Rapid cell-free forward engineering of novel genetic ring oscillators
Figures
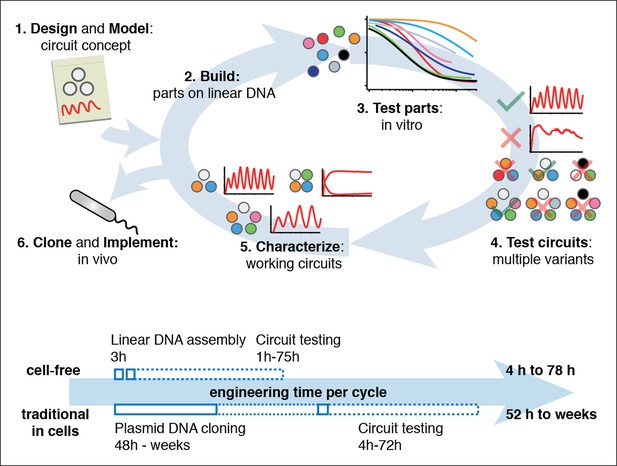
Cell-free systems allow rapid and extensive characterization of biological systems.
Schematic representation of the design-build-test cycle using the cell-free system (top). A design is first modeled to obtain intuition about the architecture. Parts are then assembled on linear DNA without cloning, and tested in vitro. With functional parts, circuit variants can then be tested and working circuits can be extensively characterized. Final circuits are cloned onto plasmids and implemented in vivo. For a specific example of the cell-free system applied to engineering a 5-node oscillator network see Figure 1. Bottom shows a comparison of the time required for testing a genetic circuit by the cell-free approach versus traditional engineering in cells.
-
Figure 1—source data 1
Comparison of a Test Cycle in TX-TL vs. a Test Cycle in traditional prototyping.
Shown are time estimates per step for each process. Assumption is that modular PCR fragments exist that can be assembled into Linear DNA. Parts used from Sun et al., (2014) Supplementary Table S2.
- https://doi.org/10.7554/eLife.09771.004
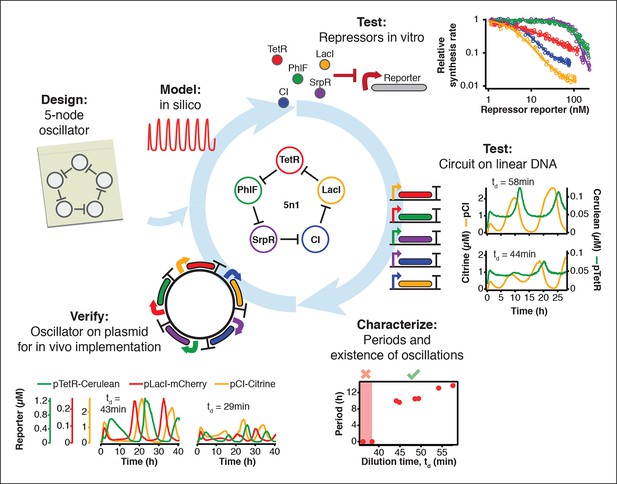
Engineering a 5-node negative feedback oscillator using the cell-free framework.
A novel network architecture, which shows the intended behavior in silico is first assembled on linear DNA using in vitro characterized parts. Initial circuit testing on linear DNA is advantageous because: (I) linear DNA can be synthesized in a few hours, (II) it allows rapid testing of multiple circuit variants, (III) and allows expression strengths of network components to be easily tuned by varying their relative concentrations. A functional circuit can then be further characterized to identify parameter ranges that support the desired behavior and to experimentally test hypotheses. If an in vivo implementation is intended, the cloned plasmids are verified for correct function in vitro before in vivo implementation.
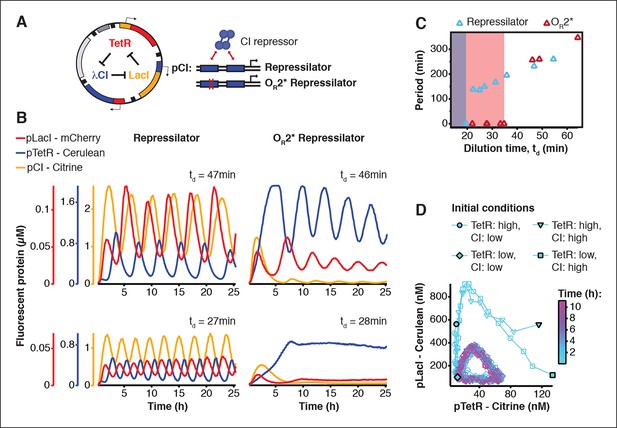
Cell-free repressilator characterization.
(A) Application of cell-free systems to characterize the original repressilator (Elowitz and Leibler, 2000) and a modified version with a point mutation in the CI promoter (OR2*) located in one of the binding sites of the CI repressor. (B) Expression from the three promoters of the repressilator and the OR2* version at different dilution times. (C) Oscillation periods of the repressilator as a function of dilution time. In the OR2* version sustained oscillations were supported in a narrower range of dilution times as compared to the original repressilator network. (D) Phase portrait of repressilator oscillations starting from different initial TetR and CI repressor concentrations.
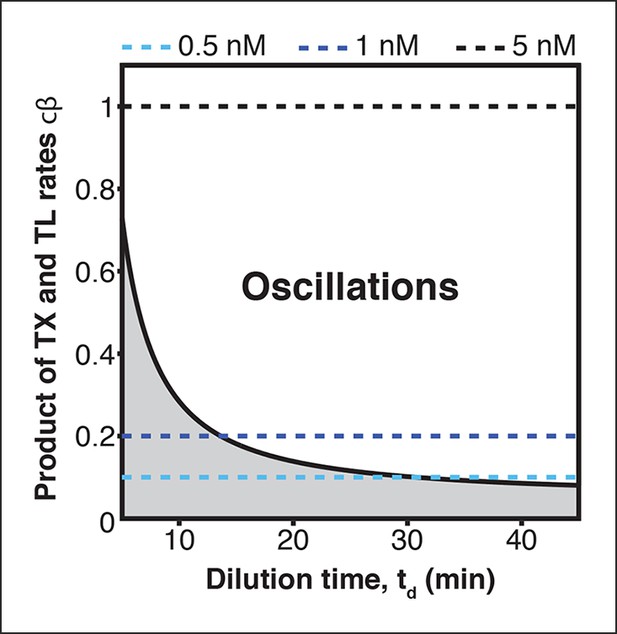
Oscillation parameter regime for a 3-node repressilator network in terms of dilution time and synthesis rates.
Transcription (TX) and translation (TL) rates supporting oscillations at different dilution times for a 3-node repressilator network. Synthesis rates resulting from the plasmid concentrations used in Figure 2—figure supplement 2 are indicated by broken lines.
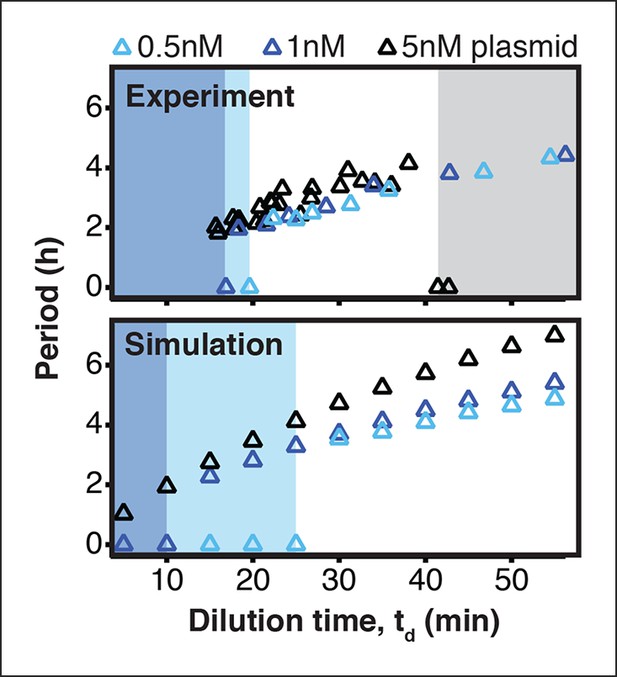
Existence of oscillations and periods for a 3-node repressilator network in terms of dilution time.
We experimentally studied the effect of varying transcription rates on the repressilator network by measuring the range of dilution times that supported sustained oscillations. Transcription rates could be rapidly adjusted by varying DNA template concentrations of the repressilator plasmid. For different DNA template concentrations, oscillations occurred in different ranges of dilution times. Markers at a period of 0 hr indicate a stable steady state, and shaded regions highlight dilution times that did not support oscillations for a specific DNA template concentration. A simulation of the repressilator network produced similar results but did not capture loading effects on the biosynthetic machinery for high DNA template concentrations.
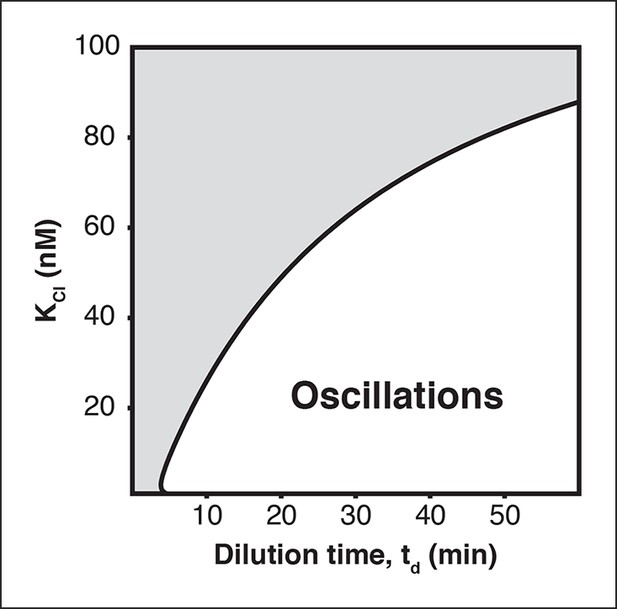
Oscillation parameter regime for a 3-node repressilator network in terms of dilution time and repressor binding affinity
Increasing the KM value, the repressor concentration needed for half-maximal repression, of one repressor, as for CI repressor in the OR2* repressilator version, reduces the range of dilution rates that support oscillations as indicated by our experimental results (Figure 2C).
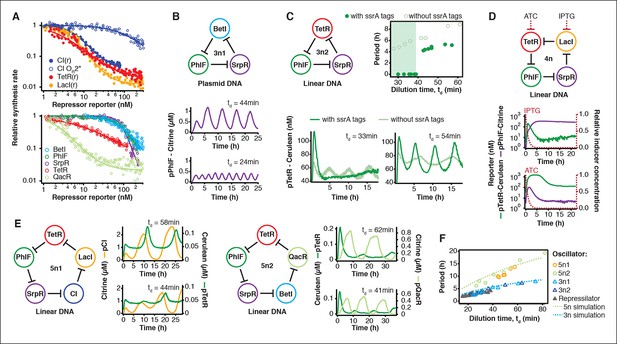
Cell-free prototyping and characterization of novel negative feedback circuits.
(A) Transfer functions of the repressilator repressor-promoter pairs (top) and TetR homologs (bottom). The TetR repressor was tested against two different promoters: the promoter used in the repressilator (top panel) and the J23119-TetR promoter (Stanton et al., 2014) (bottom panel). Lines are Hill function fits. (B) Oscillations of a novel 3-node ring oscillator (3n1) constructed on plasmid DNA. (C) Two versions of a second 3-node ring oscillator (3n2) on linear DNA were used to study the effect of ClpXP degradation on oscillator function. One version was ssrA-tagged on all repressor genes while the other version did not carry degradation tags on the repressors. The same reporter with a medium-strength degradation tag was used in both versions. (D) A 4-node cyclic negative feedback network on linear DNA has two stable steady states that depend on the initial conditions. IPTG switched the network into the state where pPhlF was on and pTetR off. An initial pulse of aTc resulted in the opposite stable steady state. (E) Oscillations of two novel 5-node ring oscillators (5n1, 5n2) constructed on linear DNA. (F) 5-node ring oscillators oscillate with longer periods than 3-node ring oscillators, as predicted by simulations (Materials and methods) and shown by experimental data.
-
Figure 3—source data 1
Transfer function parameters.
Parameter values of repressor – promoter pairs were determined by fitting to the Hill equation (Materials and methods). Promoter sequences were taken from the references cited.
- https://doi.org/10.7554/eLife.09771.012
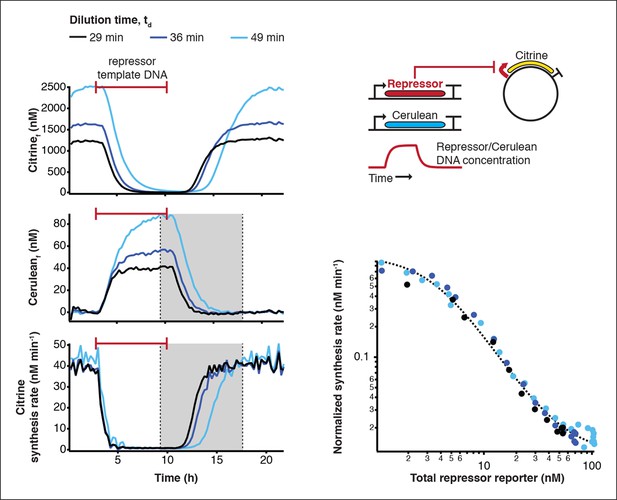
Measurement of transfer functions.
Transfer functions of the repressor – promoter pairs were determined using the cell-free framework (Materials and methods). Shown are experimental results and analysis using LacI - pLacI(r) as an example. Synthesis rates from the promoter of interest could be followed by Citrine fluorescence. Varying repressor template DNA concentration over time allowed us to determine synthesis rates at different repressor concentrations. Cerulean was co-expressed with the repressor and served as reporter for repressor concentration. Transfer functions were obtained by plotting Citrine synthesis rates from highest to lowest repressor concentration (grey shaded area) against total Cerulean concentration and were identical for different dilution times set in the nano-reactor device.
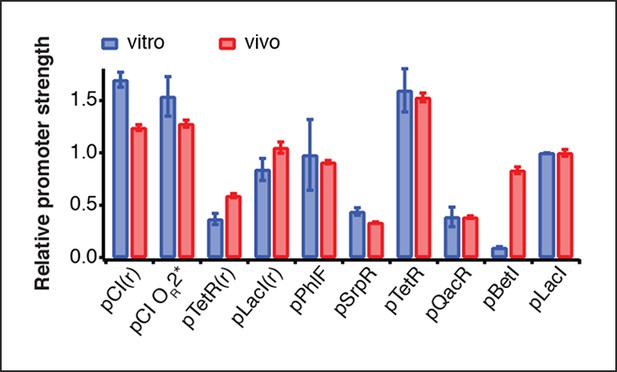
Comparison of relative promoter strengths in vitro and in vivo.
Comparison of relative promoter strengths (vmax), determined in vitro and in vivo. pCI(r), pTetR(r), and pLacI(r) are from (Elowitz and Leibler, 2000); pTetR is from (Stanton et al., 2014) and pLacI from (Lutz and Bujard, 1997). Error bars indicate standard deviations of three replicates.
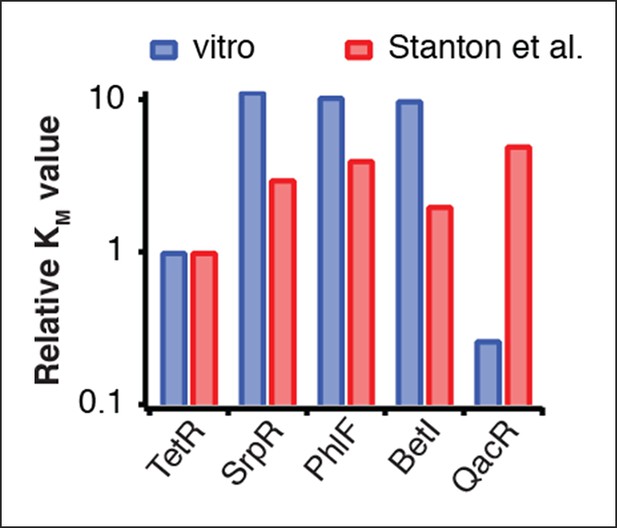
Comparison of half-maximal repressor concentrations needed for repression in vitro and in vivo.
Comparison of KM values measured in vitro in this study with KM values determined in vivo by Stanton et al. (Stanton et al., 2014). KM values were normalized to the KM of TetR.
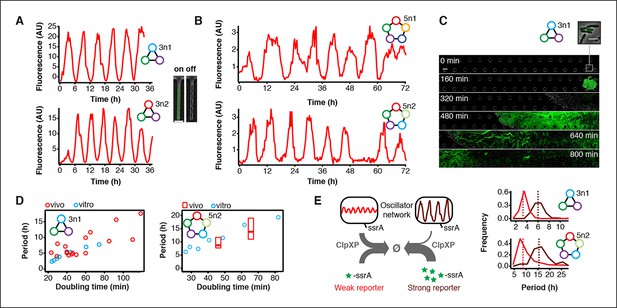
Novel 3-node and 5-node ring oscillators in cells.
(A) Time series traces of 3-node ring oscillators running in E. coli (mother machine). Single trap traces of 3n1 and 3n2 observed for 36 hr in vivo using a strong pPhlF sfGFP-ssrA reporter and a representative image from an ‘on’ and ‘off’ state of oscillation. Scale bar: 5 µm. (B) Time series traces of 5-node ring oscillators running in E. coli (mother machine). Single trap traces of 5n1 and 5n2 observed for 72 hr in vivo using a weak pPhlF sfGFP-ssrA reporter. (C) 3n1 displays population-wide oscillation pulses in vivo (CellASIC). Time series micrographs of 3n1 under a strong pPhlF sfGFP-ssrA reporter every 160 min; inset shows individual cells of the initial microcolony. Scale bar: 10 µm and 5 µm (inset). (D) Relationship between period and division time in vivo. Left, 3n1 in vivo under a strong pPhlF sfGFP-ssrA reporter. The in vitro data is shown for comparison. Each point in the in vivo data corresponds to the period and division time from aCellASIC experiment run under different media type and flow rates. Right, 5n2 in vivo under aweak pPhlF sfGFP-ssrA reporter. In vivo periods determined at 29ºC and 21ºC growth temperature in mother machine experiments. Boxes represent the inner quartile range with the median. (E) Influence of reporter concentration on oscillation periods by competing for ClpXP degradation. Left, with constant amounts of ClpXP the reporter concentration affects repressor degradation and thus oscillation period. Histograms of the periods observed with a weak and a strong pPhlF sfGFP-ssrA reporter for both 3n1 and 5n2 run in the mother machine. Dashed lines indicate the medians.
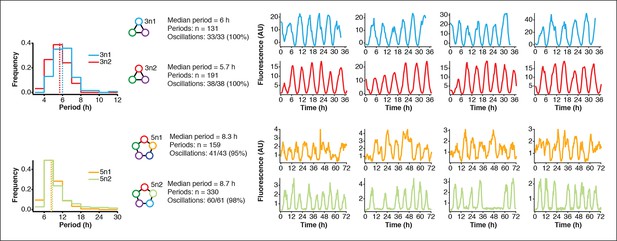
Robust oscillations of 3-node and 5-node oscillators in vivo.
3-node (top) and 5-node networks (bottom) oscillate with periods that depend on the network size in vivo. Shown are the distributions of observed period lengths with medians indicated by dashed lines. Both 3-node and 5-node networks exhibited robust oscillation with all growing cells oscillating for the 3-node networks and more than 95% of growing cells oscillating for the 5-node networks (defined as at least two distinct oscillation peaks per trace). Shown are four example traces for all oscillators in addition to the ones shown in Figure 3A,B. Both 3-node networks were analyzed using a strong pPhlF sfGFP-ssrA reporter and the two 5-node networks were analyzed using a weak pPhlF sfGFP-ssrA reporter.

Population-level oscillations of 3n2 oscillator in vivo.
3n2 oscillator displays phase synchrony in vivo. 3n2 is run under a strong pPhlF sfGFP-ssrA reporter in the CellASIC microfluidic device. Scale bar: 10 µm.

Three-color oscillations and population-level oscillations of 3n2 oscillator in vivo.
3n2 displays phase synchrony observing 3 reporters simultaneously. Reporters are a strong pPhlF Citrine-ssrA, pTetR mCherry-ssrA, and pSrpR Cerulean-ssrA. Shown is one oscillation cycle. Scale bars: 10 µm.
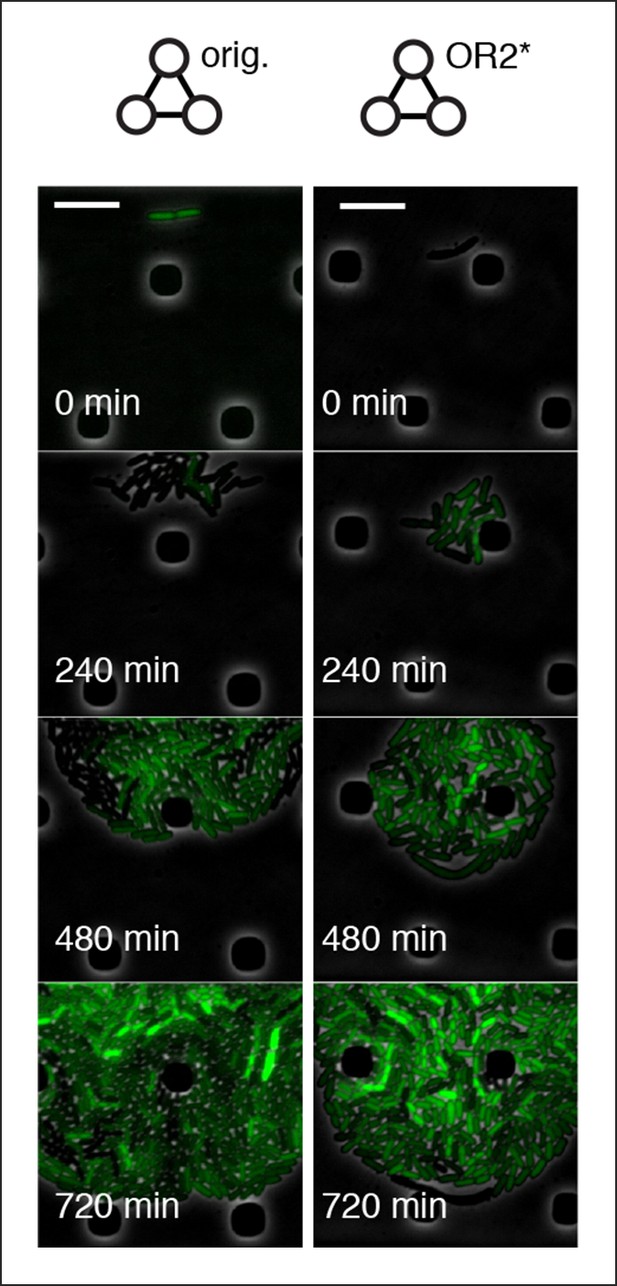
Original repressilator and OR2* mutant repressilator do not show population-level oscillations.
Original repressilator and OR2* repressilator do not show phase synchrony. These are run under pTetR(r)-eGFP(ASV) in M9 minimal media; oscillations were not supported in LB. Scale bars: 10 µm.
Videos
3-color in vitro run of repressilator at td = 47 min.
Shown on the right are individual channels of pCI-Citrine-ssrA, pTetR-Cerulean-ssrA, and pLacI-mCherry-ssrA. These are combined in the composite at the left.
3n1 in mother machine, single trap.
3n1 using a pPhlF-BCD20-sfGFP-ssrA (strong) reporter is run in the mother machine at 29ºC in LB.
3n2 in mother machine, single trap.
3n2 using a pPhlF-BCD20-sfGFP-ssrA (strong) reporter is run in the mother machine at 29ºC in LB.
Run of a panel of 3n2 oscillators in mother machine, using pPhlF-BCD20-sfGFP-ssrA (strong) reporter.
https://doi.org/10.7554/eLife.09771.0235n1 in mother machine.
5n1 using a pPhlF-BCD22-sfGFP-ssrA (weak) reporter is run in the mother machine at 29ºC in LB.
5n2 in mother machine.
5n2 using a pPhlF-BCD22-sfGFP-ssrA (weak) reporter is run in the mother machine at 29ºC in LB.
Run of a panel of 5n2 oscillators in mother machine, using pPhlF-BCD22-sfGFP-ssrA (weak) reporter.
https://doi.org/10.7554/eLife.09771.0263n1 in CellASIC. 3n1 using a pPhlF-BCD20-sfGFP-ssrA (strong) reporter is run in CellASIC.
This video corresponds to Figure 4C. Conditions: 29º°C, LB media, 12.5% lamp intensity, 200 ms exposure, 2 psi flow rate.
3n2 in CellASIC. 3n2 using a pPhlF-BCD20-sfGFP-ssrA (strong) reporter is run in CellASIC.
This video corresponds to Figure 4—figure supplement 2. Conditions: 29ºC, LB media, 12.5% lamp intensity, 200 ms exposure, 2 psi flow rate.
3n2 with 3-color output run in CellASIC.
3n2 using a pPhlF-BCD20-Citrine-ssrA, pTetR-BCD20-mCherry-ssrA, pSrpR-Cerulean-ssrA (strong) reporter is run in CellASIC. This video corresponds to Figure S5B. Conditions: 29 C, LB media, 12.5% lamp intensity, 200 ms exposure for Citrine and Cerulean (500 ms for mCherry), 2 psi flow rate.
Tables
Stoichiometry and reaction rates.
Description | Reaction | Reaction rate |
---|---|---|
Transcription of Mi | ||
Translation of Mi | ci ri | |
Degradation of Mi | ai ri | |
Degradation of Pi | bi pi |
Parameters used for simulations.
Description | Parameter value | |
---|---|---|
ai | Degradation rate of mRNAs (min-1) | ln (2)/8 (half-life time: 8 min) |
bi | Degradation rate of proteins (min-1) | ln (2)/90 (half-life time: 90 min) |
βi | Transcription rate (nM ⋅· min-1 ⋅· plasmid concentration-1) | 0.4 |
ci | Translation rate (nM ·min-1 · mRNA concentration-1) | 0.5 |
Ki | Michaelis-Menten constant (nM) | 5.0 |
νi | Hill-coefficient | 2.0 |
Additional files
-
Supplementary file 1
Supplementary file 1A-D lists linear and plasmid DNA constructs, strains, and DNA concentrations used in cell-free experiments.
(A) Linear DNAs used in this study. (B) Plasmids used in this study. (C) Strains used in this study. (D) DNA concentrations used in experiments.
- https://doi.org/10.7554/eLife.09771.032
-
Source code 1
Zip folder containing the MATLAB code to produce all simulation results, specifically the periods of 3-node and 5-node networks at different dilution times and the oscillation parameter regimes in terms of synthesis rates, repressor binding affinity and dilution times (Figure 3F, Figure 2—figure supplements 1–3).
- https://doi.org/10.7554/eLife.09771.033