Re-examining how complexin inhibits neurotransmitter release
Figures
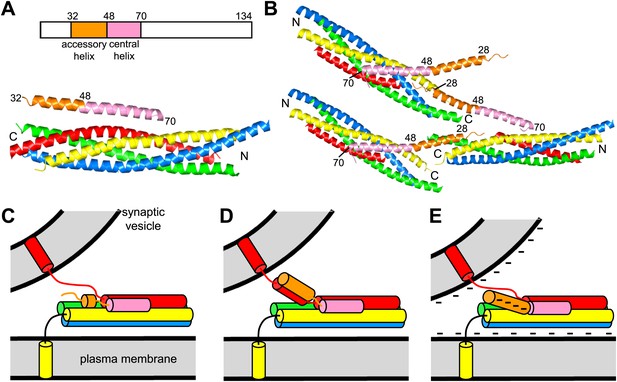
Models of complexin function.
(A) Domain diagram of CpxI and ribbon diagram of the crystal structure of the CpxI(26-83)/SNARE complex (PDB code 1KIL) (Chen et al., 2002). Selected residue numbers are indicated above the ribbon diagram and on the CpxI(26–83) ribbon in the structure. Synaptobrevin is colored in red, syntaxin-1 in yellow, SNAP-25 in blue and green (N-terminal and C-terminal SNARE motifs, respectively), and CpxI(26-83) in orange (accessory helix) and pink (central helix). The same color code is used in all panels. N and C indicate the N- and C-termini of the SNARE motifs. (B) Ribbon diagram of the crystal structure of the complex between the CpxI(26-83) superclamp mutant and a synaptobrevin truncated SNARE complex (PDB code 3RK3) (Kummel et al., 2011). Three complexes are shown to illustrate the zigzag array present in the crystals. (C–E) Models for the inhibitory activity of the complexin accessory helix. In all models, the accessory helix is proposed to prevent C-terminal assembly of a partially assembled SNARE complex either by inserting into the complex (C), by binding to the synaptobrevin SNARE motif (D), or by electrostatic repulsion with both membranes (E).
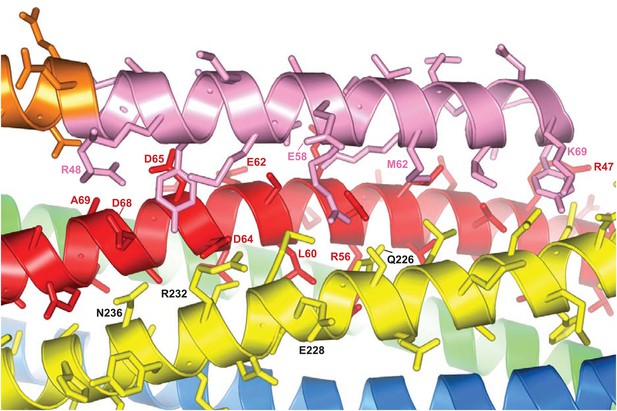
The interface between CpxI and the SNARE complex.
A ribbon diagram of the crystal structure of the CpxI(26-83)/SNARE complex(PDB code 1KIL) (Chen et al., 2002) is shown, with synaptobrevin colored in red, syntaxin-1 in yellow, SNAP-25 in blue and green (N-terminal and C-terminal SNARE motifs, respectively), and CpxI(26-83) in orange (accessory helix) and pink (central helix). The side chains of CpxI(26-83), synaptobrevin and syntaxin-1 are shown, and selected residues are labeled as a guide to see which residues interact and where were the truncations of the different proteins made.
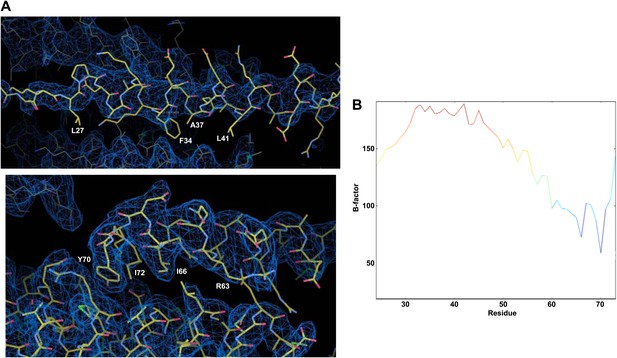
High B-factors in the accessory helix in the crystal structure of the CpxI(26-83) superclamp mutant bound to SCΔ60.
(A) Electron density images of the crystal structure of CpxI(26-83) superclamp mutant bound to SCΔ60 (PDB accession code 3RK3; Kummel et al., 2011). Superimposed on the coordinates from 3RK3 is the map with coefficients 2|mF(obs)−DF(calc)|, contoured at the r.m.s.d. level of the map. The phases and map were calculated from the deposited 3RK3 structure factors in the program PHENIX (Afonine et al., 2010). Note that little electron density is observed for the side chains of the accessory helix, even for the hydrophobic residues in the interface with the SNAREs (the native L41 and the mutant L27, F34 and A37; upper panel). In contrast, clear electron density is observed for the side chains of the central helix that contact the SNARE complex (lower panel; some of these residues are labeled). (B) Plot of average atomic displacement parameters (ADP or ‘B-factors’) over the residues of the CpxI(26-83) superclamp molecule in 3RK3. The plot is color coded from blue as the minimum to red as the maximum value of the average B-factors. Note that the B-factors are much higher for the accessory helix than for the residues of the central CpxI helix that contact the truncated SNARE complex (residues 59 to 72).
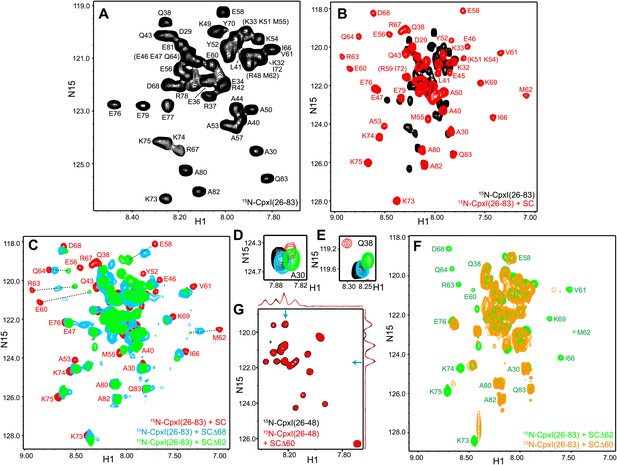
NMR analysis of interactions between 2H,15N-labeled CpxI fragments and synaptobrevin-truncated SNARE complexes.
(A–C) Expansions of 1H-15N TROSY-HSQC spectra of 2H,15N-CpxI(26-83) free (black contours), or bound to non-truncated SNARE complex (red contours), to SCΔ68 (blue contours) or SCΔ62 (green contours). Cross-peaks assignments for the free form are based on those described for full-length CpxI (Pabst et al., 2000) and assignments for CpxI(26-83) bound to non-truncated SNARE complex were described previously (Chen et al., 2002). In (B and C), the minimal contour levels of the different spectra were adjusted to enable visualization of the weakest cross-peaks of interest; hence, cross-peak intensities are not directly comparable. (D and E) Expansions of the regions containing the cross-peaks of A30 (D) or Q38 (E) of the spectra shown in panels (A–C). The minimal contour levels of the different spectra were adjusted to make the cross-peak intensities directly comparable. (F) Expansions of 1H-15N TROSY-HSQC spectra of 2H,15NCpxI(26-83) bound to SCΔ62 (green contours) or SCΔ60 (orange contours). (G) Expansions of 1H-15N TROSY-HSQC spectra of WT 2H,15N-CpxI(26-48) in the absence (black contours) or presence (red contours) of SCΔ60. Because the red and black spectra are practically identical, the black spectrum was plotted at slightly lower levels to facilitate observation of the black crosspeaks behind the red ones. However, the intensities of all the cross-peaks were the same in the black and red spectra within experimental error, as illustrated by the one-dimensional traces shown above and on the right of the two-dimensional contour plots (taken at the chemical shifts indicated by the blue arrows).
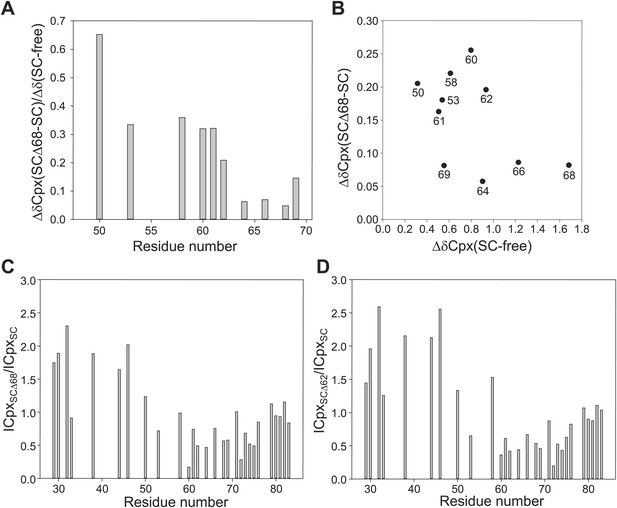
Additional analysis of the interaction between 2H,15N-labeled CpxI fragments and synaptobrevin-truncated SNARE complexes.
(A) Chemical shift changes in the CpxI central helix of SNARE complex-bound 2H,15N-CpxI(26- 83) caused by truncation of synaptobrevin to residue 68, normalized by the changes caused by binding of 2H,15N-CpxI(26-83) to the SC. The chemical shift changes were calculated as Δδ = [(ΔδHN)2 + (0.17*ΔδN)2]1/2, where ΔδHN and ΔδN are the differences in HN and N chemical shifts, respectively, between the spectra being compared. For ΔδCpx(SCΔ68-SC), we compared 1H-15N TROSY-HSQC spectra of 2H,15N-CpxI(26-83) bound to SC and bound to SCΔ68. For ΔδCpx(SC-free), we compared 1H-15N TROSY-HSQC spectra of 2H,15N-CpxI(26-83) free and bound to SC. (B) Plot of ΔδCpx(SCΔ68-SC) vs ΔδCpx(SC-free). (C and D) Ratio between the intensities of cross-peaks of 1H-15N TROSY-HSQC spectra of 2H,15N-CpxI(26-83) bound to SCΔ68 (C) or SCΔ62 (D) vs those observed for 2H,15N-CpxI(26-83) bound to SC. To correct for small differences in protein concentrations, the cross-peaks intensities measured for each spectra were normalized with a correction factor derived by averaging the cross-peak intensities of the five C-terminal residues (residues 79-83), which were practically unaffected by the synaptobrevin C-terminal truncations. In all the plots shown in A–D, comparisons between chemical shifts or cross-peak intensities were made only for cross-peaks that could be identified in all the relevant spectra based on the assignments available for free and SNARE complexbound Cpx(26-83) (Figure 2A,B) (Chen et al., 2002; Pabst et al., 2000) and the progressive movements caused by truncations in the SNARE complex (see Figure 2C).
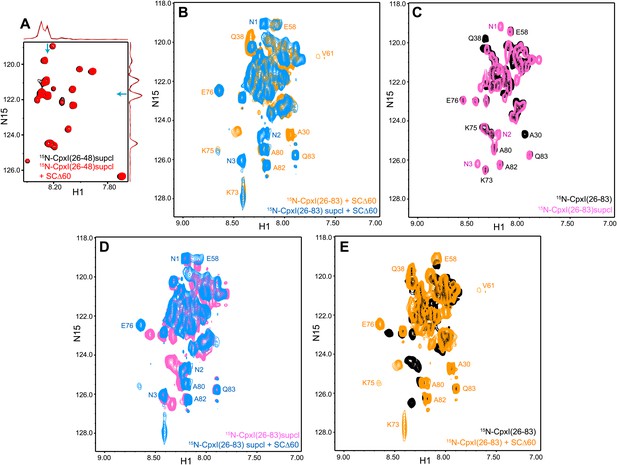
NMR analysis of interactions between 2H,15N-labeled CpxI superclamp mutant fragments and synaptobrevin-truncated SNARE complexes.
(A) Expansions of 1H-15N TROSY-HSQC spectra of D27L, E34F, R37A superclamp mutant (supcl) 2H,15N-CpxI(26-48) in the absence (black contours) or presence (red contours) of SCΔ60. Because the red and black spectra are practically identical, the black spectrum was plotted at slightly lower levels to facilitate observation of the black cross-peaks behind the red ones. However, the intensities of all the cross-peaks were the same in the black and red spectra within experimental error, as illustrated by the one-dimensional traces shown above and on the right of the two-dimensional contour plots (taken at the chemical shifts indicated by the blue arrows). (B–E) Expansions of 1H-15N TROSY-HSQC spectra of WT 2H,15N-CpxI(26-83) free (black contours) or bound to SCΔ60 (orange contours), and of D27L, E34F, R37A superclamp mutant 2H,15N-CpxI(26-83) free (pink contours) or bound to SCΔ60 (blue contours). The minimal contour levels of the different spectra were adjusted to enable visualization of the weakest crosspeaks of interest; hence, cross-peak intensities are not comparable in general. However, the spectra of panel (D) were plotted at the same contour levels to allow direct comparison of crosspeak intensities for free and SCΔ60-bound 2H,15N-CpxI(26-83)supcl. Selected well-resolved cross-peaks are labeled in the different panels. Note that no cross-peak from CpxI(26-83)supcl overlaps closely with the A30 and Q38 cross-peaks of WT Cpx(26-83) due to the mutations (B and C), and three new well-resolved cross-peaks are observed for CpxI(26-83)supcl (labeled N1–N3 in panels B–D). Cross-peaks N1–N3 must belong to the accessory helix where the three mutations were made and can be tentatively assigned to Q38, A30 and A37, respectively, based on their proximity to WT cross-peaks or the observed 15N chemical shift (for A37). The overall changes caused by SCΔ60 binding are similar for WT and superclamp mutant CpxI(26-83) (B, D, E), including the effects on the intensities of the cross-peaks from the accessory helix.
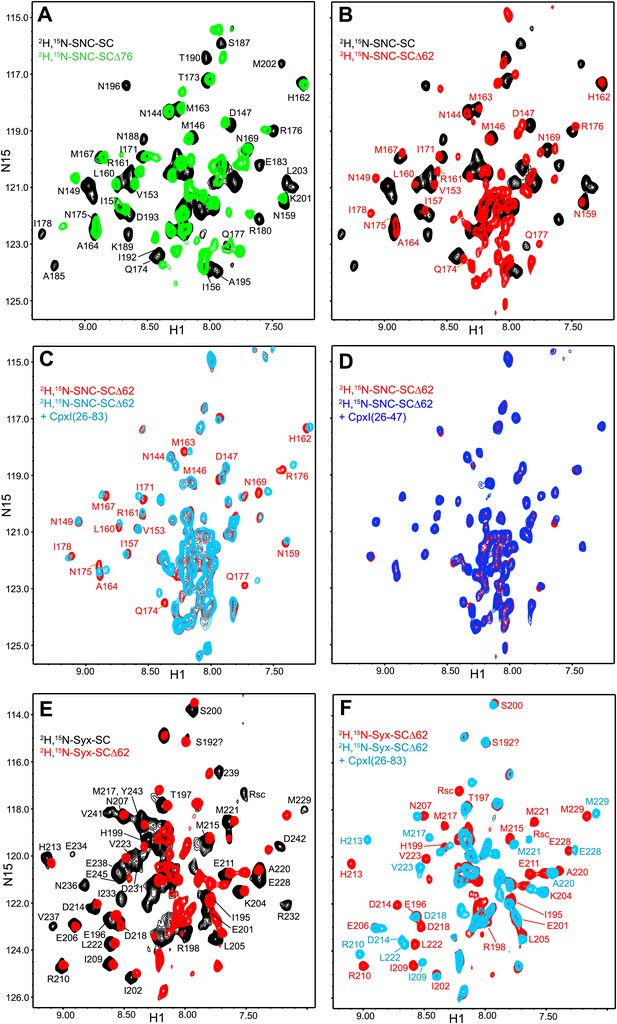
NMR analysis of interactions between 2H,15N-labeled synaptobrevin-truncated SNARE complexes and CpxI fragments.
(A and B) Expansions of 1H-15N TROSY-HSQC spectra of the non-truncated 2H,15N-SNC-SC (black contours), 2H,15N-SNC-SCΔ76 (green contours) and 2H,15N-SNC-SCΔ62 (red contours). (C and D) Expansions of 1H-15N TROSY-HSQC spectra of 2H,15N-SNC-SCΔ62 alone (red contours) or in the presence of CpxI(26-83) (light blue; C) or CpxI(26-47) (dark blue; D). (E and F) Expansions of 1H-15N TROSY-HSQC spectra of 2H,15N-Syx-SC (black contours) and of 2H,15N-Syx-SCΔ62 in the absence (red contours) or presence (light blue contours) of CpxI(26-83). Cross-peak assignments are based on those described for the non-truncated SNARE complex (Chen et al., 2002; Chen et al., 2005).
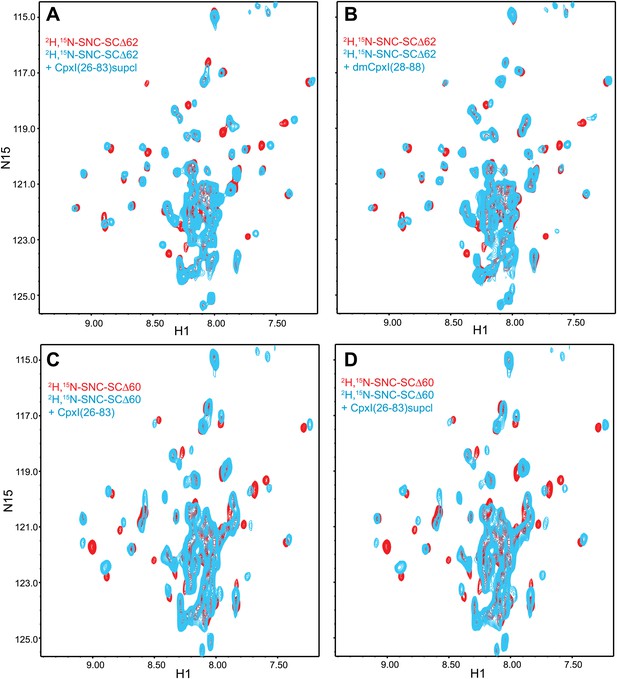
Additional NMR analyses of interactions between 2H,15N-labeled synaptobrevin-truncated SNARE complexes and CpxI fragments.
Expansions of 1H-15N TROSY-HSQC spectra of 2H,15N-SNCΔ62 (A and B) or 2H,15N-SNCΔ60 (C and D) in the absence (red contours) or presence (blue contours) of CpxI(26-83) D27L, E34F, R37A superclamp mutant (supcl) (A and D), dmCpx(28-88) (B), or CpxI(26-83) (C).
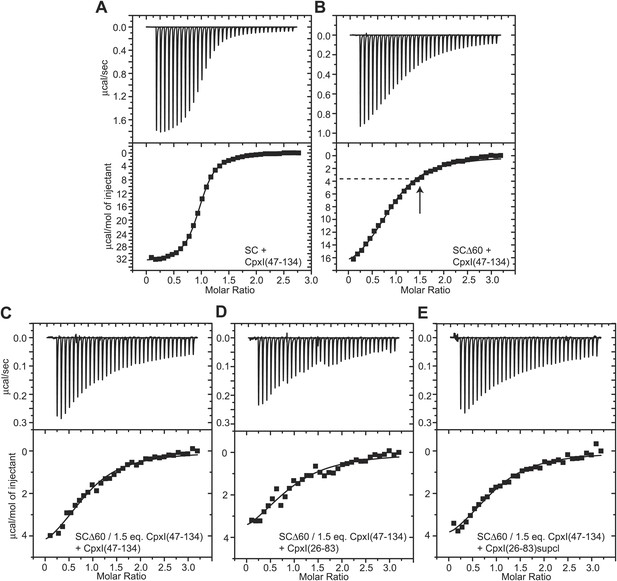
ITC analysis of binding of CpxI fragments to SNARE complexes.
(A and B) Direct titrations of non-truncated SNARE complex (SC; A) or SCΔ60 (B) with CpxI(47-134). (C–E) Competition assays where samples containing SCΔ60 and 1.5 equivalents of CpxI(47-134) were titrated with CpxI(47-134) (C), WT CpxI(26-83) (D) or CpxI(26-83) D27L, E34F, R37A superclamp mutant (supcl) (E). The arrow in panel (B) shows the point of the direct titration where 1.5 equivalents of Cpx(47-134) had been added, and the dashed line shows the heat measured at that point of the titration. The same heat (within experimental error) was measured at the start of the competition experiments of panels (C–E). Thus, the heat measurements in the competition assays correspond to the completion of the titration (i.e., the tail of the direct titration of panel B) because 1.5 equivalents of CpxI(47-134) were not sufficient to saturate SCΔ60.
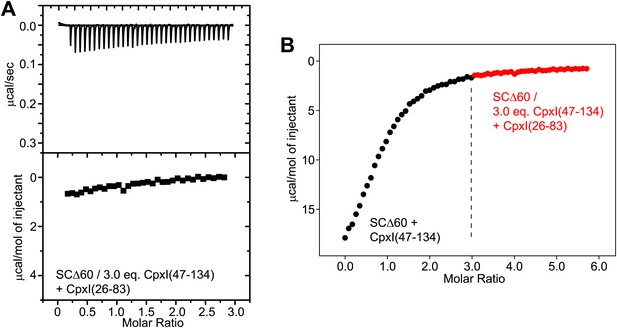
Additional ITC analysis of binding of CpxI fragments to SNARE complexes.
(A) Competition assay where a sample containing SCΔ60 and 3.0 equivalents of CpxI(47-134) was titrated with WT CpxI(26-83). (B) Composite diagram where the ITC data shown in panel (A) (shown here as red circles) was shifted by 3.0 molar ratio units in the x axis and plotted together with ITC data obtained in a direct titration of SCΔ60 with CpxI(47-134), shown by black circles (analogous to that shown in Figure 4B). Note that the data in red constitute a natural continuation of the direct titration of the central helix binding site and hence there is not additional detectable heat caused by binding of the accessory helix of Cpx(26-83) to the groove within SCΔ60.
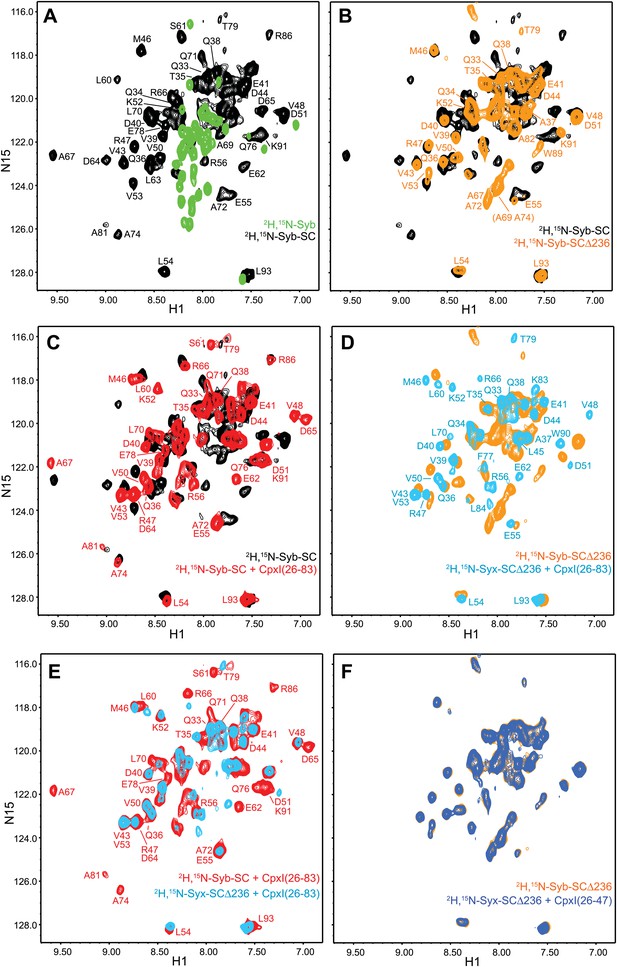
NMR analysis of interactions between 2H,15N-labeled syntaxin-1 truncated SNARE complexes and CpxI fragments.
(A and B) Expansions of 1H-15N TROSY-HSQC spectra of free 2H,15N-labeled synaptobrevin SNARE motif (2H,15N-Syb, green contours), the non-truncated 2H,15N-Syb-SC (black contours) and 2H,15N-Syb-SCΔ236 (orange contours). (C) Expansions of 1H-15N TROSY-HSQC spectra of 2H,15N-Syb-SC in the absence (black contours) and presence (red contours) of CpxI(26-83). (D) Expansions of 1H-15N TROSY-HSQC spectra of 2H,15N-Syb-SCΔ236 in the absence (orange contours) and presence (light blue contours) of CpxI(26-83). (E) Superposition of expansions of 1H-15N TROSY HSQC spectra of 2H,15N-Syb-SC (red contours) and 2H,15N-Syb-SCΔ236 (light blue contours) bound to CpxI(26-83). (F) Expansions of 1H-15N TROSY-HSQC spectra of 2H,15N-Syb-SCΔ236 in the absence (orange contours) and presence (dark blue contours) of CpxI(26-47). Cross-peaks assignments for 2H,15N-Syb-SC free and bound to CpxI(26-83) were described previously (Chen et al., 2002). Cross-peaks assignments for 2H,15N-Syb-SCΔ236 that were not immediately clear from those obtained for 2H,15N-Syb-SC were obtained using triple resonance experiments.
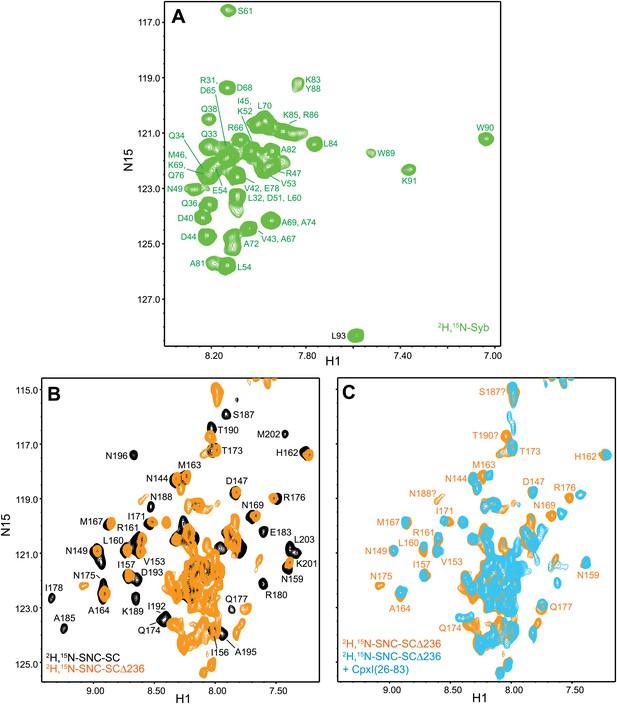
Additional NMR analyses of interactions between 2H,15N-labeled syntaxin-1 truncated SNARE complexes and CpxI fragments.
(A) Expansion of a 1H-15N TROSY-HSQC spectrum of free 2H,15N-labeled synaptobrevin SNARE motif (2H,15N-Syb). Cross-peak assignments are based on those obtained for synaptobrevin(1-96) (Hazzard et al., 1999). (B) 1H-15N TROSY-HSQC spectra of the nontruncated 2H,15N-SNC-SC (black contours) and 2H,15N-SNC-SCΔ236 (orange contours). (C) Expansions of 1H-15N TROSY-HSQC spectra of 2H,15N-SNC-SCΔ236 in the absence (orange contours) and presence (light blue contours) of CpxI(26-83). Cross-peaks assignments for 2H,15N-Syb-SC free were described previously (Chen et al., 2005).
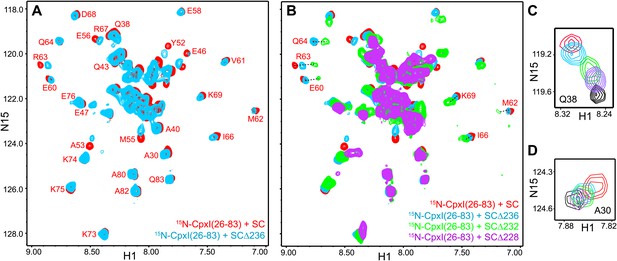
NMR analysis of interactions between 2H,15N-labeled CpxI fragments and syntaxin-1-truncated SNARE complexes.
(A and B) Expansions of 1H-15N TROSY-HSQC spectra of 2H,15N-CpxI(26-83) bound to nontruncated SNARE complex (SC; red contours), to SCΔ236 (blue contours), SCΔ232 (green contours) or SCΔ228 (purple contours). Cross-peaks assignments for CpxI(26-83) bound to nontruncated SNARE complex were described previously (Chen et al., 2002). (C and D) Expansions of the regions containing the cross-peaks of Q38 (C) or A30 (D) of the spectra shown in panels (A and B).
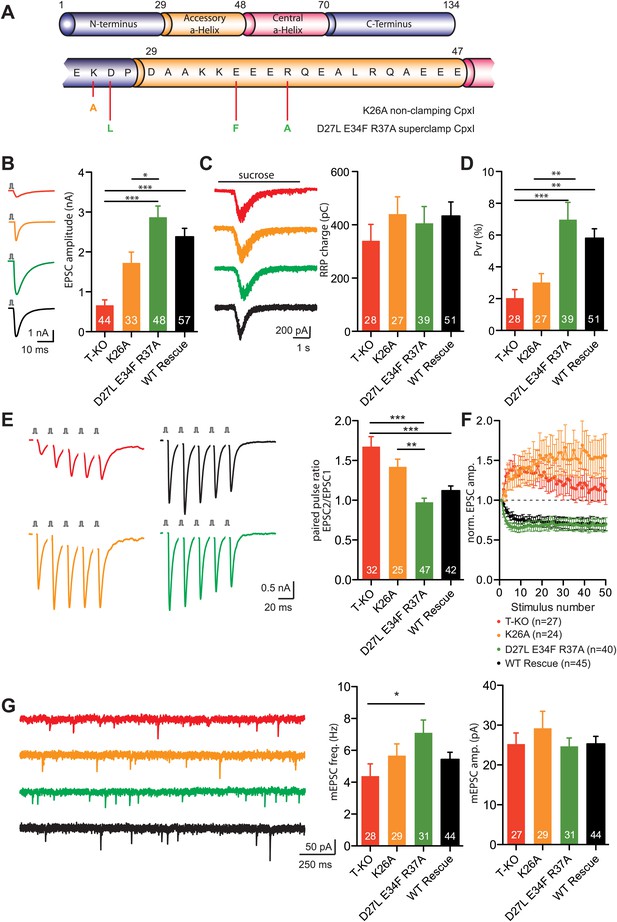
Rescue of the complexin KO phenotype with CpxI-superclamp, but not with a clamping deficient CpxI.
(A) Overview of the introduced mutations in CpxI. (B–C) Representative traces and summary data of evoked EPSC (B) and synaptic responses to hypertonic sucrose solution (RRP) (C) of T-KO, K26A, D27L E34F R37A and WT-CplxI expressing hippocampal neurons. (D) Bar graph of the calculated vesicular release probability Pvr. (E–F) Analysis of short-term plasticity behavior: Example traces of a train of 5 APs at 50 Hz of T-KO, K26A, D27L E34F R37A and WT-CpxI expressing neurons from which the paired pulse ratio was calculated (E) and amplitudes of 50 EPSCs evoked at 10 Hz which were normalized to the first EPSCs and plotted over stimulus number (F). (G) Spontaneous transmitter release: Representative traces of T-KO, K26A, D27L E34F R37A and WT-CpxI expressing neurons and summary data of mEPSC frequency and mEPSC amplitude. Data are expressed as mean ± SEM, *p<0.05; **p<0.01; ***p<0.001. The numbers of neurons analyzed are shown within the bars. Vertical bars in the traces (B and E) represent 2-ms somatic depolarizations; depolarization artifact and action potentials were blanked. Time of sucrose application is indicated as horizontal line (C).
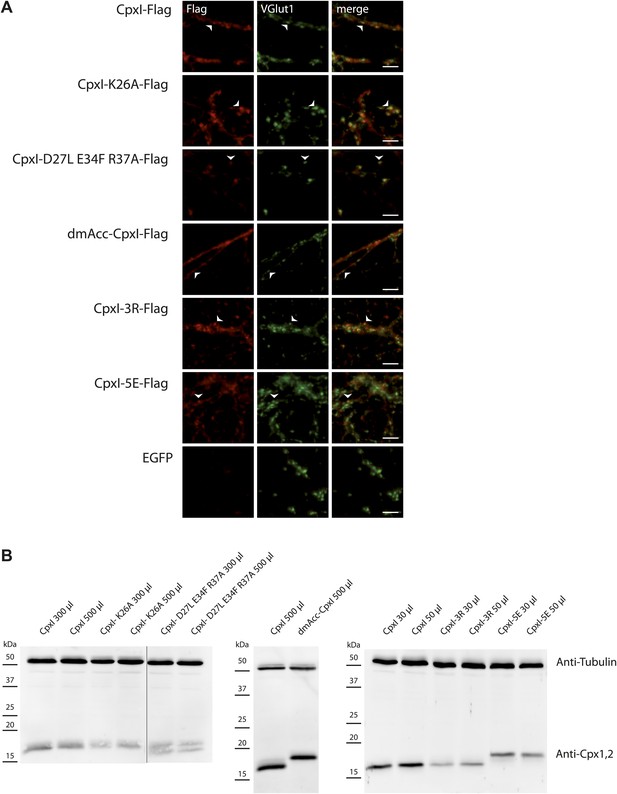
Expression of Cpx variants in hippocampal CPXI-III triple KO neurons by lentiviral transduction.
(A) Immunocytochemical detection of cytosolic complexin-FLAG tagged protein variants within presynaptic compartments illustrated by VGlut1 colocalisation. Arrowheads point towards examples of colocalisation. Scale bar: 5 μm. (B) Protein expression of different Cpx variants used in this study is detected by western blotting. The amounts of viruses to reach similar expression levels in individual virus preparations are indicated. TubulinIII served as loading control.
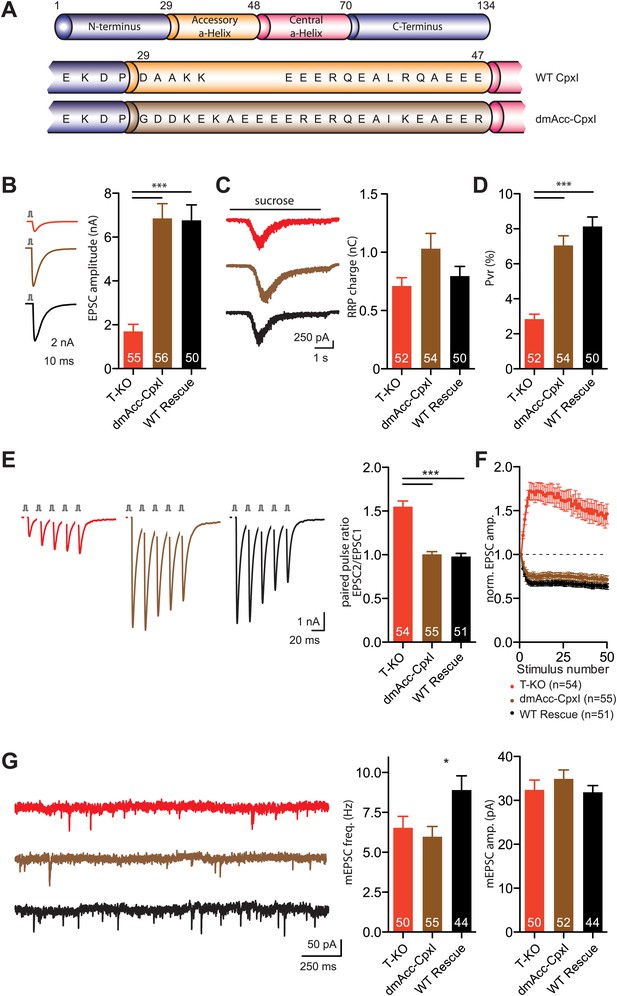
Inhibition of spontaneous release by the accessory alpha helix of dmCpx.
(A) Overview of the replacement of the accessory helix of CpxI with the accessory helix sequence of drosophila Cpx. (B) Representative traces and summary data of evoked EPSC (B) and synaptic responses to hypertonic sucrose solution (RRP) (C) of T-KO, dmAcc-CpxI and WT-CpxI expressing hippocampal neurons. (D) Bar graph of the calculated Pvr. (E–F) Analysis of short-term plasticity behavior: Example traces of a train of 5 APs at 50 Hz of T-KO, dmAcc-CpxI and WT CpxI expressing neurons from which the paired pulse ratio was calculated (E) and amplitudes of 50 EPSCs evoked at 10 Hz which were normalized to the first EPSCs and plotted over stimulus number (F). (G) Spontaneous transmitter release: Representative traces of T-KO, dmAcc-CpxI and WT-CpxI expressing neurons and summary data of mEPSC frequency and mEPSC amplitude. Data are expressed as mean ± SEM, *p<0.05; ***p<0.001. The numbers of neurons analyzed are shown within the bars.
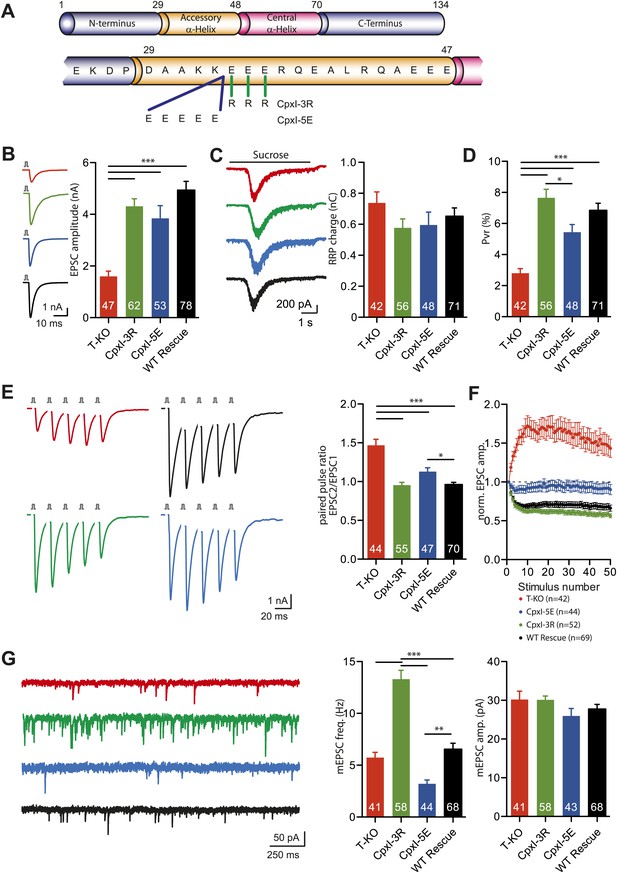
Inhibitory effect of the accessory alpha helix is charge dependent.
(A) Overview of the CpxI accessory alpha helix sequence and the introduced mutations resulting in more positively charged (CpxI-3R) or more negatively charged (CpxI-5E) accessory alpha helix. (B–C) Representative traces and summary data of evoked EPSC (B) and synaptic responses to hypertonic sucrose solution (RRP) (C) of T-KO, CpxI-3R, CpxI-5E and WT-CpxI expressing hippocampal neurons. (D) Bar graph of the calculated Pvr. (E–F) Analysis of short-term plasticity behavior: example traces of a train of 5 APs at 50 Hz of T-KO, CpxI-3R, CpxI-5E and WT-CpxI expressing neurons from which the paired pulse ratio was calculated (E) and amplitudes of 50 EPSCs evoked at 10 Hz which were normalized to the first EPSCs and plotted over stimulus number (F). (G) Spontaneous transmitter release: Representative traces of T-KO, CpxI-3R, CpxI-5E and WT-CpxI expressing neurons and summary data of mEPSC frequency and mEPSC amplitude. Data are expressed as mean ± SEM, *p<0.05; **p<0.01; ***p<0.001. The numbers of neurons analyzed are shown within the bars.
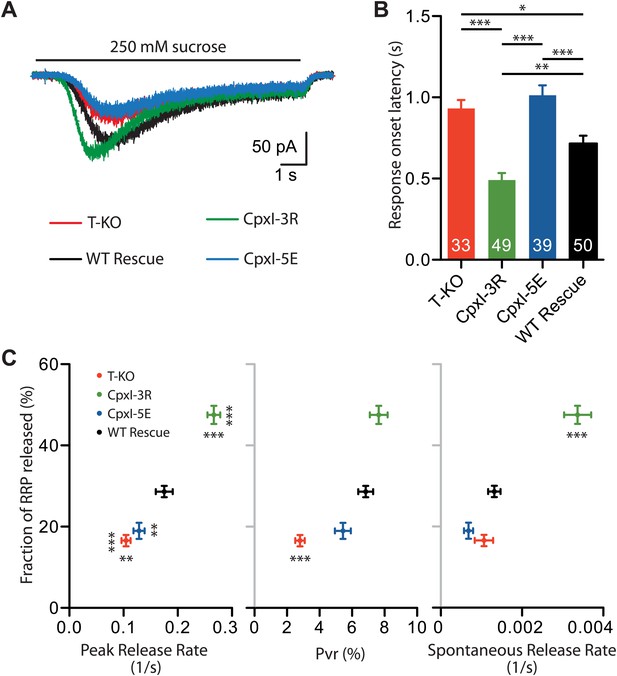
Fusogenicity of synaptic vesicles is influenced by the charge of the accessory alpha helix of CpxI.
(A) Average traces of synaptic responses induced by 250 mM sucrose solution (T-KO n = 33, CpxI-3R n = 49, CpxI-5E n = 39, WT CpxI n = 50). (B) Summary data of 250 mM sucrose solution-induced response onset latency. The numbers of neurons analyzed are shown within the bars. (C) Correlation plot of fraction of RRP released (T-KO n = 33, CpxI-3R n = 49, CpxI-5E n = 39, WT CpxI n = 50) vs peak release rate (T-KO n = 33, CpxI-3R n = 49, CpxI-5E n = 39, WT CpxI n = 50), vesicle release probability (Pvr) (T-KO n = 42, CpxI-3R n = 56, CpxI-5E n = 48, WT CpxI n = 71) and spontaneous release rate (T-KO n = 39, CpxI-3R n = 55, CpxI-5E n = 42, WT CpxI n = 63). Data are expressed as mean ± SEM, *p<0.05; **p<0.01; ***p<0.001. In (C) vertically oriented p values correspond to fraction of RRP and horizontally oriented p values correspond to peak release rate, Pvr and spontaneous release rate compared to WT rescue.