Dysfunction of Calcyphosine-Like gene impairs retinal angiogenesis through the MYC axis and is associated with familial exudative vitreoretinopathy
eLife assessment
This study explores the role of calcyphosine-like (CAPSL) in Familial Exudative Vitreoretinopathy (FEVR) via the MYC pathway, offering valuable insights into disease mechanisms that are supported by a solid, multi-pronged approach. The manuscript, which presents the phenotype of an interesting new mouse model, provides convincing evidence that CAPSL variants cause disease.
https://doi.org/10.7554/eLife.96907.3.sa0Valuable: Findings that have theoretical or practical implications for a subfield
- Landmark
- Fundamental
- Important
- Valuable
- Useful
Convincing: Appropriate and validated methodology in line with current state-of-the-art
- Exceptional
- Compelling
- Convincing
- Solid
- Incomplete
- Inadequate
During the peer-review process the editor and reviewers write an eLife Assessment that summarises the significance of the findings reported in the article (on a scale ranging from landmark to useful) and the strength of the evidence (on a scale ranging from exceptional to inadequate). Learn more about eLife Assessments
Abstract
Familial exudative vitreoretinopathy (FEVR) is a severe genetic disorder characterized by incomplete vascularization of the peripheral retina and associated symptoms that can lead to vision loss. However, the underlying genetic causes of approximately 50% of FEVR cases remain unknown. Here, we report two heterozygous variants in calcyphosine-like gene (CAPSL) that is associated with FEVR. Both variants exhibited compromised CAPSL protein expression. Vascular endothelial cell (EC)-specific inactivation of Capsl resulted in delayed radial/vertical vascular progression, compromised endothelial proliferation/migration, recapitulating the human FEVR phenotypes. CAPSL-depleted human retinal microvascular endothelial cells (HRECs) exhibited impaired tube formation, decreased cell proliferation, disrupted cell polarity establishment, and filopodia/lamellipodia formation, as well as disrupted collective cell migration. Transcriptomic and proteomic profiling revealed that CAPSL abolition inhibited the MYC signaling axis, in which the expression of core MYC targeted genes were profoundly decreased. Furthermore, a combined analysis of CAPSL-depleted HRECs and c-MYC-depleted human umbilical vein endothelial cells uncovered similar transcription patterns. Collectively, this study reports a novel FEVR-associated candidate gene, CAPSL, which provides valuable information for genetic counseling of FEVR. This study also reveals that compromised CAPSL function may cause FEVR through MYC axis, shedding light on the potential involvement of MYC signaling in the pathogenesis of FEVR.
Introduction
A well-organized vascular system is crucial for the proper development of most tissue and organ morphogenesis (Vogenstahl et al., 2022; Ye et al., 2009). Consequently, angiogenic defects have been associated with several congenital human diseases (Carmeliet and Jain, 2011; Fallah et al., 2019). Familial exudative vitreoretinopathy (FEVR) is a heritable vitreoretinopathy characterized by defective retinal angiogenesis and various complications, such as extensive neovascularization, exudation, retinal folds and detachments, and vision loss (Yonekawa et al., 2015). Based on an ocular screening of over 60,000 Chinese newborns, the estimated prevalence of FEVR is around 0.46% (Fei et al., 2021). Due to the significant genetic heterogeneity, FEVR displays all Mendelian forms of inheritance: autosomal dominant (AD), autosomal recessive (AR), or X-linked recessive (XR) (Plager et al., 1992; Gow and Oliver, 1971; de Crecchio et al., 1998). To date, mutations in 17 genes and 1 locus have been identified to cause FEVR, including Norrin (NDP) (Chen et al., 1993), frizzled 4 (FZD4) (Robitaille et al., 2002), low-density lipoprotein receptor-related protein 5 (LRP5) (Gong et al., 2001; Jiao et al., 2004), low-density lipoprotein receptor-related protein 6 (LRP6) (Li et al., 2022), tetraspanin-12 (TSPAN12) (Junge et al., 2009; Nikopoulos et al., 2010), α-catenin (CTNNA1) (Zhu et al., 2021), β-catenin (CTNNB1) (Panagiotou et al., 2017; Liu et al., 2024; He et al., 2023), p120-catenin (CTNND1) (Yang et al., 2022), zinc finger protein 408 (ZNF408) (Collin et al., 2013), kinesin family member 11 (KIF11) (Robitaille et al., 2014), atonal homolog 7 (ATOH7) (Khan et al., 2012), exudative vitreoretinopathy 3 (EVR3) (Downey et al., 2001), integrin-linked kinase (ILK) (Park et al., 2019), jagged canonical Notch ligand 1 (JAG1) (Zhang et al., 2020), discs large MAGUK scaffold protein 1 (DLG1) (Zhang et al., 2021), transforming growth factor beta receptor 2 (TGFBR2) (Asano et al., 2021), sorting nexin 31 (SNX31) (Xu et al., 2023), and ER membrane protein complex subunit 1 (EMC1) (Li et al., 2023). Nevertheless, these mutations can explain only approximately 50% of FEVR cases (Salvo et al., 2015; Kashani et al., 2014).
Calcyphosine-Like (CAPSL) is a protein-coding gene whose function remains elusive. In 2019, Julia Schreml pointed out that CAPSL is a promising candidate gene for multiple symmetric lipomatosis (MSL), a rare adipose tissue disorder of largely unknown etiology (Lindner et al., 2019). Nevertheless, the specific pathogenic mechanism by which CAPSL triggers MSL remains unclear. The molecular mechanisms governing cellular processes and signaling cascades regulated by CAPSL have yet to be elucidated.
MYC gene family is a group of genes most widely investigated and implicated in the formation, maintenance, and progression of several different cancer types, such as breast cancer, lymphoma, and prostatic cancer (Dang, 2016; Beaulieu et al., 2020; Carroll et al., 2018; Sander et al., 2012). MYC is a master regulator in MYC signaling, which participates in cell metabolism, proliferation, differentiation, and migration (de Alboran et al., 2001). The important role of Myc in vascularization and angiogenesis during tumor development has already been reported in mouse models (Baudino et al., 2002; Knies-Bamforth et al., 2004). Vascular endothelial cell (EC)-specific knockout of Myc in mice led to impaired vascular expansion, thinned and poorly branched vascular, and reduced endothelial proliferation (Wilhelm et al., 2016). Conversely, EC-specific Myc overexpression caused sustained vascular outgrowth, increased EC proliferation, and vessel density (Wilhelm et al., 2016). These studies indicate that MYC is a regulating factor in coordinating EC behavior and vessel development.
In the current study, from a large cohort of FEVR patients, we identified a missense mutation and a stop-gain mutation in the CAPSL gene from four FEVR patients by whole-exome sequencing (WES) analysis. Furthermore, EC-specific Capsl-knockout mouse model exhibited FEVR-like retinal vascular defects, emphasizing the indispensable role of Capsl for the retinal vascular architecture. Knockdown of CAPSL led to compromised proliferation of stalk ECs and retarded migration of tip ECs by regulating the MYC signaling axis. Collectively, this study not only identifies CAPSL as a candidate gene for FEVR, but also presents that a compromised MYC signaling axis might contribute to FEVR.
Results
WES analysis revealed two CAPSL variants in FEVR patients
To evaluate the causative variants that account for FEVR, we applied WES on genomic DNA samples from 120 FEVR families without mutations in known FEVR genes. Sanger sequencing was further applied to validate the variants and genotype–phenotype co-segregation analysis. Thus, two novel candidate variants in the CAPSL gene (NM_144647) were identified in patients with FEVR, and the variants were predicted to be pathogenic by Mutation taster, Polyphen-2, and PROVEAN (Supplementary file 1). In family 3036, a heterozygous c.88C>T (p.R30X) variant was identified in an infant (age 0–5 years old) (Figure 1A). His father was a heterozygous carrier and manifested vascular defects in the retina, including peripheral neovascularization area and leakage by fundus fluorescein angiography, whereas the wild-type mother showed normal vision (Figure 1B, D). The affected amino acid is highly conserved among different species (Figure 1C). The proband (II:1) in the 3104 family has been diagnosed with FEVR and identified with a heterozygous variant c.247C>T (p.L83F) (Figure 1A). His father has also been identified as a carrier of this heterozygous variant, manifested with FEVR symptoms, according to the medical records. Nevertheless, clinical examination data are presently unavailable.
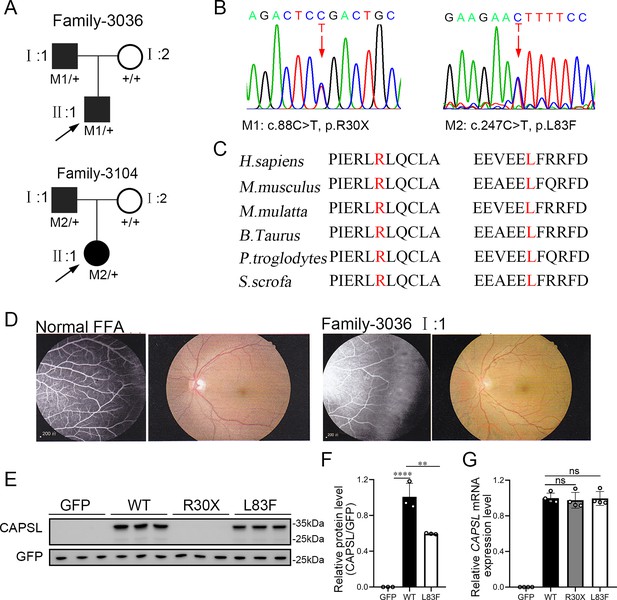
CAPSL point mutations in two families with familial exudative vitreoretinopathy (FEVR).
(A, B) FEVR pedigrees (patients are denoted with black symbols) and Sanger sequencing of two heterozygous mutations identified in two families. Black arrows indicate the proband of each family and red arrows indicate the changed nucleotides. (C) Alignment of amino sequences surrounding the CAPSL variants in different species and all mutated sites are highly conserved. Altered amino acid residues are highlighted in red. (D) Fundus fluorescein angiography (FFA) (left panel) and fundus photography (right panel) of a normal individual and FEVR-affected patient (I:1) in family 3036. Western blot (E, F) and quantitative real time polymerase chain reaction (RT-qPCR) analysis (G) of CAPSL expression of WT and mutant plasmids. An empty vector with GFP tag was used as a negative control. GFP was used as an internal reference. Error bars indicate the standard deviation (SD). **p < 0.01, ****p < 0.0001, ns: no significance, by Student’s t test (n = 3).
-
Figure 1—source data 1
Uncropped and labeled gels for Figure 1.
- https://cdn.elifesciences.org/articles/96907/elife-96907-fig1-data1-v1.zip
-
Figure 1—source data 2
Raw unedited gels for Figure 1.
- https://cdn.elifesciences.org/articles/96907/elife-96907-fig1-data2-v1.zip
To determine the pathogenesis of CAPSL variants for FEVR, we first investigated the effect of variants on the expression of CAPSL. Wild-type and mutant coding sequences of CAPSL were introduced to the GFP-tagged pcDNA3.1 vector, and an IRES2 box was inserted to prevent the fusion expression of CAPSL and GFP. Immunoblot analysis of cells transfected with plasmids revealed that the CAPSL-R30X protein was undetectable, and the L83F variant resulted in a considerably reduced protein level (Figure 1E, F). Conversely, neither variant influenced the mRNA levels of CAPSL (Figure 1G). CAPSL is localized in both the cytoplasm and the nucleus of cells (https://www.proteinatlas.org/), and we then further verified whether these variants have any impact on CAPSL localization. Immunocytochemistry analysis revealed that, consistent with immunoblot analysis, CAPSL-R30X protein was undetectable, and CAPSL-L83F mutant protein exhibited no significant change in localization (Figure 1—figure supplement 1). The TGA stop codon can also have an effect on alternative splicing in certain scenarios, and bioinformatics predictions suggested that the impact of CAPSL 88C>T variant on alternative splicing is minimal (Figure 1—figure supplement 2). Thus, these variants may cause FEVR by reducing CAPSL protein abundance.
Depletion of Capsl in vascular ECs caused FEVR-liked phenotypes
We generated an inducible EC-specific Capsl-knockout mouse model by breeding mice carrying a loxp-flanked allele of Capsl with tamoxifen-inducible Pdgfb-iCreER (Claxton et al., 2008) transgenic animals to determine whether depletion of Capsl in mouse ECs could cause FEVR-like phenotypes (Figure 2—figure supplement 1A). Sanger sequencing analysis confirmed that all experimental mice did not harbor confounding mutations such as rd1 and rd8 mutations (Figure 2—figure supplement 2). Capslloxp/loxp, Pdgfb-CreER mice (hereafter named iECKO), Capslloxp/+, Pdgfb-CreER mice (hereafter named iECKO/+), and their control littermates (Capslloxp/loxp and Pdgfb-CreER, hereafter named Ctrl) were induced by consecutive intraperitoneal injection of 50 μg tamoxifen per pup at postnatal day 1 (P1) to P3 before sacrifice (Figure 2—figure supplement 1B, C). iECKO mice showed indistinguishable overall appearance compared to their Ctrl littermates. The efficiency of EC-specific deletion of Capsl was confirmed by real-time quantitative PCR (RT-qPCR) and western blot analysis of P35 mouse lung tissues. The results showed a pronounced reduction in CAPSL expression, at both mRNA and protein levels, in iECKO mice compared to Ctrl littermates (Figure 2—figure supplement 1D).
FEVR is a genetically heterogeneous eye disease characterized by incomplete vascularization of the peripheral retina in human patients. We initially assessed the vascular development in P5 iECKO/+ mice. The results exhibited no overt differences in vascular progression, vessel density, and vessel branchpoints between iECKO/+ mice and control littermates (Figure 2—figure supplement 3). This feature resembles the vascular development characteristics observed in other heterozygous mice carrying FEVR pathogenic genes, such as Lrp5 (Xia et al., 2008). To assess the role of CAPSL in the growth and patterning of retinal vasculature, we further employed retinal flat-mount analysis to visualize the retinal vessels at different postnatal ages in homozygous mice. As expected, the radial vascular growth, vessel density, and vascular branching were dramatically reduced in P5 iECKO retina relative to Ctrl mice (Figure 2A, D–F). The number of retinal vascular tip cells, which leads EC migration toward high vascular endothelial growth factor (VEGF) by extending filopodia at the angiogenic front (Rattner et al., 2019), was considerably decreased and sparsely distributed in iECKO retinae, compared to that of control littermates (Figure 2B, C, G, H).
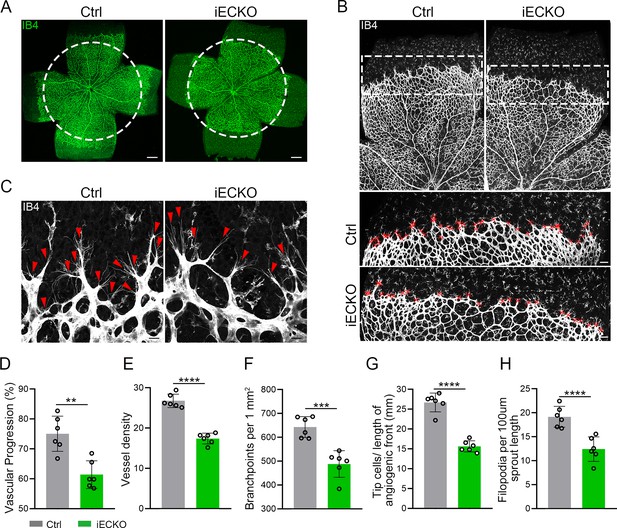
Endothelial cell (EC)-specific inactivation of Capsl impairs retina angiogenesis.
(A) Flat-mounted retinas obtained from P5 Ctrl and littermate iECKO mice were stained with Isolectin-B4 (IB4) to visualize blood vessel. Dashed circle mark the edge of the developing retina vessel in iECKO mice. Scale bar: 250 μm. (B) Low magnification images (top panels) and high magnification images (bottom panels) in boxed areas of IB4-stained angiogenic front of Ctrl and iECKO mice, respectively. Red cross mark tip cells at the angiogenic growth front. Scale bar: 100 μm (top panels), 25 μm (bottom panels). (C) High magnification images of filopodia-extending cells at the edge of retinal angiogenic growth front from Ctrl and iECKO mice. Red arrowheads indicate the sprouts at the angiogenic growth front. Scale bar: 50 μm. (D–H) Quantification of retinal vascular development parameters, including vascular progression, vessel density, branchpoints, number of tip cells, and number of filopodia. Error bars indicate the standard deviation (SD). **p < 0.01, ***p < 0.001, ****p < 0.0001, by Student’s t test (n = 6).
The onset of mouse retinal vascular development occurs postnatally at P0, coinciding with the regression of the hyaloid vasculature (Saint-Geniez and D’amore, 2004; Ito and Yoshioka, 1999). Similar to the previously reported hyaloid phenotypes observed in the FEVR-associated mouse models (Junge et al., 2009; Chen et al., 2012), the hyaloid vasculature in iECKO at P10 displayed delayed regression in comparison to the Ctrl littermates (Figure 3A). Following the expansion of the superficial vessel plexus at around P8, retinal vessels extend perpendicularly into the deep retina, forming secondary and deep third capillary layers (Fruttiger, 2007). To investigate whether the depletion of Capsl hinders deep capillary vascular development, we conducted immunostaining on P10 retinal sections. The results revealed a delayed development of deep retinal vessels in iECKO mouse retinae compared to that of Ctrl (Figure 3B). In addition, immunostaining of P14 flat-mount retina confirmed an incomplete architecture of the deep vascular layer in iECKO mouse (Figure 3C). By P21, EC growth was closely resembling control retinas in iECKO mice when angiogenesis almost complete (Figure 3—figure supplement 1).
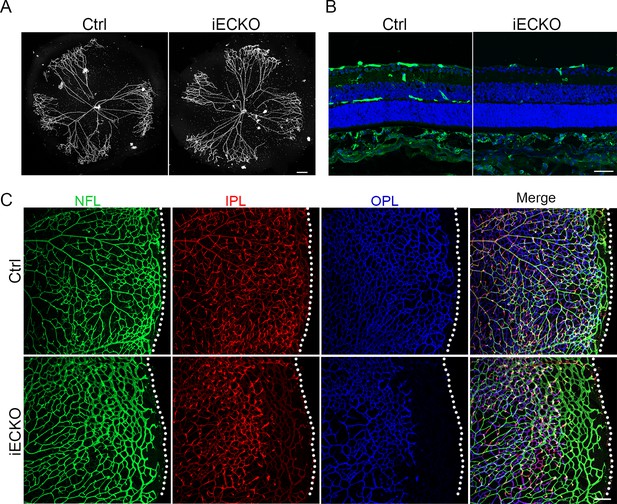
Loss of Capsl results in delayed hyaloid regression and deep retinal blood vessel growth.
(A) Hyaloid vessels stained with DAPI in the eyes of Ctrl and iECKO mice at P10. Scale bar: 250 μm. (B) Retina sections from P10 Ctrl and iECKO mice were co-stained with Isolectin-B4 (IB4) (greed) and DAPI (blue). Scale bar: 100 μm. (C) Flat-mounted retains stained with IB4 at P14 Ctrl and iECKO mice. Optical sections of z-stacked confocal images were divided to represent the nerve fiber layer (NFL), inner plexiform layer (IPL), and outer plexiform layer (OPL). Dashed lines mark the edge of the developing retina. Scale bar: 100 μm.
CAPSL controls vascular proliferation and migration
The development of retinal vasculature is a complex process encompassing not only the coordinated behavior of ECs but also the intricate interactions among different cell types and cytokines within the retina (Selvam et al., 2018). Astrocytes form a mesh as a template prior to vascular growth and guide angiogenic expansion by secreting gradient distributed VEGFA (Dorrell et al., 2002; Gerhardt et al., 2003; Stenzel et al., 2011). Abnormal activation of astrocytes and subsequent inflammatory responses have been observed in multiple FEVR mouse models (Junge et al., 2009; Zhu et al., 2021; Fruttiger, 2007; Luhmann et al., 2005). To explore whether the gradient and expression level of VEGFA were affected by the depletion of Capsl, we conducted immunostaining of VEGFA on the retina. However, the results showed no alteration of VEGFA (Figure 4—figure supplement 1A). Meanwhile, the activation state and the pattern of the vascular template for EC outgrowth formed by astrocytes remained normal, indicated by glial fibrillary acidic protein (GFAP) (Figure 4—figure supplement 1B). Pericyte recruitment is necessary for vessel stability and maturation (Hellström et al., 2001). The immunostaining of Desmin or NG2 staining exhibited no difference between Ctrl and iECKO mice (Figure 4—figure supplement 1C). To assess the integrity of the retinal vascular barrier, we performed the intraperitoneal injection of 5- (and-6)-tetramethylrhodamine biocytin (biocytin-TMR, 869 Da), a small-molecular-weight fluorescent tracerm (Knowland et al., 2014). The results showed no notable leakage in the iECKO retina, suggesting the intact blood barrier despite the depletion of ECs-Capsl (Figure 4—figure supplement 1D). The extracellular matrix (ECM) coordinates the behavior of ECs by regulating cell–cell communication processes (Liu and Senger, 2004). The deficiency and abnormal accumulation of ECM can both disrupt the adhesion, proliferation, and migration of the ECs, leading to defects in blood vessel architecture (Fujiwara et al., 2004; Fischer et al., 2009; Santos-Oliveira et al., 2015). We thus analyzed the ECM accumulation using fibronectin 1, and the result showed normal deposition of ECM (Figure 4—figure supplement 1E). In conclusion, EC-specific knockout of Capsl may mainly lead to defects in retinal vascular ECs rather than other vascular-associated cells.
Considering the compromised vascular progression and decreased vessel density in iECKO mouse retinae, we first performed the EdU assay on retina flat mounts co-stained with ETS transcription factor ERG (endothelial cell nuclear marker) to assess the proliferation of ECs. The results showed a strongly reduced proliferation of Capsl-defective ECs compared to that of Ctrl (Figure 4A). As previously reported, the regressed retinal vessels exhibited sleeve-like positivity for basement matrix component Collagen IV and negativity for Isolectin IB4 (Birdsey et al., 2015). To compare the vascular regression between iECKO and Ctrl retinae, we conducted co-staining of the Collagen IV and IB4. The results revealed that depletion of Capsl leads to an increased vascular regression, evidenced by a significant increase in Collagen IV basal membrane sleeves lacking IB4 both in the capillary plexus and angiogenic front (Figure 4B).
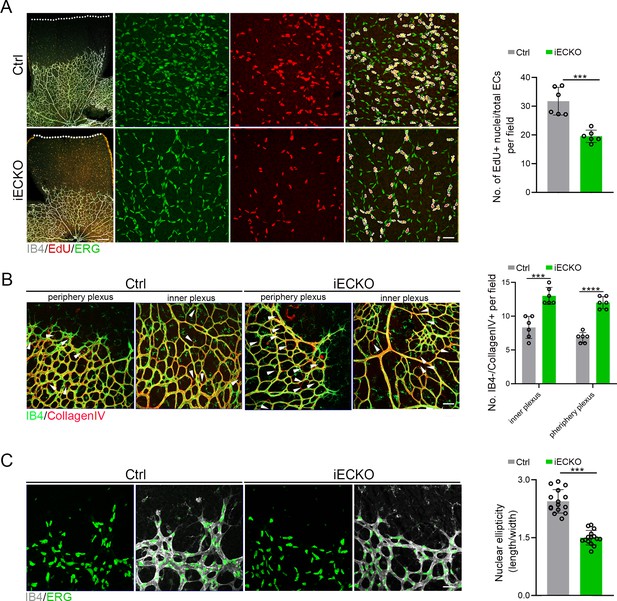
Deletion of Capsl impairs endothelial cell (EC) proliferation and migration.
(A) Retina EC proliferation of Ctrl and iECKO mice at the vitreal surface was measured with EdU and ERG labeling at P5. Images captured at higher magnification are shown at right. Dashed lines mark the edge of the developing retina, and dashed circles represent both EdU+ and ERG+ cells. EC proliferation ability was measured by the ration of EdU+ and ERG+ cells per vascular area. Scale bar: 200 and 50 μm (enlarged insets). Error bars indicate the standard deviation (SD). ***p < 0.001, by Student’s t test (n = 6). (B) Representative images of retinal vessels at the periphery plexus and inner plexus of Ctrl and iECKO mice at P5 co-stained with Isolectin-B4 (IB4) (green) and Collagen IV (red). Arrowhead point to empty Collagen IV sleeves. And quantification of ratio of Collagen IV-positive vessel segments to IB4 labeling-negative vessel segments. Scale bar: 50 μm. Error bars indicate the standard deviation (SD). ***p < 0.001, ****p < 0.0001, by Student’s t test (n = 6). (C) Magnified images of IB4+ vessels and ERG+ nuclei of ECs at angiogenic front of Ctrl and iECKO mice at P5. Quantification of tip cell nuclear ellipticity at the angiogenic growth front. Scale bar: 50 μm. Error bars indicate the standard deviation (SD). ***p < 0.001, by Student’s t test (n = 14).
Additionally, considering the pivotal role of tip cells in the direction of vascular development, we then asked whether depletion of Capsl impairs the polarity of tip cells by analyzing the morphology of cell nuclei at the angiogenic front. This analysis was based on the observation that the nuclei of actively migrating tip cells are elliptical and exhibit increased sphericity (decreased ellipticity) in static tip cells (Coxam et al., 2014; Kim et al., 2019). In Ctrl mice retinae, the nuclei of tip ECs were predominantly elliptical, pointing toward the avascular area (Figure 4C). Conversely, in the retinae of iECKO mice, the nuclei of tip ECs appeared more spherical and did not orient toward the avascular area (Figure 4C). These findings suggest that CAPSL is critical for EC proliferation, vascular regression, and cell polarity.
Endothelial CAPSL is required for cell polarity and filopodia/lamellipodia formation
To investigate the functional mechanism and signaling cascade by which CAPSL regulates EC behaviors, we performed in vitro experiments on primary human retinal microvascular endothelial cells (HRECs). Stable CAPSL knockdown HRECs were generated using the lentivirus-mediated shRNA system. RT-qPCR analysis showed that the mRNA level of CAPSL was significantly decreased (Figure 2—figure supplement 1E). In line with the overall reduced vascular coverage in iECKO mice retina, CAPSL-knockdown HRECs (shCAPSL-ECs) exhibited considerably impaired tube formation in vitro (Figure 5A). EdU labeling also revealed decreased cell proliferation of shCAPSL-ECs compared to that of shCtrl-ECs (Figure 5B). We further conducted flow cytometry to determine whether cell cycle arrest occurred upon depletion of CAPSL in HRECs. The redistribution of cell cycle phases indicated that the cell cycle was arrested during the G0/G1 phase in the shCAPSL-ECs (Figure 5C, D). To further investigate the role of CAPSL in EC migration, we performed the scratch-wound assay in confluent shCAPSL-ECs and shCtrl-ECs, as previously reported (Kim et al., 2019; Carvalho et al., 2019). Nine hours after scratching, ECs were co-stained with GM130 and phalloidin to visualize the Golgi apparatus and the cytoskeleton. The results indicated that the nuclei of shCAPSL-ECs were more spherical than those of shCtrl-ECs (Figure 5E, F). Given that the axial polarity can reflect the direction and migration of ECs (Franco et al., 2015; Kwon et al., 2016), we further measured the cell polarity (nucleus-to-Golgi polarity axis) of ECs at the wound edge (Kim et al., 2019; Carvalho et al., 2019). As a result, the Golgi apparatus of shCtrl-ECs was predominantly positioned around the wound edge, while that of shCAPSL-ECs showed more random positioning (Potente et al., 2011; Figure 5H).
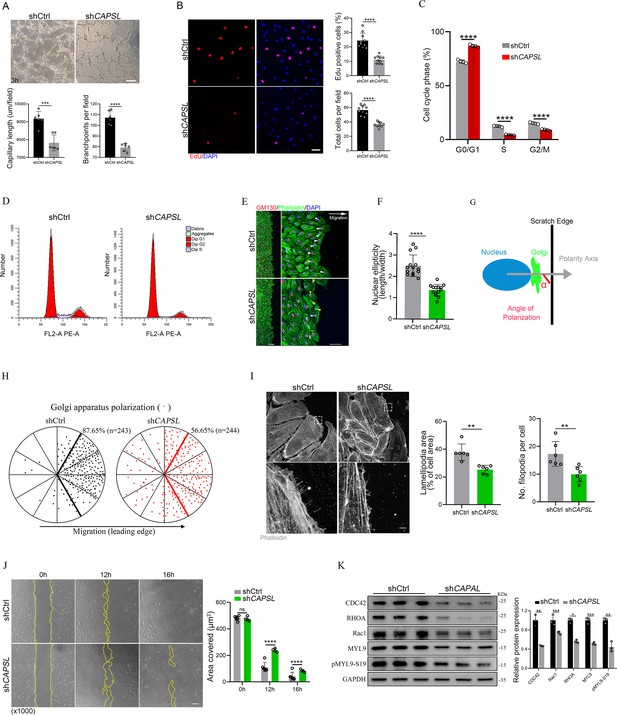
Depletion of CAPSL in human retinal microvascular endothelial cells (HRECs) compromises in vitro endothelial cell (EC) proliferation and migration.
(A) Representative images of in vitro tube formation after transfection of HRECs with shRNA. Scale bar: 200 μm. Error bars indicate the standard deviation (SD). ***p < 0.001, ****p < 0.0001, by Student’s t test (n = 6). (B) Incorporation of EdU in shRNA transfected HRECs. Representative confocal images and quantification of proliferating HRECs both in number per field and proportion of EdU-positive cells. Scale bar: 50 μm. Error bars indicate the standard deviation (SD). ****p < 0.0001, by Student’s t test (n = 10). (C, D) Cell cycle analysis of shCtrl-ECs and shCAPSL-ECs by flow cytometry. Error bars indicate the standard deviation (SD). ****p < 0.0001, by Student’s t test (n = 4). (E) Representative images of phalloidin actin cytoskeleton (green) and GM130 (red) showing polarity angles of shCtrl-ECs and shCAPSL-ECs at the edge of scratch wound. The arrow points toward the wound. Colored arrowheads represent different migration state. Scale bar: 200 μm (left panel), 50 μm (right panel). (F) Quantification of nuclear ellipticity of HRECs at the margin of wound scratch. Error bars indicate the standard deviation (SD). ****p < 0.0001, by Student’s t test (n = 14). (G) Schematic pictures showing the define of polarity axis of each cell. Polarity axis was measured with the angle (α) between the scratch edge and the vector drawn from the center of nucleus to the center of the Golgi apparatus. (H) Polar plots showing Golgi apparatus polarization. The bold lines represent 120° region centered on the vector, which is perpendicular to the wound scratch. The dots represent the angle (α) of each cell and the numbers indicate the frequency of dots within the 120° region of the bold line of shCtrl-ECs (n = 243) and shCAPSL-ECs (n = 244). (I) Images of phalloidin-stained actin cytoskeleton and comparisons of indicated parameters in shCtrl-ECs and shCAPSL-ECs at the edge of scratch wound. The dashed boxed region is shown at higher magnification at the bottom panel. Scale bar: 50 μm (top panels), 25 μm (bottom panels). Error bars indicate the standard deviation (SD). **p < 0.01, by Student’s t test (n = 6). (J) Representative images of wound scratch assay at 0, 12, and 16 hr after wound was made. And the quantification of covered area at different time point. The dashed line indicates the gap of the wound after wound scratch at different time point. Scale bar: 200 μm. Error bars indicate the standard deviation (SD). ****p < 0.0001, ns:no significance, by Student’s t test (n = 6). (K) Immunoblot and quantification analysis of expression of small GTPase proteins and a key regulator of contractile force MYL9 in shCtrl-ECs and shCAPSL-ECs. Error bars indicate the standard deviation (SD). *p < 0.05, **p < 0.01, ***p < 0.001, by Student’s t test (n = 3).
-
Figure 5—source data 1
Uncropped and labeled gels for Figure 5.
- https://cdn.elifesciences.org/articles/96907/elife-96907-fig5-data1-v1.zip
-
Figure 5—source data 2
Raw unedited gels for Figure 5.
- https://cdn.elifesciences.org/articles/96907/elife-96907-fig5-data2-v1.zip
At the angiogenic front, the dynamic behaviors of tip cells largely rely on the rearrangement and organization of the actin cytoskeleton (De Smet et al., 2009). Tip cells extend actin-driven filopodia and lamellipodia to probe chemotactic guidance cues, providing direction and migration for the developing vascular network (Kurz et al., 1996; Marin‐Padilla, 1985; Ruhrberg et al., 2002). Notably, shCAPSL-ECs exhibited fewer filopodia, smaller lamellipodia, and significantly impaired wound closure compared to shCtrl-ECs at the wound scratch edge (Figure 5I). Also, as expected, shCAPSL-ECs exhibited significantly impaired wound closure at the same time point after scratching relative to shCtrl-ECs (Figure 5J). Small Rho GTPases, including CDC42 and Rac, are essential for lamellipodial extension and cell migration (Fraccaroli et al., 2015; Hall, 2005). Western blot analysis uncovered markedly attenuated expression of CDC42, RHOA, and Rac1 (Figure 5K). Moreover, both MYL9 and phosphorylated MYL9 at Ser19, which are crucial regulators of contractile force, were also drastically reduced in shCAPSL-ECs compared to those of shCtrl-ECs (Figure 5K). These results indicated that CAPSL regulates EC polarity, filipodia/lamellipodia formation, and migration by modulating the actomyosin cytoskeleton.
CAPSL regulates EC proliferation through the MYC signaling axis
Given the fact that FEVR is predominantly characterized by the compromised Norrin/β-catenin signaling pathway as its main causative factor (Chen et al., 1993; Junge et al., 2009; Zhu et al., 2021; Panagiotou et al., 2017), we first asked whether loss of CAPSL function causes FEVR through Norrin/β-catenin signaling. TopFlash reporter gene system was used to determine the activity of the Norrin/β-catenin signaling pathway. Knocking down CAPSL expression in human embryonic kidney 293 (HEK293) SuperTopFlash (STF) cells led to similar luciferase activity, compared to cells transfected with shCtrl (Figure 6—figure supplement 1A). Furthermore, transfection of shRNA-resistant CAPSL plasmid (WT, R30X, or L83F) in CAPSL-knockdown 293STF cells resulted in no significant difference in luciferase activity (Figure 6—figure supplement 1B). These results indicated that CAPSL was not a major player in Norrin/β-catenin signaling to regulate retinal vascular development.
We next performed unbiased transcriptomic and proteomic analyses on the control and CAPSL-knockdown HRECs to explore the underlying mechanism by which CAPSL regulates EC proliferation and migration. RNA sequencing (RNA-seq) identified 1961 up-regulated genes and 2205 down-regulated genes in shCAPSL-ECs compared to shCtrl-ECs (logFC >0.58, p-value <0.05), 1342 up-regulated genes and 1218 down-regulated genes in ECs overexpressing CAPSL (LentiOE-ECs) compared to Ctrl-ECs (Figure 6A). Gene set enrichment analysis (GSEA) on transcriptomic data showed multiple down-regulated signaling axes upon the depletion of CAPSL, including E2F targets (NES = −3.2944438, p-value = 0), G2/M checkpoints (NES = −3.2851038, p-value = 0), MYC targets (NES = −2.8795638, p-value = 0), and mitotic spindle (NES = −2.646523, p-value = 0) (Figure 6B, C). In line with this, overexpression of CAPSL in HRECs also caused a significant increase in E2F targets (NES = 2.7765026, p-value = 0), G2/M checkpoints (NES = 2.6528199, p-value = 0), and MYC targets (NES = 2.6528199, p-value = 0) (Figure 6D, E). Meanwhile, proteomic profiling showed similar expression patterns with that of transcriptomic profiling upon knockdown or overexpression of CAPSL (Figure 6F–I). Furthermore, we applied correlation analysis on the transcriptomic and proteomic data, which divided the genes into nine quadrants (Figure 6J). GSEA analysis was performed on genes in the third and seventh quadrants, which were regulated at the transcriptional level (Figure 6K and Supplementary file 2). Interestingly, the down-regulated signaling pathways are highly consistent with those observed in GSEA analysis of both transcriptomic and proteomic analyses (Figure 6K). Given that the MYC was an established driver of cell growth and migration (Lourenco et al., 2021), and that the genes in the MYC signaling were down-regulated upon CAPSL depletion (Figure 6L), we speculated that CAPSL might play a pivotal role in MYC signaling in HRECs. RT-qPCR analysis was further performed to validate the downregulation fo MYC target genes in the lung tissue of Ctrl and iECKO mice (Figure 6—figure supplement 2).
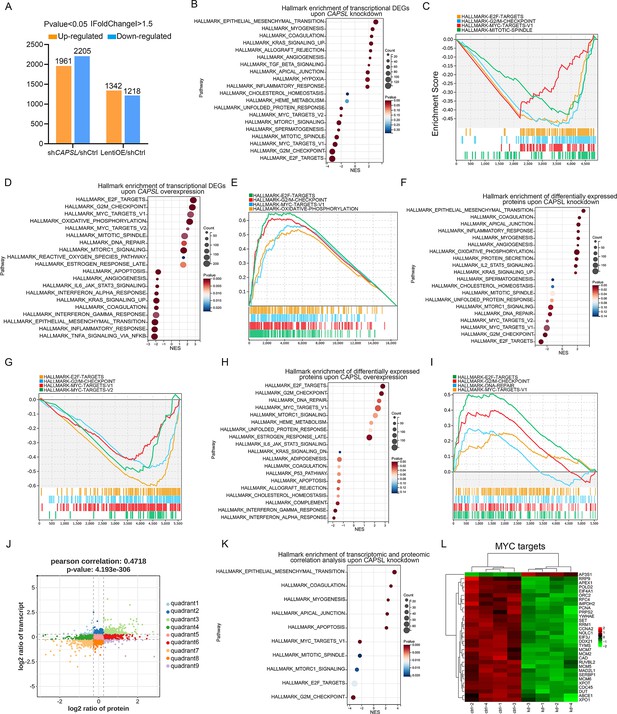
CAPSL suppresses MYC signaling axis.
(A) Differential gene expression information of shCAPSL-ECs versus shCtrl-ECs group and LentiOE-ECs versus shCtrl-ECs group. (B–E) Gene set enrichment analysis (GSEA) on the RNA sequencing data of human retinal microvascular endothelial cells (HRECs). Top 10 ranked up- or down-regulated signaling axis was listed (B) and top 4 down-regulated gene sets were listed (C) in comparison of shCtrl-ECs versus shCAPSL-ECs. Top 10 ranked up- or down-regulated signaling axis was listed (D) and top 4 up-regulated gene sets were listed (E) in comparison of LentiOE-ECs versus shCtrl-ECs. (F–I) GSEA on the proteomic profiling data of HRECs. Top 10 ranked up- or down-regulated signaling axis was listed (F) and top 4 down-regulated gene sets were listed (G) in comparison of shCtrl-ECs versus shCAPSL-ECs. Top 10 ranked up- or down-regulated signaling axis was listed (H) and top 4 up-regulated gene sets were listed (I) in comparison of LentiOE-ECs versus shCtrl-ECs. (J) Correlated RNAs and proteins enriched in nine quadrants of shCtrl-ECs versus shCAPSL-ECs. (K) GSEA on the genes/proteins in quadrants 3 and 7, and top 5 ranked up- or down-regulated signaling axis was listed. (L) Clustered heatmap of the expression fold changes of several MYC signature genes of in both RNA profiling and proteomic profiling of shCtrl-ECs and shCAPSL-ECs.
We next incorporated an analysis of transcriptome profiling of human umbilical vein endothelial cells (HUVECs) infected with lentiviruses encoding control- or MYC-targeting genomic RNAs (gRNAs; GSE161815) (Andrade et al., 2021). Intriguingly, GSEA analysis revealed that ablation of cMYC in HUVECs led to compromised E2F targets, MYC targets, and G2/M checkpoint signaling activity (Figure 7A, B), largely consistent with those observed in the absence of CAPSL. This also suggests that cMYC might play a role in the upstream regulation of E2F targets and G2/M checkpoint signaling. Venn diagram analysis of down-regulated genes (LogFC <−0.585, p < 0.05) in CAPSL-depleted HRECs and MYC-depleted HUVECs revealed a large proportion of shared genes, indicative of similar transcriptional regulatory patterns between CAPSL and MYC (Figure 7C and Supplementary file 3). Additionally, these genes are predominently enriched in the MYC targets signaling (Figure 7D–F).
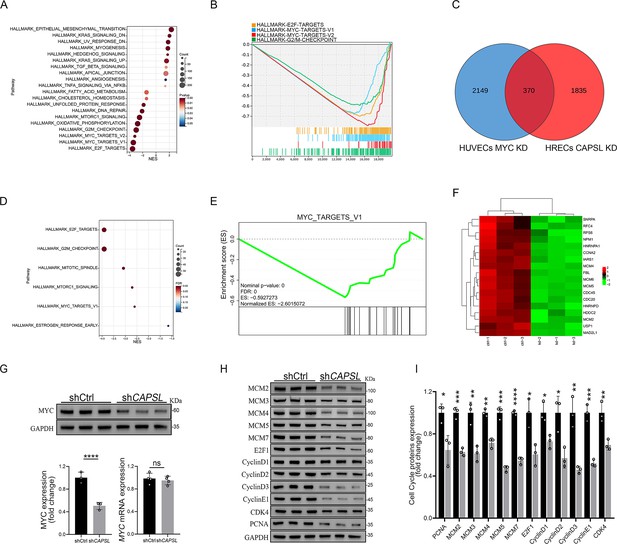
Loss of CAPSL led to similar transcriptional regulatory patterns to the loss of MYC in human umbilical vein endothelial cells (HUVECs).
(A, B) Gene set enrichment analysis (GSEA) on the RNA sequencing data in comparison of shCtrl-HUVECs versus shMYC-HUVECs. Top 10 ranked up- or down-regulated signaling axis was listed (A) and top 4 down-regulated gene sets were listed (B). (C) Venn diagram analysis of down-regulated genes in CAPSL-depleted human retinal microvascular endothelial cells (HRECs) and cMYC-depleted HUVECs. (D, E) GSEA on the shared down-regulated genes in CAPSL-depleted HRECs and MYC-depleted HUVECs. (F) Heatmap of MYC signature genes of shared genes based on Venn analysis. (G) MYC expression level in shCtrl-ECs and shCAPSL-ECs was quantified by western blot and RT-qPCR. Error bars indicate the standard deviation (SD). ****p < 0.0001, ns: no significance by Student’s t test (n = 3). (H) Western blot analysis of expression of MYC targets. (I) Quantification analysis of MYC targets. Error bars indicate the standard deviation (SD). *p < 0.05, **p < 0.01, ***p < 0.001, ****p < 0.0001, by Student’s t test (n = 3).
-
Figure 7—source data 1
Uncropped and labeled gels for Figure 7.
- https://cdn.elifesciences.org/articles/96907/elife-96907-fig7-data1-v1.zip
-
Figure 7—source data 2
Raw unedited gels for Figure 7.
- https://cdn.elifesciences.org/articles/96907/elife-96907-fig7-data2-v1.zip
We, therefore, asked whether CAPSL regulates MYC expression. Notably, the depletion of CAPSL significantly decreased the protein level of MYC, rather than the mRNA level in HRECs (Figure 7G). Subsequently, western blot analysis revealed that expression levels of MYC target genes, such as MCM family, E2F1, CyclinD family, and PCNA, were decreased in the absence of CAPSL in HRECs (Figure 7H, I). Taken together, these findings suggest MYC as a potential functional regulator downstream of CAPSL.
Discussion
Over the last decades, major progress has been made in understanding the molecular mechanisms underlying FEVR, and 17 FEVR-causing genes have been identified (Chen et al., 1993; Robitaille et al., 2002; Gong et al., 2001; Jiao et al., 2004; Li et al., 2022; Junge et al., 2009; Nikopoulos et al., 2010; Zhu et al., 2021; Panagiotou et al., 2017; Liu et al., 2024; He et al., 2023; Yang et al., 2022; Collin et al., 2013; Robitaille et al., 2014; Khan et al., 2012; Downey et al., 2001; Park et al., 2019; Zhang et al., 2020; Zhang et al., 2021; Asano et al., 2021; Xu et al., 2023; Li et al., 2023). However, the pathogenic genes and mechanisms underlying approximately 50% of clinical cases remain elusive (He et al., 2023; Yang et al., 2022). In this study, we report a new FEVR candidate gene CAPSL and uncover the pivotal roles of CAPSL in retina vascular development. The EC-specific Capsl-knockout mouse model exhibited FEVR phenotypes, including delayed vascular progression, retarded hyaloid vessel regression, and decreased vessel density. Deficiency of CAPSL in ECs resulted in compromised cell proliferation and defective EC migration, providing insights into the regulatory roles of CAPSL in retina vascularization (Figures 2—5).
During retinal development, angiogenesis comprises various processes such as EC specification, adhesion, proliferation, migration, and pruning (Fruttiger, 2007). The regulation of angiogenesis entails the control of EC behaviors and the interactions between ECs, ECM, and other types of retinal cells (Franco et al., 2015). In this study, we observed impaired radial expansion and vertical invasion, as well as the increased pruning of the retinal vascular ECs upon inactivating endothelial Capsl (Figures 2 and 3). However, neither the expression level nor the gradience of VEGFA was disturbed in iECKO mice, which could be attributed to the intact blood barrier in the iECKO retina (Figure 4—figure supplement 1). In addition, the loss of Capsl resulted in the presence of more spherical tip cell nuclei, which were directed more randomly to the avascular area (Figure 4). These results provide evidence that CAPSL acts as a novel regulator for collective EC behavior.
In vitro functional studies demonstrated that depletion of CAPSL impaired tube formation, EC proliferation ability, and EC polarity (Figure 5). Moreover, the formation of filopodia and lamellipodia is also disturbed by the depletion of CAPSL (Figure 5). Using the unbiased transcriptomic and proteomic sequencing, bioinformatic analysis, and western blot assay, we demonstrated that the defects in CAPSL affect EC function by down-regulating the MYC signaling cascade (Figures 6 and 7). MYC has been shown to be a critical mediator of anabolic metabolism, cell growth, and migration (Dang, 2013; Adhikary and Eilers, 2005). Aberrant upregulation of the MYC signaling pathway is frequently observed in many types of cancers (Dang, 2012; Stine et al., 2015). Interestingly, the phenotypes of retinal vessels in iECKO mice resemble the vascular defects in MyciEC-KO mice, exhibiting FEVR-like phenotypes, such as impaired vascular progression, decreased branch points, and reduced EC proliferation (de Alboran et al., 2001; Wilhelm et al., 2016).
The Norrin/β-catenin signaling pathway is a subset of Wnt signaling pathway that is specifically activated during vascular development of the central nervous system (He et al., 2023; Martowicz et al., 2019). Although several signaling pathways have been implicated in the pathogenesis of FEVR, variants in crucial components of Norrin/β-catenin signaling account for most of the FEVR cases (Junge et al., 2009; Zhu et al., 2021; Panagiotou et al., 2017). It is worth mentioning that MYC is widely recognized as a downstream target of Wnt signaling, which is transcriptionally regulated during Wnt activation (Green et al., 2024; Rennoll and Yochum, 2015; Cairo et al., 2012). However, to our knowledge, there is a lack of literature reporting on the correlation between the MYC signaling pathway and FEVR. Interestingly, here we proved that the absence of CAPSL leads to a decrease in MYC protein level, which down-regulates MYC signaling without affecting Norrin/β-catenin signaling (Figure 7 and Figure 6—figure supplement 1). Consequently, we propose MYC as a potential therapeutic target for FEVR, a hypothesis that warrants substantiation through subsequent investigations.
Additionally, the formation of filopodia and lamellipodia, which is reported to be intimately related to the actomyosin dynamics and the activity of small Rho GTPases such as CDC42 (Barry et al., 2015; Zihni et al., 2016), is significantly reduced in CAPSL-defective HRECs. In our study, we confirmed that the knockdown of CAPSL led to a significant reduction of CDC42, which might be the causative mechanism behind the disturbed formation of filopodia and lamellipodia (Figure 5).
Taken together, our study demonstrated that CAPSL is potentially a novel candidate gene associated with FEVR. We also demonstrated that variants in the CAPSL gene, independently of canonical Norrin/β-catenin signaling, cause FEVR through inactivating MYC signaling, expanding FEVR-involved signaling pathways.
Materials and methods
DNA sequencing of patients and controls
Request a detailed protocolThe study was approved by the Institutional Research Committees of Sichuan Provincial People’s Hospital (approval number: NSFC2014-009). Informed consent was obtained from all participants and from guardians of minors involved in genetic testing. The patients were clinically diagnosed with exudative vitreoretinopathy. Genomic DNA was extracted from the peripheral blood of FEVR families and control individuals using a blood DNA extraction kit (QIAGEN, Germantown, Maryland, USA) according to standard protocol. WES was performed on DNA samples to identify candidate pathogenic genes for FEVR as previously described (Zhu et al., 2021). Family ID numbers 3036 and 3104 are not known to anyone outside the research group.
Mouse model and genotyping
Request a detailed protocolCapslloxp/+ (named Capslem1Zxj) model was generated using the CRISPR/Cas9 nickase technique by Viewsolid Biotechology (Beijing, China) in C57BL/6J background. The gRNA sequence was as follows: Capsl-L gRNA: 5′-CTATCCCAA TTGTGCTCCTGG-3′; Capsl-R gRNA: 5′-TGGGACTCATGGTTCTAGAGG-3′. Pdgfb-iCreER (Claxton et al., 2008) transgenic mice on a mixed background of C57BL/6 and CBA was obtainted from Dr. Marcus Fruttiger and backcrossed to background for 6 generations. Capslloxp/+ mice were bred with Pdgfb-iCreER (Claxton et al., 2008) transgenic mice to generate Capslloxp/loxp, Pdgfb-iCreER mice. Genomic DNA samples extracted from mouse tails were genotyped using PCR to detect loxp-flanked Capsl and Pdgfb-iCreER. Primer sets used for Capsl loxp were: 5′-GGCAGGTAAGATGGTGTC-3′ and 5′-TCTGTTTGTGGATCAATGTG-3′; and primer sets used for Pdgfb-iCreER were: 5′-GCCGCCGGGATCACTCTCG-3′ and 5′-CCAGCCGCCGTCGCAACTC-3′. All mice were maintained as breeding colonies with standard rodent water and diet in a 12:12-hr dark cycle under specific pathogen-free conditions, and both males and female mice were used for experimental analysis. Experiments involving animals were conducted in accordance with institutional guidelines and following the protocols approved by the Institutional Animal Care and Use Committee of Sichuan Provincial People’s Hospital (approval number: NSFC2014-009).
Preparation of flat-mount retinae and retina sections
Request a detailed protocolEnucleated eyes were fixed for 15 min in 4% paraformaldehyde (PFA) at room temperature, followed by immersion in phosphate buffered saline (PBS) for the same duration. The retinae were then dissected and partially cut into four quadrants as previously described (Liu et al., 2022). The dissected retinal cups were post-fixed overnight and then stained as described below. As for retina sections, after fixation in 4% PFA for 2 hr, the enucleated eyes were dehydrated until they settled to the bottom of the tube with 30% sucrose and then embedded in Tissue Freezing Medium (Servicebio). Specimens were sectioned, cleaned, and stained as previously described (Yang et al., 2019).
Hyaloid vessel preparation
Request a detailed protocolNeonatal eyes were fixed in 4% PFA for 2 hr before the cornea, lens, and iris were removed. The eye cup was immersed in 5% gelatin at 4°C overnight. The solidified gelatin was extricated and heat melted on a glass slide, washed with warm water, air-dried, and imaged with DAPI staining.
Immunohistochemistry
Request a detailed protocolAll immunostainings of retinae were performed using littermates with similar body size and treated under the same conditions. The flat-mount retina and retina sections were permeabilized and blocked in PBS containing 5% fetal bovine serum and 0.2% Triton X-100 at room temperature for 2 hr. Next, they were rinsed in PBS and incubated in directly conjugated IB4 Alexa 488 or Alexa 594 (1:200; Thermo Fisher, USA) and primary antibodies in a blocking buffer at 4°C overnight. After three washes, the retinae were processed for multiple labeling or flat mounted onto microscope glass slides with Fluoromount (Sigma-Aldrich, USA).
For in vivo analysis of cell proliferation, each pup was injected intraperitoneally with 200 µg EdU 3 hr before the mice were euthanasia. Retinae were dissected and permeabilized as previously described. EDU-positive labeling was stained and detected by means of a Click-iT EDU Alexa Fluor-647 Imaging Kit (C10640, Thermo Fisher Scientific, USA) according to the manufacturer’s instruction. For paracellular BRB integrity analysis, each pup was injected intraperitoneally with 2% fluorescent tracer, 5-(and-6)-tetramethylrhodamine biocytin, biocytin-TMR (CAT#T12921, Thermo Fisher Scientific, USA) for 24 hr prior to sacrifice, followed by flat-mount preparation, blocking, and staining of Isolectin B4.
Images of stained flat-mounted retinae, retina sections, and HRECs were acquired using Zeiss LSM 800 confocal microscope (Thornwood, NY, USA) and processed with Zeiss processing software, Angio Tool, and Adobe Photoshop. The detailed information about antibodies used for immunofluorescence is listed in Supplementary file 4.
Cell lines and primary cell culture
Request a detailed protocolHRECs were purchased from Cell System and cultured in EBM-2 media (CC-3156, Lonza, Switzerland) containing 5% fetal bovine serum, 0.4% hydrocortisone, 4% Hfgf-b, 0.1% vascular endothelial growth factor (VEGF), 0.1% R3-IGF, 0.1% ascorbic acid, 0.1% human epidermal growth factor (hEGF) 0.1% GA-1000, and 0.1% heparin at 37°C in a 5% CO2 incubator. HRECs at passages 3–7 were used for experiments.
HEK-293T and HEK-293STF were obtained from the American Type Culture Collection (ATCC) and cultured in Dulbecco's Modified Eagle Medium (DMEM) (SH30023.01, Hycolon, USA) with 10% fetal bovine serum and cells were maintained at 37°C in a 5% CO2 incubator. Both cell lines were authenticated by STR profiling, and no mycoplasma contamination was found.
Gene knockdown and overexpression strategies
Request a detailed protocolHRECs were seeded on 6 cm dishes at the day before transfected with lentivirus carrying shRNA for human CAPSL (5′-AAGACCTTCGTGAAGTATA-3′, Genechem, Shanghai, China) or negative control shRNA (5′-TTCTCCGAACGTGTCACGT-3′) according to the protocol of the manufacture. Cells were incubated for at least 72 hr before used for further experiments.
For overexpression of exogenous protein in HEK-293T cells, cells were transfected with Lipofectamine-3000 (Invitrogen, USA) according to the manufacturer’s instructions.
Matrigel EC tube formation assay
Request a detailed protocolHRECs transfected with the corresponding shRNA after 72 hr were counted and planted onto the matrigel as previously described (Zhang et al., 2019). After 6 hr of incubation at 37°C in a 5% CO2 incubator, the images were captured under an anatomical lens (Carl Zeiss, Germany).
Immunofluorescence of HREC
Request a detailed protocolAfter cell counting, the same number of HRECs transfected with shCtrl and shCAPSL was seeded on slides in 24-well plates. Cells were fixed with 4% PFA at room temperature for 15 min. After being rinsed in PBS three times, cells were blocked in the blocking buffer at room temperature for 2 hr. The cells were labeled with primary antibodies overnight at 4°C. Following three washes in PBS, Alexa-Fluor-594- or Alexa-Fluor-647-conjugated antibodies were administered along with DAPI, and cells were incubated at room temperature for 1 hr. After three washes in PBS, stained cells were mounted on microscope glass slides with Fluoromount (Sigma-Aldrich, USA).
For in vitro analysis of cell proliferation, 20 μM EdU (Thermo Fisher Scientific, USA) was added into 96-well plated 3 hr before cells were harvested. EdU-positive cells were stained and detected with the Click-iT EDU Alexa Fluor-647 Imaging Kit (C10640, Thermo Fisher Scientific, USA) according to the manufacturer’s instruction.
Wound healing assay
Request a detailed protocolSeventy-two hours after shRNA transfection, HRECs were seeded in 6-well plates with complete medium for 24 hr until grown to confluence. Plates were then coated with 10 μg/ml fibronectin for half an hour in 37°C before cell culture. 200 μl pipette tips were used to generate wounds, and each well was washed twice with Utrasalin A (Lonza, Switzerland) to remove detached cells. Cells were then starved in the EBM-2 medium at 37°C in a 5% CO2 incubator. Images of the wounds were captured with an optical microscope at 0, 12, and 16 hr after the wounds were made, respectively. The wound closure areas were measured using ImageJ software.
For analyses of Golgi apparatus polarization, cells were maintained on 24-well glass slides and fixed with 4% PFA for 15 min at 9 hr after cell migration was initiated.
RNA extraction and RT-qPCR
Request a detailed protocolTotal RNA was extracted from cultured HRECs or fresh lungs from mouse using TRIzol reagent (Life Technologies, USA) according to the manufacturer’s instructions. RNA concentration and quality were measured with NanoDrop 2000 spectrophotometer (Thermo Fisher Scientific, USA), and 1 μg total RNA was reverse transcribed using EasyScript One-Step RT-PCR SuperMix (TransGen Biotech, Beijing, China) following the standard protocol. RT-qPCR was performed using a 7500 Real-Time PCR System (Applied Biosystems, USA) with TransStart Tip Green qPCR Supermix (TransGen Biotech). At least three experiments were performed, and PCR reactions were performed in triplicate. Glyceraldehyde-3-Phosphate Dehydrogenase (GAPDH) was used as a reference gene. The primer sets are listed in Supplementary file 5. All experiments were replicated three times and representative results were shown.
Protein extraction and western blotting
Request a detailed protocolHRECs or lung tissue from mice were lysed in standard radioimmunoprecipitation assay buffer (PIPA, 50 mM Tris–HCl, 150 mM NaCl, 1% Triton X-100, 0.5% sodium deoxycholate, 0.1% sodium dodecyl sulfate, pH 7.4) supplemented with Protease and Phosphatase Inhibitor Cocktail (Roche, USA). After sonication, centrifugation, and protein quantification, the supernatant was diluted in 2× sodium dodecyl sulfate (SDS) loading buffer. Equal amounts of protein (20 μg) were loaded and resolved on 10–15% SDS–polyacrylamide, and western blotting was performed. After gels were transfected onto a polyvinylidene difluoride membrane (GE Healthcare, USA), the membranes were incubated in a blocking buffer containing 8% skimmed milk (9999, Cell Signaling Technology) in tris buffered saline with Tween 20 (TBST) for 1 hr at room temperature on a rocking platform, followed by overnight incubation at 4°C with the primary antibodies diluted in blocking buffer. Membranes were then washed in TBST three times and incubated in the blocking buffer containing secondary Horseradish Peroxidase-conjugated antibodies for 1 hr at room temperature. Protein blots were visualized with e-BLOT Touch Imager (e-BLOT, China) and blots were relatively quantified with ImageJ. GAPDH was used as an internal reference. Detailed information about antibodies used for western blot is listed in Supplementary file 4. All experiments were replicated three times and representative blot images were shown.
Luciferase assays
Request a detailed protocolDual-luciferase assays were performed as previously described (Zhu et al., 2021). Briefly, 293STF cells were plated on 24-well plates at a confluency of ~30%. The following day, the cells were transfected with corresponding plasmids with lip3000. Forty-eight hours post-transfection, cells were harvested, and the lysates were used to measure Firefly and Renilla luciferase activity according to the manufacturer’s instructions (TransGen, Bejing, China).
Quantification of retinal parameters
Request a detailed protocolAll quantifications were done using Zeiss processing software on high-resolution confocal images. Vascular progression was measured in a straight line from the optic nerve to the angiogenic front of the retinal plexus for each retina quadrant (n = 6, each group). Vessel density and branch points were calculated with Angio Tool software from flat-mounted retinae (n = 6, each group). Endothelial tip cell numbers were measured by counting endothelial sprouts at the angiogenic front of the entire vascular plexus of the same length (n = 6, each group). Endothelial tip cell filopodia density was calculated with high-resolution images (×60 objective) of six randomly selected angiogenic front fields from six retinae per genotype (n = 6, each group). EC proliferation was calculated by measuring the number of both EDU- and ERG-positive cells within retinae of the same size and same position at the edge of the vascular plexus (n = 6, each group). Retinal pericyte coverage was quantified by comparing the NG2+ or Desmin+ pericytes with IB4+ vessels (n = 6, each group). The nuclear ellipticity of the cells at the scratch edge was calculated by the distance of the nuclear long axis (Height) divided by the maximum vertical distance to the nuclear long axis (Width).
Transcriptomic profiling
Request a detailed protocolTotal RNA was extracted from shCtrl-ECs, shCAPSL-ECs, and LentiOE-ECs and quantified using NanoDrop2000 Spectrophotometer (Thermo Fisher Scientific, USA). RNA integrity was determined by Bioanalyzer RNA 6000 Nano assay kit (Agilent, China). RNA library construction was performed with Illumina TruseqTM RNA sample prep Kit and RNA-seq was performed with Truseq SBS Kit (300 cycles) in BIOZERON (BIOZERON, China). RNA-seq reads were performed using the TopHat software tool to acquire the alignment file, which was used to quantify mRNA expression and determine the differentially expressed genes. All gene expression values were changed to log2 values for further analysis, and a p-value of less than or equal to 0.05 was considered to indicate significance. The GSEA was performed with the omicshare platform (https://www.omicshare.com). The raw data have been uploaded to the Genome Sequence Archive (https://ngdc.cncb.ac.cn/gsa/browse/HRA010305), and the assigned accession number was HRA010305.
Proteomic profiling
Request a detailed protocolTotal protein was extracted from shCtrl-ECs, shCAPSL-ECs, and LentiOE-ECs and quantified using a BCA protein quantification kit (Beyotime, China). Samples were sent to PTM Biolab (PTM Biolab, China) to perform proteomic profiling using 4D-Labelfree. All protein expression values were changed to log2 values for further analysis, with a corrected p-value <0.05. The GSEA was performed with the omicshare platform (https://www.omicshare.com). The raw data have been uploaded to the Genome Sequence Archive (https://www.ebi.ac.uk/pride/archive), and the assigned accession number was PXD051696.
Image acquisition and statistical analysis
Request a detailed protocolImmunofluorescence images were obtained using a laser scanning microscope (LSM 900, Zeiss). Statistical analyses were performed with GraphPad Prism 8.0 software. Data were analyzed using the Student’s t test for comparison between two groups or one-way analysis of variance followed by Dunnett multiple comparison test for comparison of multiple groups, and plotted as mean ± SD. At least three independent experiments were performed. ImageJ was used to compare results for the tube formation assay experiments and bands of immunoblot. Results were considered significant when *p<0.05, **p<0.01, ***p<0.001, or ****p<0.0001. ‘ns’ stands for ‘no significance’.
Study approval
Request a detailed protocolThis study was approved by the institutional review board of Sichuan Provincial People’s Hospital (approval number: NSFC2014-009) and Xinhua Hospital Affiliated to Shanghai Jiao Tong University School of Medicine, and informed consent was obtained from all participants or legal guardians for minors. All animal studies were performed according to established ethical guidelines approved by the animal care committee of Sichuan Provincial People’s Hospital (approval number: NSFC2014-009).
Data availability
All data produced or analyzed in the present study are included in the manuscript and supplementary files. RNAseq data and Proteomic profiling data of shCtrl-ECs, shCAPSL-ECs, and LentiOE-ECs have been uploaded to the Genome Sequence Archive (https://ngdc.cncb.ac.cn/gsa-human/browse/HRA007150) and EMBL-EBI Archive (https://www.ebi.ac.uk/pride/archive/PXD051696).
-
Genome Sequence ArchiveID HRA007150. RNA-seq raw data for CAPSL HRECs.
-
PRIDEID PXD051696. Proteomic raw data for ctrl HRECs, CAPSL knockdown HRECs,and CAPSL overexpression HRECs.
References
-
Transcriptional regulation and transformation by Myc proteinsNature Reviews. Molecular Cell Biology 6:635–645.https://doi.org/10.1038/nrm1703
-
Control of endothelial quiescence by FOXO-regulated metabolitesNature Cell Biology 23:413–423.https://doi.org/10.1038/s41556-021-00637-6
-
Activation of Wnt and Myc signaling in hepatoblastomaFrontiers in Bioscience 4:480–486.https://doi.org/10.2741/393
-
Principles and mechanisms of vessel normalization for cancer and other angiogenic diseasesNature Reviews. Drug Discovery 10:417–427.https://doi.org/10.1038/nrd3455
-
MYC, metabolism, cell growth, and tumorigenesisCold Spring Harbor Perspectives in Medicine 3:a014217.https://doi.org/10.1101/cshperspect.a014217
-
ConferenceA time for MYC: metabolism and therapyCold Spring Harbor Symposia on Quantitative Biology. pp. 79–83.https://doi.org/10.1101/sqb.2016.81.031153
-
Mechanisms of vessel branching: filopodia on endothelial tip cells lead the wayArteriosclerosis, Thrombosis, and Vascular Biology 29:639–649.https://doi.org/10.1161/ATVBAHA.109.185165
-
Retinal vascular development is mediated by endothelial filopodia, a preexisting astrocytic template and specific R-cadherin adhesionInvestigative Ophthalmology & Visual Science 43:3500–3510.
-
A new locus for autosomal dominant familial exudative vitreoretinopathy maps to chromosome 11p12-13American Journal of Human Genetics 68:778–781.https://doi.org/10.1086/318790
-
Therapeutic targeting of angiogenesis molecular pathways in angiogenesis-dependent diseasesBiomedicine & Pharmacotherapy = Biomedecine & Pharmacotherapie 110:775–785.https://doi.org/10.1016/j.biopha.2018.12.022
-
Development of the retinal vasculatureAngiogenesis 10:77–88.https://doi.org/10.1007/s10456-007-9065-1
-
Rac regulates integrin-mediated endothelial cell adhesion and migration on laminin-8Experimental Cell Research 292:67–77.https://doi.org/10.1016/j.yexcr.2003.08.010
-
VEGF guides angiogenic sprouting utilizing endothelial tip cell filopodiaThe Journal of Cell Biology 161:1163–1177.https://doi.org/10.1083/jcb.200302047
-
Familial exudative vitreoretinopathy: an expanded viewArchives of Ophthalmology 86:150–155.https://doi.org/10.1001/archopht.1971.01000010152007
-
WNT‐activated, MYC ‐amplified medulloblastoma displaying intratumoural heterogeneityNeuropathology and Applied Neurobiology 50:e12945.https://doi.org/10.1111/nan.12945
-
Rho GTPases and the control of cell behaviourBiochemical Society Transactions 33:891–895.https://doi.org/10.1042/BST20050891
-
Novel truncating variants in CTNNB1 cause familial exudative vitreoretinopathyJournal of Medical Genetics 60:174–182.https://doi.org/10.1136/jmedgenet-2021-108259
-
Lack of pericytes leads to endothelial hyperplasia and abnormal vascular morphogenesisThe Journal of Cell Biology 153:543–553.https://doi.org/10.1083/jcb.153.3.543
-
Regression of the hyaloid vessels and pupillary membrane of the mouseAnatomy and Embryology 200:403–411.https://doi.org/10.1007/s004290050289
-
Autosomal recessive familial exudative vitreoretinopathy is associated with mutations in LRP5American Journal of Human Genetics 75:878–884.https://doi.org/10.1086/425080
-
In vivo modulation of endothelial polarization by Apelin receptor signallingNature Communications 7:11805.https://doi.org/10.1038/ncomms11805
-
Variants in the Wnt co-receptor LRP6 are associated with familial exudative vitreoretinopathyJournal of Genetics and Genomics = Yi Chuan Xue Bao 49:590–594.https://doi.org/10.1016/j.jgg.2021.11.010
-
LMBR1L regulates the proliferation and migration of endothelial cells through Norrin/β-catenin signalingJournal of Cell Science 135:jcs259468.https://doi.org/10.1242/jcs.259468
-
Frameshift variants in the C-terminal of CTNNB1 cause familial exudative vitreoretinopathy by AXIN1-mediated ubiquitin-proteasome degradation condensationInternational Journal of Biological Macromolecules 258:128570.https://doi.org/10.1016/j.ijbiomac.2023.128570
-
MYC protein interactors in gene transcription and cancerNature Reviews. Cancer 21:579–591.https://doi.org/10.1038/s41568-021-00367-9
-
Role of the Norrie disease pseudoglioma gene in sprouting angiogenesis during development of the retinal vasculatureInvestigative Ophthalmology & Visual Science 46:3372–3382.https://doi.org/10.1167/iovs.05-0174
-
Early vascularization of the embryonic cerebral cortex: Golgi and electron microscopic studiesJournal of Comparative Neurology 241:237–249.https://doi.org/10.1002/cne.902410210
-
Endothelial β-catenin signaling supports postnatal brain and retinal angiogenesis by promoting sprouting, tip cell formation, and VEGFR (Vascular Endothelial Growth Factor Receptor) 2 ExpressionArteriosclerosis, Thrombosis, and Vascular Biology 39:2273–2288.https://doi.org/10.1161/ATVBAHA.119.312749
-
Next-generation sequencing of a 40 Mb linkage interval reveals TSPAN12 mutations in patients with familial exudative vitreoretinopathyAmerican Journal of Human Genetics 86:240–247.https://doi.org/10.1016/j.ajhg.2009.12.016
-
Defects in the cell signaling mediator β-catenin cause the retinal vascular condition FEVRAmerican Journal of Human Genetics 100:960–968.https://doi.org/10.1016/j.ajhg.2017.05.001
-
X-linked recessive familial exudative vitreoretinopathyAmerican Journal of Ophthalmology 114:145–148.https://doi.org/10.1016/S0002-9394(14)73977-7
-
Roles of HIFs and VEGF in angiogenesis in the retina and brainThe Journal of Clinical Investigation 129:3807–3820.https://doi.org/10.1172/JCI126655
-
Regulation of MYC gene expression by aberrant Wnt/β-catenin signaling in colorectal cancerWorld Journal of Biological Chemistry 6:290–300.https://doi.org/10.4331/wjbc.v6.i4.290
-
Development and pathology of the hyaloid, choroidal and retinal vasculatureThe International Journal of Developmental Biology 48:1045–1058.https://doi.org/10.1387/ijdb.041895ms
-
Next-generation sequencing and novel variant determination in a cohort of 92 familial exudative vitreoretinopathy patientsInvestigative Ophthalmology & Visual Science 56:1937–1946.https://doi.org/10.1167/iovs.14-16065
-
The force at the tip--modelling tension and proliferation in sprouting angiogenesisPLOS Computational Biology 11:e1004436.https://doi.org/10.1371/journal.pcbi.1004436
-
Retinal vasculature development in health and diseaseProgress in Retinal and Eye Research 63:1–19.https://doi.org/10.1016/j.preteyeres.2017.11.001
-
MYC, Metabolism, and CancerCancer Discovery 5:1024–1039.https://doi.org/10.1158/2159-8290.CD-15-0507
-
Vascular regulation of developmental neurogenesisFrontiers in Cell and Developmental Biology 10:890852.https://doi.org/10.3389/fcell.2022.890852
-
A model for familial exudative vitreoretinopathy caused by LPR5 mutationsHuman Molecular Genetics 17:1605–1612.https://doi.org/10.1093/hmg/ddn047
-
Tmem30a deficiency leads to retinal rod bipolar cell degenerationJournal of Neurochemistry 148:400–412.https://doi.org/10.1111/jnc.14643
-
TMEM30A deficiency in endothelial cells impairs cell proliferation and angiogenesisJournal of Cell Science 132:jcs225052.https://doi.org/10.1242/jcs.225052
-
Whole-exome sequencing identified DLG1 as a candidate gene for familial exudative vitreoretinopathyGenetic Testing and Molecular Biomarkers 25:309–316.https://doi.org/10.1089/gtmb.2021.0013
-
Catenin α 1 mutations cause familial exudative vitreoretinopathy by overactivating Norrin/β-catenin signalingThe Journal of Clinical Investigation 131:e139869.https://doi.org/10.1172/JCI139869
-
Tight junctions: from simple barriers to multifunctional molecular gatesNature Reviews. Molecular Cell Biology 17:564–580.https://doi.org/10.1038/nrm.2016.80
Article and author information
Author details
Funding
National Natural Science Foundation of China (82371083)
- Xianjun Zhu
National Natural Science Foundation of China (82121003)
- Zhenglin Yang
Sichuan Province Science and Technology Support Program (2023ZYD0172)
- Xianjun Zhu
Sichuan Provincial People’s Hospital Postdoctoral fund (2022BH019)
- Wenjing Liu
Sichuan Provincial People’s Hospital Huanhua Talent program
- Xianjun Zhu
National Natural Science Foundation of China (82101153)
- Mu Yang
CAMS Innovation Fund for Medical Sciences
- Zhenglin Yang
Sichuan Intellectual Property Office (China)
- Xianjun Zhu
The funders had no role in study design, data collection, and interpretation, or the decision to submit the work for publication.
Ethics
The study was approved by the Institutional Research Committees of Sichuan Provincial People's Hospital (approval number: NSFC2014-009). Informed consent was obtained from all participants and from guardians of minors involved in genetic testing. The patients were clinically diagnosed with exudative vitreoretinopathy. Family ID numbers 3036 and 3104 are not known to anyone outside the research group.
All experiments involving animals were conducted in accordance with institutional guidelines and following the protocols approved by the Institutional Animal Care and Use Committee of Sichuan Provincial People's Hospital (approval number: NSFC2014-009).
Version history
- Sent for peer review:
- Preprint posted:
- Reviewed Preprint version 1:
- Reviewed Preprint version 2:
- Version of Record published:
Cite all versions
You can cite all versions using the DOI https://doi.org/10.7554/eLife.96907. This DOI represents all versions, and will always resolve to the latest one.
Copyright
© 2024, Liu, Li, Yang et al.
This article is distributed under the terms of the Creative Commons Attribution License, which permits unrestricted use and redistribution provided that the original author and source are credited.
Metrics
-
- 902
- views
-
- 77
- downloads
-
- 1
- citation
Views, downloads and citations are aggregated across all versions of this paper published by eLife.
Citations by DOI
-
- 1
- citation for Version of Record https://doi.org/10.7554/eLife.96907.3