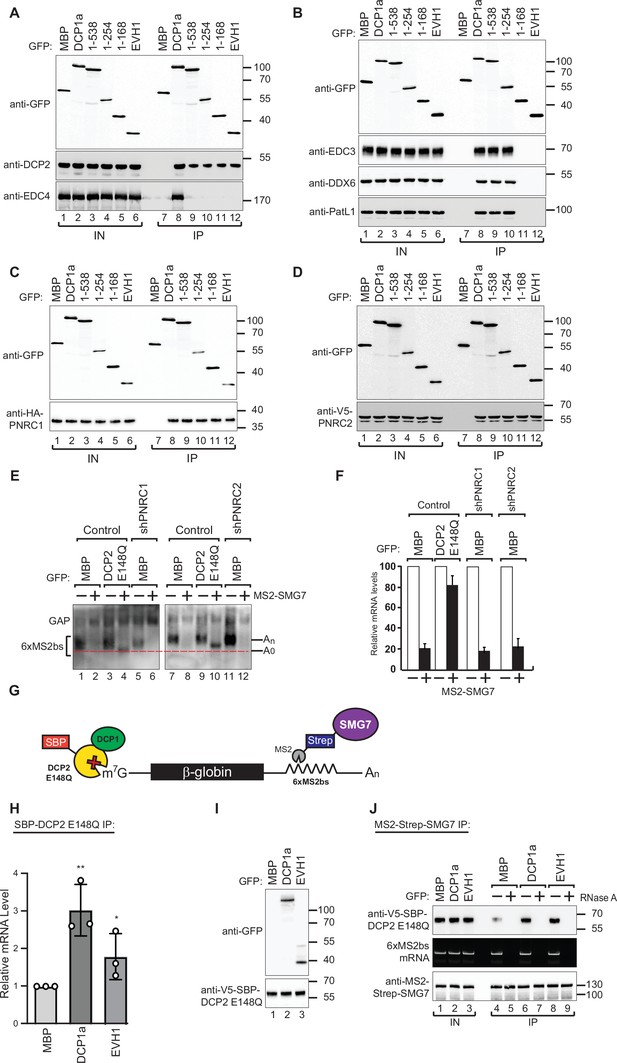
DCP1 facilitates DCP2 interactions with RNA molecules in human cells.
(A–D) The interaction of GFP-tagged DCP2 with various decapping factors was examined. Proteins were immunoprecipitated using anti-GFP antibodies and analyzed by western blotting as described in Figure 2D and E. All experimental results were independently repeated at least three times. These panels demonstrate the binding affinity and specificity of DCP2 for different decapping co-factors, highlighting the importance of these interactions in the decapping process. (E) Tethering assays were performed in HEK-293T cells using control, shPNRC1, or shPNRC2 plasmids, following a procedure similar to that described in Figure 1A. All experimental results were independently repeated at least three times. This panel investigates the effect of knocking down PNRC1 or PNRC2 on the decapping activity, showing how these factors contribute to the stability and regulation of the β-globin-6xMS2bs mRNA. (F) The β-globin-6xMS2bs mRNA levels were normalized to those of the control mRNA. These normalized values were set to 100 in cells expressing MS2-HA (white bars). The mean values for relative mRNA levels in cells expressing MS2-SMG7 were estimated with standard deviations (SD) from three independent experiments (black bars). This quantification provides a comparative measure of the mRNA stability under different experimental conditions. (G) Schematic representation of the experimental procedure used in panels (H) and (J). This diagram outlines the steps and components involved in the transfection and subsequent analysis, providing a visual aid for understanding the experimental setup. (H) DCP1a/b-null HEK-293T cells were transfected with a mixture of plasmids, including β-globin-6xMS2bs, MS2-HA-Strep-SMG7, V5-SBP-DCP2 E148Q, and either full-length or the EVH1 domain of GFP-DCP1a. GFP-MBP was used as a control. The levels of β-globin-6xMS2bs mRNA bound to V5-SBP-DCP2 E148Q were immunoprecipitated using streptavidin beads and quantified by RT-PCR with GAPDH as a reference. The results represent three biological replicates' mean values ± SD. An unpaired t-test was used to evaluate the statistical difference between samples (ns: not significant; *p≤0.05; **p≤0.01; ***p≤0.001). This experiment assesses the role of different DCP1a constructs in the interaction and binding efficiency of DCP2 to the target mRNA. (I) The V5-SBP-DCP2 E148Q immunoprecipitated samples corresponding to panel (H) were analyzed by western blotting using the indicated antibodies. This panel confirms the tagged proteins' expression and proper immunoprecipitation, ensuring the RT-PCR results' validity in panel (H). (J) The plasmid mixture described in panel (H) was transfected into DCP1a/b-null HEK-293T cells. Subsequently, Strep-tag beads were used to immunoprecipitate the MS2-HA-Strep-SMG7 bound β-globin-6xMS2bs mRNA to study the in vivo interaction levels between RNA molecules and DCP2 E148Q in the presence of either full-length GFP-DCP1a or its EVH1 domain. GFP-MBP served as a control. All experimental results were independently repeated at least three times. This panel explores how different domains of DCP1a influence the interaction between DCP2 and its RNA targets, providing insights into the functional domains required for effective decapping.
-
Figure 3—source data 1
Original file for the western blot in Figure 3A.
- https://cdn.elifesciences.org/articles/94811/elife-94811-fig3-data1-v1.zip
-
Figure 3—source data 2
Figure 3A and original scans of the relevant western blot with highlighted bands and sample labels.
- https://cdn.elifesciences.org/articles/94811/elife-94811-fig3-data2-v1.pdf
-
Figure 3—source data 3
Original file for the western blot in Figure 3B.
- https://cdn.elifesciences.org/articles/94811/elife-94811-fig3-data3-v1.zip
-
Figure 3—source data 4
Figure 3B and original scans of the relevant western blot analysis with highlighted bands and sample labels.
- https://cdn.elifesciences.org/articles/94811/elife-94811-fig3-data4-v1.pdf
-
Figure 3—source data 5
Original file for the western blot in Figure 3C.
- https://cdn.elifesciences.org/articles/94811/elife-94811-fig3-data5-v1.zip
-
Figure 3—source data 6
Figure 3C and original scans of the relevant western blot analysis with highlighted bands and sample labels.
- https://cdn.elifesciences.org/articles/94811/elife-94811-fig3-data6-v1.pdf
-
Figure 3—source data 7
Original file for the western blot in Figure 3D.
- https://cdn.elifesciences.org/articles/94811/elife-94811-fig3-data7-v1.zip
-
Figure 3—source data 8
Figure 3D and original scans of the relevant western blot analysis with highlighted bands and sample labels.
- https://cdn.elifesciences.org/articles/94811/elife-94811-fig3-data8-v1.pdf
-
Figure 3—source data 9
Original file for the northern blot analysis in Figure 3E.
- https://cdn.elifesciences.org/articles/94811/elife-94811-fig3-data9-v1.zip
-
Figure 3—source data 10
Figure 3E and original scans of the relevant northern blot analysis with highlighted bands and sample labels.
- https://cdn.elifesciences.org/articles/94811/elife-94811-fig3-data10-v1.pdf
-
Figure 3—source data 11
Original file for the western blot in Figure 3I.
- https://cdn.elifesciences.org/articles/94811/elife-94811-fig3-data11-v1.zip
-
Figure 3—source data 12
Figure 3I and original scans of the relevant western blot with highlighted bands and sample labels.
- https://cdn.elifesciences.org/articles/94811/elife-94811-fig3-data12-v1.pdf
-
Figure 3—source data 13
Original file for the western blot in Figure 3J.
- https://cdn.elifesciences.org/articles/94811/elife-94811-fig3-data13-v1.zip
-
Figure 3—source data 14
Figure 3J and original scans of the relevant western blot with highlighted bands and sample labels.
- https://cdn.elifesciences.org/articles/94811/elife-94811-fig3-data14-v1.pdf
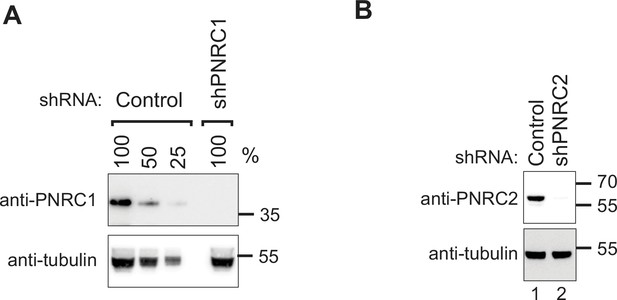
Validation of PNRC1 and PNRC2 knockdown in HEK-293T cells.
(A) HEK-293T cells were transfected with shRNA plasmid sequences targeting PNRC1 to induce RNAi-mediated silencing. As a negative control, cells were also transfected with a non-targeting shRNA. Protein levels of PNRC1 were subsequently detected using western blot analysis. This panel shows the effectiveness of shRNA-mediated knockdown of PNRC1 compared to the control. (B) HEK-293T cells were transfected with shRNA plasmid sequences targeting PNRC2 to induce RNAi-mediated silencing. As a negative control, cells were also transfected with a non-targeting shRNA. Protein levels of PNRC2 were subsequently detected using western blot analysis. This panel demonstrates the effectiveness of shRNA-mediated knockdown of PNRC2 compared to the control.
-
Figure 3—figure supplement 1—source data 1
Original file for the western blot analysis in Figure 3—figure supplement 1A.
- https://cdn.elifesciences.org/articles/94811/elife-94811-fig3-figsupp1-data1-v1.zip
-
Figure 3—figure supplement 1—source data 2
Figure 3—figure supplement 1A and original scans of the relevant western blot analysis with highlighted bands and sample labels.
- https://cdn.elifesciences.org/articles/94811/elife-94811-fig3-figsupp1-data2-v1.pdf
-
Figure 3—figure supplement 1—source data 3
Original file for the western blot analysis in Figure 3—figure supplement 1B.
- https://cdn.elifesciences.org/articles/94811/elife-94811-fig3-figsupp1-data3-v1.zip
-
Figure 3—figure supplement 1—source data 4
Figure 3—figure supplement 1B and original scans of the relevant Western blot analysis with highlighted bands and sample labels.
- https://cdn.elifesciences.org/articles/94811/elife-94811-fig3-figsupp1-data4-v1.pdf
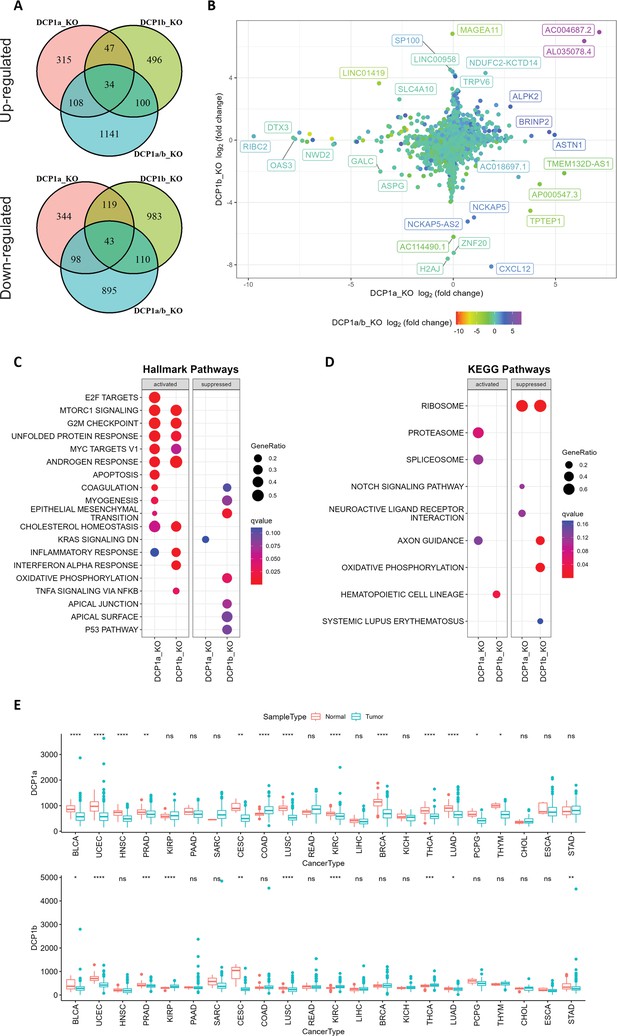
Gene expression analysis and pathway enrichment reveal distinct roles of DCP1a and DCP1b in human cells.
(A) The upper and lower panels display Venn diagrams illustrating the number of genes that were significantly up- and downregulated in DCP1a, DCP1b, and DCP1a/b knockout cells (referred to as DCP1a_KO, DCP1b_KO, and DCP1a/b_KO), respectively. The overlapping regions indicate the number of genes significantly altered in multiple cell lines, highlighting shared and unique gene expression changes across different knockouts. (B) The plot shows the distribution of fold changes in gene expression when comparing DCP1a_KO or DCP1b_KO to wild-type (WT) cells. The points are colored according to the fold changes observed when comparing DCP1a/b_KO to WT, visually representing how the loss of DCP1a and DCP1b individually and combined affects gene expression. (C) Gene Set Enrichment Analysis (GSEA) results for hallmark pathways comparing DCP1a knockout and WT cells (left panel) and DCP1b knockout and WT cells (right panel). Activated and suppressed pathways are shown, with dots colored based on the q-value, indicating the statistical significance of the enrichment. (D) GSEA results for KEGG pathways comparing DCP1a knockout and WT cells (left panel) and DCP1b knockout and WT cells (right panel). Like panel (C), activated and suppressed pathways are shown, with dots colored according to the q-value. This analysis helps identify the biological pathways most affected by the loss of DCP1a or DCP1b. (E) Boxplots of the mRNA expression levels of DCP1a and DCP1b in various cancer types from The Cancer Genome Atlas (TCGA). Statistically significant differences in expression levels are labeled on top of each pair, with significance determined by the Wilcoxon test (ns: not significant; *p≤0.05; **p≤0.01; ***p≤0.001). This panel provides insights into the potential roles of DCP1a and DCP1b in different cancers, highlighting their differential expression patterns.
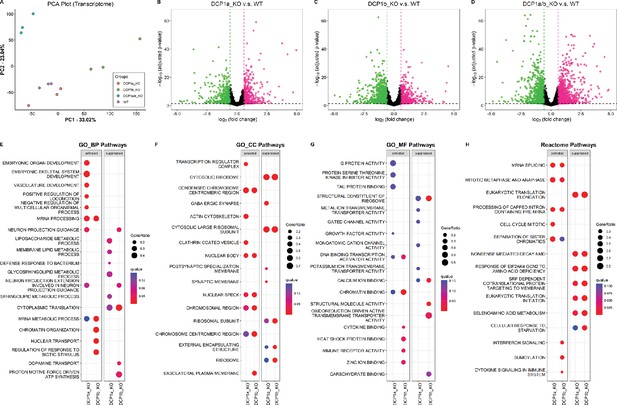
Transcriptome analysis and pathway enrichment in DCP1 knockout HEK-293T cells.
(A) Principal component analysis (PCA) plot showing the transcriptome profiles of 12 samples, including wild-type HEK-293T and DCP1 knockout cell lines (DCP1a_KO, DCP1b_KO, and DCP1a/b_KO). This plot illustrates the distinct clustering of samples based on their transcriptome profiles, highlighting the differences between wild-type and knockout cells. (B–D) Volcano plots comparing transcript levels between (B) DCP1a knockout and wild-type, (C) DCP1b knockout and wild-type, and (D) DCP1a/b double knockout and wild-type. Genes that show a significant increase are marked in pink (fold change >1.5, adjusted p-value<0.05), while genes that show a significant decrease are marked in green (fold change <–1.5, adjusted p-value<0.05). These plots identify the genes significantly upregulated or downregulated in each knockout compared to wild-type cells. (E–H) Gene Set Enrichment Analysis (GSEA) results comparing various pathways and Gene Ontology (GO) terms between DCP1a knockout and wild-type, and DCP1b knockout and wild-type. (E) Biological Process GO terms. The left panel shows activated pathways, while the right panel shows suppressed pathways. Dots are colored based on the q-value, indicating the statistical significance of the enrichment. (F) Cellular Component GO terms, following the same format as panel (E), highlighting the cellular components most affected by the knockouts. (G) Molecular Function GO terms, again with activated pathways on the left and suppressed pathways on the right, provide insights into the molecular functions impacted by the loss of DCP1a or DCP1b. (H) Reactome pathways, showing the enriched pathways in DCP1a knockout and wild-type, and DCP1b knockout and wild-type comparisons. The left and right panels show activated and suppressed pathways, respectively.
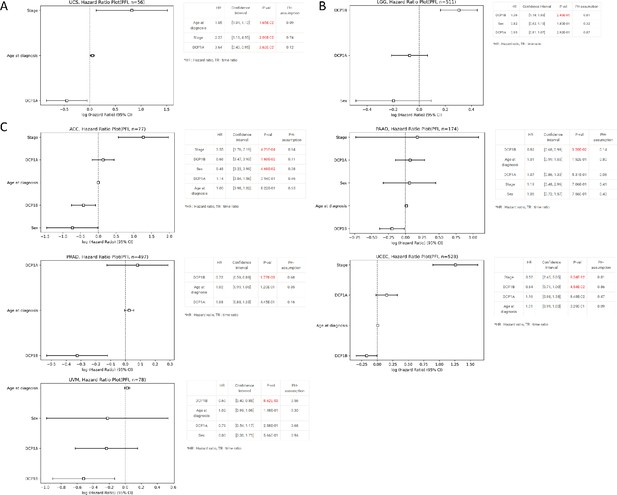
Results of Cox regression analysis for survival analysis of genes DCP1a and DCP1b in progression-free interval (PFI) in cancers.
(A) Age at diagnosis, stage, and expression level of DCP1a are all significantly associated with PFI in Uterine Carcinosarcoma (UCS). The hazard ratio of DCP1a smaller than 1 suggests that a higher expression level of DCP1a is associated with longer PFI. (B) A higher expression level of DCP1b is significantly associated with a shorter PFI in Brain Lower Grade Glioma (LGG), with a hazard ratio significantly larger than 1. (C) A higher expression level of DCP1b is significantly associated with a longer PFI in adrenocortical carcinoma (ACC), pancreatic adenocarcinoma (PAAD), prostate adenocarcinoma (PRAD), uterine corpus endometrial carcinoma (UCEC), and uveal melanoma (UVM).
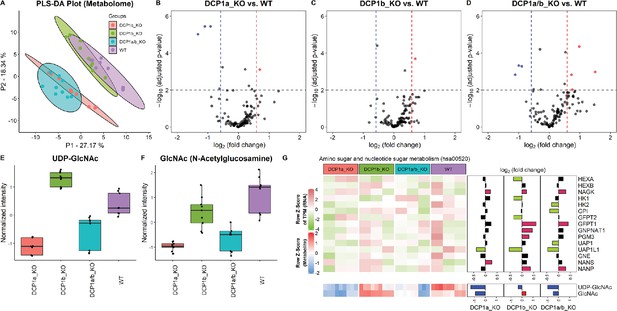
Metabolome profiling unveils unique functions of DCP1 paralogs in human cells.
(A) Partial least squares discriminant analysis (PLS-DA) plot illustrating the metabolome profiles of 12 samples, with three technical replicates for each knockout cell line. This plot shows how the metabolomic profiles of DCP1a_KO, DCP1b_KO, and DCP1a/b_KO cells differ from each other and wild-type (WT) cells, indicating distinct metabolic alterations resulting from the knockouts. (B–D) Volcano plots comparing metabolite levels between (B) DCP1a_KO and WT, (C) DCP1b_KO and WT, and (D) DCP1a/b_KO and WT. Metabolites with an absolute fold change greater than 1.5 and a false discovery rate (FDR)-adjusted p-value less than 0.01 were considered significant. These plots highlight the metabolites significantly upregulated or downregulated in each knockout compared to WT, providing insight into the metabolic impact of DCP1a and DCP1b loss. (E, F) Box plots of (E) UDP-GlcNAc and (F) GlcNAc levels between different cell lines. These plots show the relative abundance of specific metabolites in the different knockout and WT cells, indicating how the disruption of DCP1a and DCP1b affects specific metabolic pathways. (G) Normalized expression levels, abundance of transcripts and metabolites, and fold changes in the amino sugar and nucleotide sugar metabolism pathway. Significantly upregulated and downregulated genes are colored in pink and blue, respectively. Upregulated and downregulated metabolites are colored in red and blue, respectively. This panel integrates transcriptomic and metabolomic data to provide a comprehensive view of how the amino sugar and nucleotide sugar metabolism pathway is altered in DCP1 knockout cells.
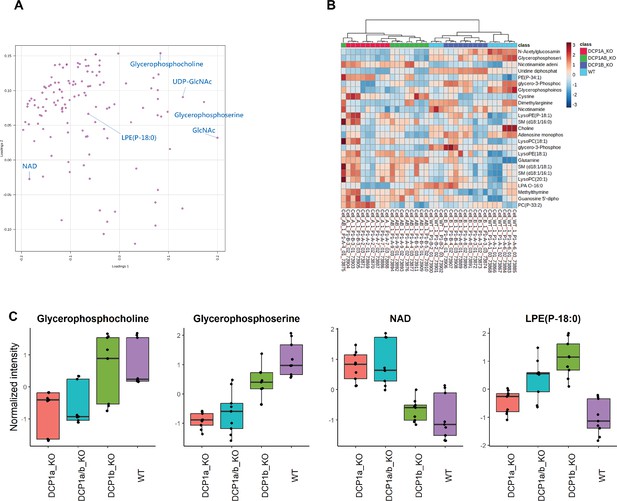
Metabolomic analysis of DCP1 knockout and wild-type HEK-293T cells.
(A) Partial least squares discriminant analysis (PLS-DA) loading plot displaying the top 25 features with the highest Variable Importance in Projection (VIP) scores from DCP1a_KO, DCP1b_KO, DCP1a/b_KO, and wild-type (WT) cell lines. This plot highlights the metabolites that contribute most to the separation between different cell lines, providing insights into the metabolic changes associated with DCP1 knockouts. (B) Heatmap of the top 25 features with the highest VIP scores, showing their relative abundance across DCP1a_KO, DCP1b_KO, DCP1a/b_KO, and WT cell lines. The heatmap visually represents the differences in metabolite levels between the cell lines, with color coding indicating relative abundance. (C–F) Box plots comparing the levels of specific metabolites: (C) glycerophosphocholine levels; (D) glycerophosphoserine levels; (E) NAD levels; (F) LPE(P-18:0) levels between wild-type and DCP1 knockout cell lines. These box plots provide quantitative comparisons of these metabolites, illustrating the impact of DCP1 knockouts on their abundance. Each panel presents the mean values ± standard deviations (SD) for three independent experiments, highlighting significant metabolic alterations in the knockout cells.
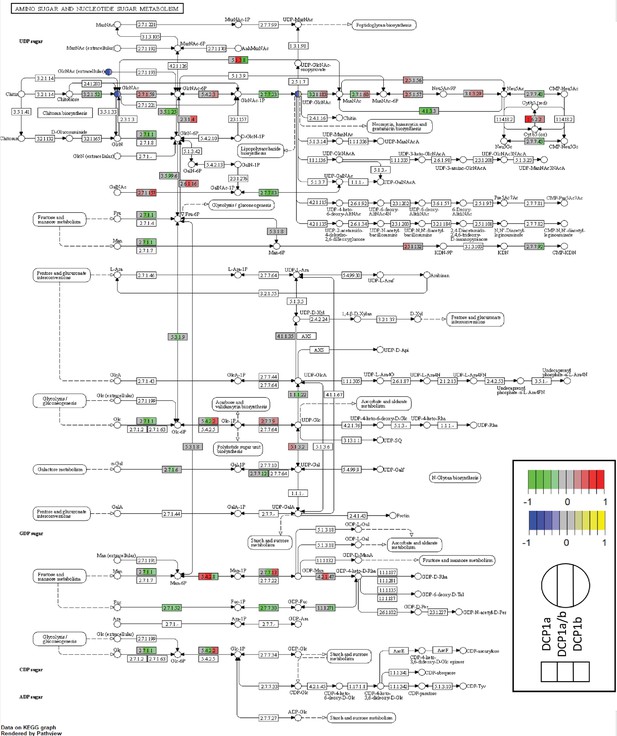
Amino sugar and nucleotide sugar metabolism pathways in DCP1 knockout HEK-293T cells.
Amino sugar and nucleotide sugar metabolism pathways colored based on the fold change of transcript (green and red) or metabolites (blue and yellow) in an order of DCP1a_KO to WT, DCP1a/b_KO to WT and DCP1b_KO to WT. This visualization provides a comprehensive overview of how DCP1 knockouts affect the metabolism of amino sugars and nucleotide sugars at both the transcriptomic and metabolomic levels, highlighting key metabolic shifts in these pathways across different knockout cell lines compared to wild-type cells.
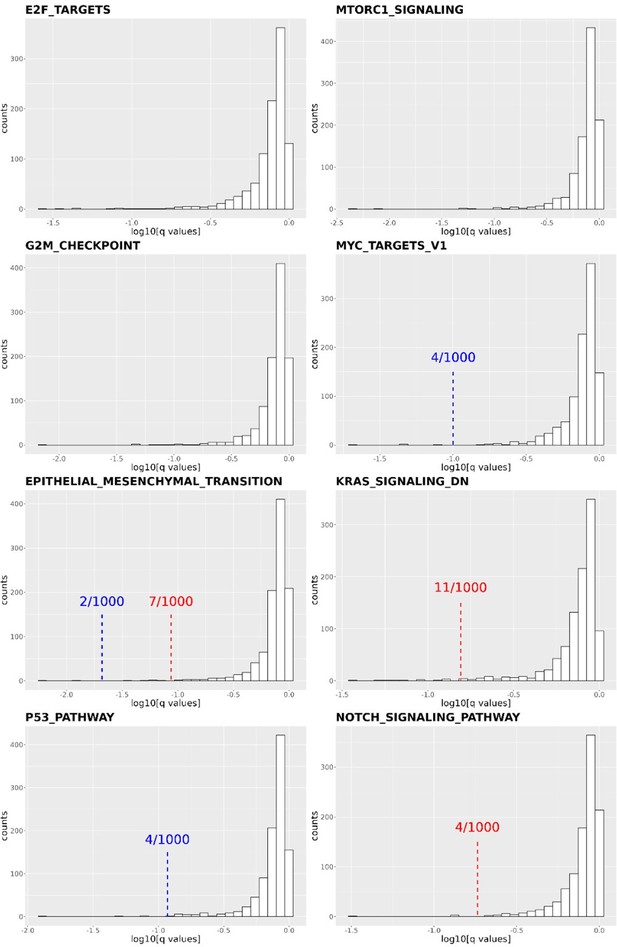
Distribution of q-values resulting from the Gene Set Enrichment Analysis (GSEA) conducted on 1,000 shuffled gene lists for eight cancer-related pathways.
The q-values derived from Figure 4C and 4D are indicated by red (DCP1a_KO) and blue (DCP1b_KO) dashed lines, respectively. Some q-values derived from Figure 4C are too small to be labeled on the plots, such as in E2F targets (q value: 5.87E-07), MTORC1 signaling (q values: 6.59E-07 and 1.58E-06 for DCP1a_KO and DCP1b_KO, respectively), MYC target V1 (q value: 0.004644174 for DCP1a_KO), etc. The numbers x/1000 indicate how often the shuffled q-values were smaller than the real q-value out of 1,000 permutations.
Tables
Reagent type (species) or resource | Designation | Source or reference | Identifiers | Additional information |
---|---|---|---|---|
Gene (Homo sapiens) | DCP1a | GenBank | HGNC:18714 | |
Gene (H. sapiens) | DCP1b | GenBank | HGNC:24451 | |
Strain, strain background (Escherichia coli) | BL21 Star (DE3) | Thermo Fisher | Invitrogen: C601003 | |
Cell line (H. sapiens) | HEK-293T | ATCC | CRL-3216 | |
Cell line (H. sapiens) | HEK-293T DCP1a KO | Elisa Izaurralde Lab | This paper | Cell line obtained from Elisa Izaurralde's laboratory. |
Cell line (H. sapiens) | HEK-293T DCP1b KO | Elisa Izaurralde Lab | This paper | Cell line obtained from Elisa Izaurralde's laboratory. |
Cell line (H. sapiens) | HEK-293T DCP1a/b KO | Elisa Izaurralde Lab | This paper | Cell line obtained from Elisa Izaurralde's laboratory. |
Transfected construct (H. sapiens) | pT7-EGFP-C1-MBP | Elisa Izaurralde Lab | Addgene #146318 | |
Transfected construct (H. sapiens) | pT7-EGFP-C1-HsDCP2 | Elisa Izaurralde Lab | Addgene #25031 | |
Transfected construct (H. sapiens) | pT7-EGFP-C1-HsDCP2 E148Q | Elisa Izaurralde Lab | Addgene #147650 | |
Transfected construct (H. sapiens) | pCIneo-V5-SBP-HsDCP2 | Elisa Izaurralde Lab | This paper | Plasmid obtained from Elisa Izaurralde's laboratory. |
Transfected construct (H. sapiens) | pT7-EGFP-C1-HsDCP1a | Elisa Izaurralde Lab | Addgene #25030 | |
Transfected construct (H. sapiens) | pT7-EGFP-C1-HsDCP1a EVH1 | Elisa Izaurralde Lab | Addgene #147089 | |
Transfected construct (H. sapiens) | pT7-EGFP-C1-HsDCP1a 1–168 | Elisa Izaurralde Lab | This paper | Plasmid obtained from Elisa Izaurralde's laboratory. |
Transfected construct (H. sapiens) | pT7-EGFP-C1-HsDCP1a 1–254 | Elisa Izaurralde Lab | This paper | Plasmid obtained from Elisa Izaurralde's laboratory. |
Transfected construct (H. sapiens) | pT7-EGFP-C1-HsDCP1a 1–538 | Elisa Izaurralde Lab | This paper | Plasmid obtained from Elisa Izaurralde's laboratory. |
Transfected construct (H. sapiens) | pT7-EGFP-C1-HsDCP1a ΔEVH1 | Elisa Izaurralde Lab | Addgene #147090 | |
Transfected construct (H. sapiens) | pT7-EGFP-C1-HsDCP1a ΔHLM | Elisa Izaurralde Lab | This paper | Plasmid obtained from Elisa Izaurralde's laboratory. |
Transfected construct (H. sapiens) | pT7-EGFP-C1-HsDCP1b | Elisa Izaurralde Lab | Addgene #147022 | |
Transfected construct (H. sapiens) | pEF-DEST51 HA-PNRC1 | Giovanni Tonon Lab | Addgene #123294 | |
Transfected construct (H. sapiens) | pCIneo-V5-SBP-MBP-PNRC2 | Elisa Izaurralde Lab | Addgene #147761 | |
Transfected construct (H. sapiens) | pcDNA3.1-β-globin-6xMS2bs | Elisa Izaurralde Lab | Chang et al., 2014 | |
Transfected construct (H. sapiens) | pcDNA3.1-β-globin-GAPDH | Elisa Izaurralde Lab | Chang et al., 2014 | |
Transfected construct (H. sapiens) | pcDNA3.1-MS2-HA | Elisa Izaurralde Lab | Addgene #147544 | |
Transfected construct (H. sapiens) | pcDNA3.1-MS2-HA-HsSMG7 | Elisa Izaurralde Lab | Addgene #147556 | |
Transfected construct (H. sapiens) | pcDNA3.1-MS2-HA-Strep-HsSMG7 | Elisa Izaurralde Lab | This paper | Plasmid obtained from Elisa Izaurralde's laboratory. |
Transfected construct (H. sapiens) | pcDNA3.1-MS2-HA-HsTNRC6A SD | Elisa Izaurralde Lab | Addgene #147556 | |
Transfected construct (H. sapiens) | pcDNA3.1-MS2-HA-HsNanos1 | Elisa Izaurralde Lab | Addgene #147931 | |
Transfected construct (H. sapiens) | pSUPER.puro-PNRC1 | Elisa Izaurralde Lab | This paper | Target sequence: CAAAGTTTAGTGATCCACCTTT |
Transfected construct (H. sapiens) | pSUPER.puro-PNRC2 | Elisa Izaurralde Lab | This paper | Target sequence: AGTTGGAATTCTAGCTTAT |
Antibody | Anti-V5 (mouse monoclonal) | Bio-Rad | Bio-Rad #MCA1360GA | WB (1:1000) |
Antibody | Anti-GFP (rabbit polyclonal) | Elisa Izaurralde Lab | For IP | |
Antibody | Anti-GFP (mouse monoclonal) | Roche | Roche #11814460001 | WB (1:2000) |
Antibody | Anti-HA-HRP (mouse monoclonal) | Roche | Roche #12013819001 | WB (1:5000) |
Antibody | Anti-Tubulin (mouse monoclonal) | Sigma-Aldrich | Sigma-Aldrich #T6199 | WB(1:5000) |
Antibody | Anti-DCP2 (rabbit polyclonal) | Bethyl | A302-597A | WB (1:1000); IF (1:1000) |
Antibody | Anti-DDX6 (rabbit polyclonal) | Bethyl | Bethyl #A300-461Z | WB (1:5000); IF (1:1000) |
Antibody | Anti-EDC4 (mouse monoclonal) | Santa Cruz Biotechnology | sc-8418 | WB (1:1000); IF (1:1000) |
Antibody | Anti-DCP1a (C-terminal region; Epitope: a.a 512–528) (rabbit polyclonal) | Sigma | D5444 | WB (1:1000) |
Antibody | Anti-DCP1a (N-terminal region; Epitope: a.a 1–50) (Rabbit polyclonal) | aviva | ARP39353_T100 | WB (1:1000) |
Antibody | Anti-DCP1b (Epitope: a.a 132–236) (Rabbit polyclonal) | Novus | NBP1-82018 | WB (1:1000) |
Antibody | Anti-EDC3 (mouse monoclonal) | Abcam | Ab57780 | WB (1:1000) |
Antibody | Anti-PatL1 (rabbit polyclonal) | Bethyl | A303-482A-M | WB (1:1000) |
Antibody | Anti-4ET (rabbit polyclonal) | Bethyl | A300-706A | WB (1:1000); IF (1:1000) |
Sequence-based reagent | β-globin_F | This paper | qPCR primers | ATGGTGCACCTGACTCCTGAG |
Sequence-based reagent | β-globin_R | This paper | qPCR primers | TTAGTGATACTTGTGGGCCAGGGC |
Sequence-based reagent | GAPDH_F | Weber and Chang, 2024 | qPCR primers | ctctgctcctcctgttcgacag |
Sequence-based reagent | GAPDH_R | Weber and Chang, 2024 | qPCR primers | ttcccgttctcagccttgacgg |
Software, algorithm | RSEM | Li and Dewey, 2011 | ||
Other | GENCODE hg38 | Frankish et al., 2019 | ||
Software, algorithm | DESeq2 | Love et al., 2014 | ||
Software, algorithm | GSEA | Subramanian et al., 2005 | ||
Software, algorithm | clusterProfiler | Wu et al., 2021a | ||
Software, algorithm | ComplexHeatmap | Gu et al., 2016 | ||
Software, algorithm | DoSurvive | Wu et al., 2023 |
Additional files
-
Supplementary file 1
Differentially expressed genes identified by comparing transcriptomes between DCP1 knockout and wild-type samples.
(a) Differentially expressed genes identified by comparing transcriptomes between DCP1a knockout and wild-type samples. (b) Differentially expressed genes identified by comparing transcriptomes between DCP1b knockout and wild-type samples. (c) Differentially expressed genes identified by comparing transcriptomes between DCP1a/b knockout and wild-type samples.
- https://cdn.elifesciences.org/articles/94811/elife-94811-supp1-v1.xlsx
-
MDAR checklist
- https://cdn.elifesciences.org/articles/94811/elife-94811-mdarchecklist1-v1.pdf