The postsynaptic t-SNARE Syntaxin 4 controls traffic of Neuroligin 1 and Synaptotagmin 4 to regulate retrograde signaling
Abstract
Postsynaptic cells can induce synaptic plasticity through the release of activity-dependent retrograde signals. We previously described a Ca2+-dependent retrograde signaling pathway mediated by postsynaptic Synaptotagmin 4 (Syt4). To identify proteins involved in postsynaptic exocytosis, we conducted a screen for candidates that disrupted trafficking of a pHluorin-tagged Syt4 at Drosophila neuromuscular junctions (NMJs). Here we characterize one candidate, the postsynaptic t-SNARE Syntaxin 4 (Syx4). Analysis of Syx4 mutants reveals that Syx4 mediates retrograde signaling, modulating the membrane levels of Syt4 and the transsynaptic adhesion protein Neuroligin 1 (Nlg1). Syx4-dependent trafficking regulates synaptic development, including controlling synaptic bouton number and the ability to bud new varicosities in response to acute neuronal stimulation. Genetic interaction experiments demonstrate Syx4, Syt4, and Nlg1 regulate synaptic growth and plasticity through both shared and parallel signaling pathways. Our findings suggest a conserved postsynaptic SNARE machinery controls multiple aspects of retrograde signaling and cargo trafficking within the postsynaptic compartment.
https://doi.org/10.7554/eLife.13881.001eLife digest
Synapses are connections that allow a neuron to communicate with a neighboring cell (often another neuron). When an electrical impulse traveling down the “presynaptic” neuron reaches the synapse, it causes the neuron to release molecules called neurotransmitters. These molecules then bind to receptors on the surface of the other “postsynaptic” cell and cause that cell to respond in a particular way.
Communication between the two cells at the synapse can also go in the opposite direction, with the postsynaptic cell signaling to the presynaptic cell. Such “retrograde” signals typically regulate the properties of the synaptic connection, such as changing the strength or shape of the synapse, or altering which proteins are present there.
While a lot is known about how a presynaptic neuron communicates with the postsynaptic cell, not as much is known about how retrograde signals are regulated. Harris et al. therefore set out to identify and characterize new factors that control retrograde signaling, and started by producing a list of likely candidate molecules. These candidates were then screened by removing them one at a time from the synapses between motor neurons and muscle cells in fruit flies and observing the effect this had on a molecule called Synaptotagmin 4.
Synaptotagmin 4 is normally found at the membrane of the postsynaptic cell. Harris et al. found that removing one candidate molecule, called Syntaxin 4, from the postsynaptic cell reduced the amount of Synaptotagmin 4 at the membrane. Further investigation showed that Syntaxin 4 also helps to deliver a protein called Neuroligin 1 to the postsynaptic membrane, which is important for organizing the synapse.
By identifying Syntaxin 4 as a new regulator of retrograde signaling, Harris et al. open up several avenues of investigation that could reveal more about the mechanisms that influence how synapses work.
https://doi.org/10.7554/eLife.13881.002Introduction
Synaptic connections form and mature through signaling events in both pre- and postsynaptic cells. The release of signaling molecules into the synaptic cleft depends on SNARE proteins that drive membrane fusion. This machinery is well understood for neurotransmitter release from the presynaptic cell: in response to an action potential, a v-SNARE in the synaptic vesicle membrane (Synpatobrevin/VAMP) engages t-SNARES in the presynaptic membrane (Syx1 and SNAP-25), forming a four-helix structure that brings the membranes into close proximity and initiates fusion (Jahn and Scheller, 2006; Südhof and Rothman, 2009). Although SNARE-dependent fusion drives membrane dynamics in all cell types, it is specialized in the presynaptic terminal to be Ca2+-dependent, employing Ca2+ sensors like Synaptotagmin 1 (Syt1) to link synaptic vesicle fusion to Ca2+ influx following an action potential.
The postsynaptic cell also exhibits activity-dependent exocytosis. Altering the composition of the postsynaptic membrane, including regulated trafficking of neurotransmitter receptors, is an important plastic response to neural activity (Chater and Goda, 2014). The postsynaptic cell also releases retrograde signals into the synaptic cleft to modulate synaptic growth and function. These retrograde messengers include lipid-derived molecules like endocannabinoids (Ohno-Shosaku and Kano, 2014), gases like nitric oxide (Hardingham et al., 2013), neurotransmitters (Koch and Magnusson, 2009; Regehr et al., 2009), neurotrophins (Zweifel et al., 2005), and other signaling factors like TGF-β and Wnt (Poon et al., 2013; Salinas, 2005; Sanyal et al., 2004; Speese and Budnik, 2007). Adhesion complexes that provide direct contacts across the synaptic cleft also participate in retrograde signaling (Futai et al., 2007; Gottmann, 2008; Hu et al., 2012; Mozer and Sandstrom, 2012; Peixoto et al., 2012; Vitureira et al., 2012).
Although retrograde signaling is a key modulator of synaptic function, little is known about how postsynaptic exocytosis is regulated and coordinated. Components of a postsynaptic SNARE complex have been recently identified in mammalian dendrites. The t-SNAREs Syntaxin 3 (Stx3) and SNAP-47 are required for regulated AMPA receptor exocytosis during long term potentiation, while the v-SNARE synaptobrevin-2 regulates both activity-dependent and constitutive AMPAR trafficking (Jurado et al., 2013). Stx4 has also been implicated in activity-dependent AMPAR exocytosis (Kennedy et al., 2010). In Drosophila, a Ca2+-dependent retrograde signaling pathway relies on the postsynaptic Ca2+ sensor Syt4. Syt4 vesicles fuse with the postsynaptic membrane in an activity-dependent fashion (Yoshihara et al., 2005), and loss of Syt4 leads to abnormal development and function of the NMJ. Syt4 null animals have smaller synaptic arbors, indicating a defect in synaptic growth, and also fail to exhibit several forms of synaptic plasticity seen in control animals, including robust enhancement of presynaptic release in response to high frequency stimulation, and rapid budding of synaptic boutons in response to strong neuronal stimulation (Barber et al., 2009; Korkut et al., 2013; Piccioli and Littleton, 2014; Yoshihara et al., 2005). However, a detailed understanding of how the postsynaptic cell regulates constitutive and activity-dependent signaling of multiple retrograde pathways is lacking. In addition to exocytosis, it is likely that many cellular processes including vesicle trafficking and polarized transport of protein and transcript are specialized to facilitate postsynaptic signaling. Identifying such regulatory mechanisms is crucial for understanding synaptic development and function.
We conducted a candidate-based transgenic RNAi screen to identify regulators of postsynaptic exocytosis at the Drosophila NMJ, a model for studying glutamatergic synapse growth and plasticity (Harris and Littleton, 2015). Using a fluorescently tagged form of the postsynaptic Ca2+ sensor Syt4, we screened for candidate gene products that disrupted the localization of Syt4 at the postsynaptic membrane. Here we describe our characterization of one candidate from this screen, Syntaxin 4 (Syx4). Drosophila Syx4 is the sole homolog of the mammalian Stx 3/4 family of plasma membrane t-SNAREs that also includes Syntaxin 1 (Littleton, 2000). The mammalian Stx3 and Stx4 homologs regulate activity-dependent AMPA receptor trafficking in mammalian neurons (Jurado et al., 2013; Kennedy et al., 2010), while Stx4 also participates in regulated secretory events in several other mammalian cell types, including insulin-stimulated delivery of the glucose transporter to the plasma membrane in adipocytes and glucose-stimulated insulin secretion from pancreatic beta cells (reviewed by Jewell et al., 2010). Our results demonstrate that the Drosophila Syx4 homolog is essential for retrograde signaling, regulating the membrane delivery of both Syt4 and Neuroligin (Nlg1), a transsynaptic adhesion protein that plays important roles in synapse formation and function, and is linked to autism spectrum disorder (ASD) (Bang and Owczarek, 2013; Bottos et al., 2011; Südhof, 2008). Through genetic interaction experiments, we define functions of the Syx4, Syt4, and Nlg1 pathway in regulating multiple aspects of synaptic growth and plasticity within the postsynaptic compartment.
Results
A candidate RNAi screen for regulators of postsynaptic exocytosis
To identify regulators of Syt4 trafficking, we conducted a candidate-based RNAi screen at the NMJ. Our screening approach employed transgenic animals expressing Syt4 tagged with pHluorin, a pH-sensitive variant of GFP under the control of the UAS promoter (UAS-Syt4-pH). When expressed with the muscle driver 24B-GAL4, Syt4-pH protein decorates the postsynaptic membrane of the NMJ, overlapping with glutamate receptor (GluR) fields opposite active zones (AZs) (Yoshihara et al., 2005; Figure 1A). Syt4-pH is also found in numerous vesicular structures throughout the muscle, many of which overlap with the Golgi marker Lava lamp (Lva) (Figure 1B). Postsynaptic expression of Syt4-pH rescues synaptic phenotypes previously reported in Syt4 null animals (Figure 1—figure supplement 1), including a decrease in the number of synaptic boutons and a decrease in the ability to grow new boutons (“ghost boutons”, or GBs) in response to strong neuronal stimulation (Barber et al., 2009; Korkut et al., 2013; Piccioli and Littleton, 2014; Yoshihara et al., 2005). Thus, Syt4-pH is functional at the NMJ.
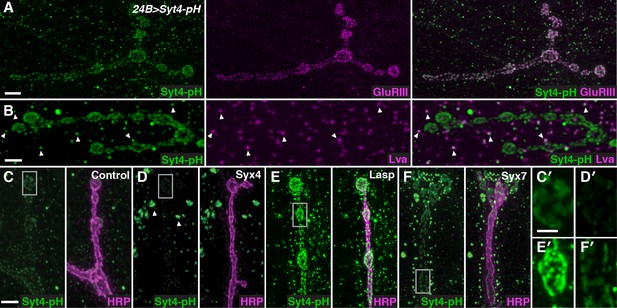
A candidate RNAi screen for regulators of postsynaptic exocytosis.
(A,B) Representative images of Syt4-pH expressed with the postsynaptic muscle driver 24B-GAL4. Syt4-pH (green) accumulates in postsynaptic membrane that also contains domains of GluRIII (magenta) (A). Syt4-pH also decorates numerous cytoplasmic puncta, many of which overlap with the Golgi marker Lva (magenta), arrowheads (B). (C–F) Examples of candidate RNAis affecting Syt4-pH localization: control (C); Syx4-RNAi reduces Syt4-pH at the membrane and causes a redistribution to prominent cytoplasmic puncta, arrowheads (D); Lasp-RNAi increases Syt4-pH at the membrane (E); and Syx7-RNAi causes a redistribution of Syt4-pH puncta around the NMJ without affecting the intensity at the membrane (F). (C′–F′) Close-ups of C–F. Scale bars = 7 μm (A), 5 μm (B–F), 2 μm (C′–F′).
Candidate UAS-RNAi constructs were co-expressed with UAS-Syt4-pH in muscle, and animals were examined for changes in Syt4-pH distribution. We looked for changes in Syt4-pH intensity at the postsynaptic membrane (defined as discreet Syt4-pH fields adjacent to the neuronal membrane), or other changes in the distribution, size or intensity of Syt4-pH-positive vesicular structures. Resolution of Syt4-pH localization was best achieved following tissue fixation, which is expected to interfere with the pH sensitivity of the pHluorin tag. Indeed, treatment of fixed samples with a low pH (5.5) adjusted buffer did not affect our detection of Syt4-pH in fixed tissue, compared to a dramatic quenching of fluorescence that was observed in a live preparation (Figure 1—figure supplement 2). Thus, we interpret the Syt4-pH localization pattern in our fixed-tissue assay as non-pH-dependent.
We assembled a candidate list of gene products resident at synapses and/or involved in membrane trafficking (Supplementary file 1) using the following criteria: 1) Drosophila orthologs of proteins identified in proteomics studies of mouse and rat brain synaptic membranes (Abul-Husn et al., 2009; Li et al., 2007c); 2) candidate genes identified in a Drosophila screen for transposon insertions affecting glutamate receptor expression or localization (Liebl and Featherstone, 2005); and 3) known regulators of membrane trafficking (eg Rabs, SNARE proteins, Vps proteins). Transgenic RNAi lines were obtained from the Transgenic RNAi Project (TRiP) at Harvard Medical School (Perkins et al., 2015) or the Vienna Drosophila RNAi Center (Dietzl et al., 2007). For 190 candidates that had no RNAi stock already available, transgenic RNAi stocks were generated by the TRiP at Harvard Medical School; these stocks are currently available from the Bloomington stock center.
Among the 442 lines screened, 15 candidates were identified with abnormal Syt4-pH distribution (Table 1). These candidates fell into three qualitatively distinct categories based on the intensity of Syt4-pH at the postsynaptic membrane: decreased intensity of Syt4-pH (7/15, Figure 1D,D′, eg. Syx4-RNAi), increased intensity of Syt4-pH (3/15, Figure 1E,E′, eg. Lasp-RNAi), and changes in the distribution of Syt4-pH-positive vesicles with otherwise normal intensity (5/15, Figure 1F,F′, eg. Syx7-RNAi). Two candidates had phenotypes consistent with previously published studies, supporting the efficacy of the screen: knockdown of Syx18/Gtaxin dramatically reduced Syt4 delivery, consistent with a role in postsynaptic membrane addition (Gorczyca et al., 2007), and knockdown of β-spectrin resulted in changes in the spacing of Syt4-pH domains, consistent with a role for the spectrin cytoskeleton in AZ/GluR spacing (Pielage et al., 2006). We chose to focus our analysis on one candidate, the plasma membrane t-SNARE Syx4. Knockdown of Syx4 produced a decrease in the intensity of Syt4-pH at the postsynaptic membrane, along with large accumulations of Syt4-pH in the cytoplasm (Figure 1D,D′), suggesting that Syx4 may regulate membrane levels of Syt4 and modulate Syt4-dependent signaling mechanisms.
RNAis that alter the localization of Syt4-pH Candidate gene products are listed, along with the predicted gene function, the specific effect on Syt4-pH, and the RNAi constructs tested. RNAi lines were obtained from the Transgenic RNAi Project (TRiP) at Harvard Medical School (Perkins et al., 2015)a or the Vienna Drosophila RNAi Center (Dietzl et al., 2007)b.
Gene product | CG | Function | Syt4-pH distribution | RNAis |
---|---|---|---|---|
Syntaxin 4 | CG2715 | t-SNARE | Reduced intensity at NMJ, large clusters in cytoplasm | JF01714a V32413b |
Syntaxin 6 | CG7736 | t-SNARE | Reduced intensity at NMJ, large clusters in cytoplasm | V1579b V1501b |
Syntaxin 18 (Gtaxin) | CG13626 | t-SNARE | Reduced intensity at NMJ, large clusters in cytoplasm | JF02263a |
MyoV | CG2146 | Dilute class unconventional myosin | Reduced intensity at NMJ, large clusters in cytoplasm | JF03035a V16902b |
Actin-related protein 2/3 complex, subunit 3A | CG4560 | Arp2/3 complex-mediated actin nucleation | Reduced intensity and size at NMJ, smaller cytoplasmic puncta | JF02370a |
Gdi | CG4422 | Rab GDP-dissociation inhibitor | Reduced intensity at NMJ, large clusters in cytoplasm | JF02617a V26537b |
Rabex-5 | CG9139 | Rab5 guanyl-nucleotide exchange factor activity | Reduced intensity at NMJ, large clusters in cytoplasm | JF02521a |
Lasp | CG3849 | Actin binding | Increased intensity at NMJ | JF02075a |
Neuroglian | CG1634 | Cell adhesion; axon guidance; synapse organization | Increased intensity at NMJ | JF03151a V27201b |
Contactin | CG1084 | Cell adhesion | Increased intensity at NMJ | HM05134a HMS00186a |
Syntaxin 7 | CG5081 | t-SNARE, early endosomal regulation | Intensity at NMJ normal, many small bright puncta cluster adjacent to NMJ | JF02436a V5413b |
Dynamin associated protein 160 | CG1099 | Synaptic vesicle endocytosis; cell polarity | Intensity at NMJ normal, many small bright puncta cluster adjacent to NMJ | JF01918a V16158b |
Adaptor Protein complex 2, σ subunit | CG6056 | Endocytosis | Intensity at NMJ normal, many small bright puncta cluster adjacent to NMJ | JF02631a |
Adaptor Protein complex 2, α subunit | CG4260 | Endocytosis | Intensity at NMJ normal, many small bright puncta cluster adjacent to NMJ | HMS00653a |
β-spectrin | CG5870 | Cytoskeleton; synapse organization | Irregular size and spacing at NMJ | HMS01746a V42053b |
Syntaxin 4 is enriched postsynaptically at the NMJ
To investigate the function of Syx4, we created mutant alleles by mobilizing a transposable P-element located in the 5’-UTR of the Syx4 locus (Figure 2A). Syx4 encodes a protein with a large N-terminal domain, a SNARE domain, and a C-terminal transmembrane domain (Figure 2B). Two Syx4 proteins (Syx4A and Syx4B) are predicted from genome analysis, resulting in a longer (A) or shorter (B) N-terminus. RT-PCR analysis indicated that both of these isoforms are expressed in Drosophila larvae (Figure 2—figure supplement 1).
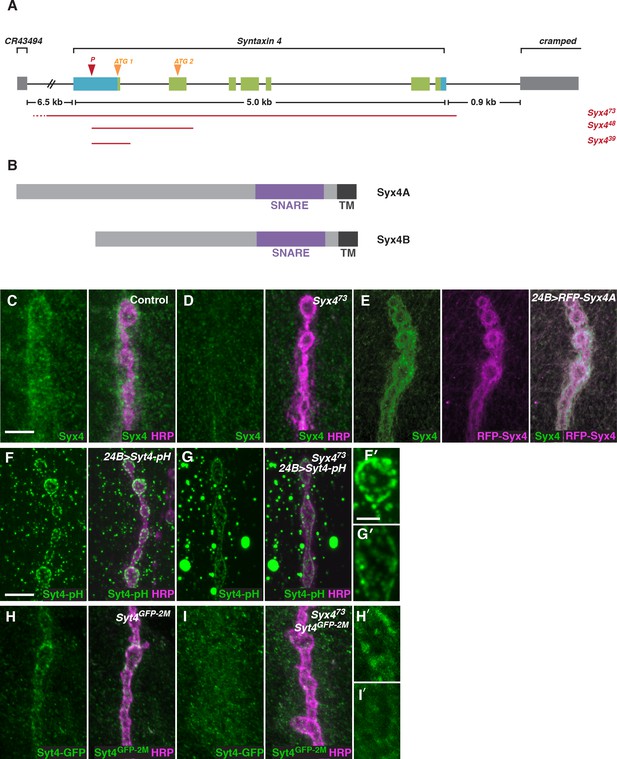
Syntaxin 4 is a postsynaptic plasma membrane SNARE.
(A) Syx4 genomic region. Coding exons are indicated in green while non-coding exons are in blue. Two predicted start sites (ATG) are indicated in orange. The location of the P-element used for mutagenesis (P) is indicated in red. Three alleles of Syx4 were isolated. Deleted regions are indicated in red. Solid lines indicate regions known to be deleted from PCR analysis and sequencing, while dotted lines indicate regions within which breakpoints have been mapped. (B) Syx4 encodes a protein with an N-terminal domain, a SNARE domain and a C-terminal transmembrane domain. There are two predicted isoforms that differ in the size of the N-terminal domain. (C,D) Representative images of NMJs stained for Syx4 (green) and the neuronal membrane marker HRP (magenta). Syx4 staining at the synapse in precise excision control animals (C) is absent in Syx473 mutant animals (D). (E) Representative image from an animal stained for Syx4 (green) and expressing RFP-Syx4 (magenta) with 24B-GAL4. (F,G) Representative images from animals expressing Syt4-pH with 24B-GAL4 in a control (F) or Syx473 (G) background. Syt4-pH is reduced at the postsynaptic membrane and redistributed to large cytoplasmic accumulations in Syx473 mutants. (F′,G′) Close-ups of F and G. (H,I) Representative images from Syt4GFP-2M knock-in animals in a control (H) or Syx473 (I) background. Synaptic localization of Syt4GFP-2M is reduced in Syx473 mutants. (H′,I′) Close-ups of H and I. Scale bars = 5 μm (C–I), 2 μm (F′,G′,H′,I′).
We isolated three alleles that delete parts of the Syx4 coding region. Syx439 carries a deletion from the 5’-UTR to the first intron, removing the first exon and the start site for the Syx4A isoform. Syx448 carries a deletion from the 5’-UTR to the second intron, removing the first two exons and both predicted start sites. Finally, Syx473 carries a deletion from the 5’-UTR through the entire coding region of the gene. Several lines of evidence discussed below indicate Syx473 is a null allele. A precise excision with no deletion was also generated, and was used as a genetic background control.
To examine the subcellular distribution of Syx4, we generated polyclonal antisera against purified Syx4A protein. Syx4 is expressed in the muscle at the NMJ and is enriched postsynaptically, as revealed by co-staining with an antibody against HRP to highlight the presynaptic membrane (Figure 2C). This staining is absent in Syx473 mutants (Figure 2D), consistent with this allele removing the entire coding region of the gene. We also produced UAS-Syx4 and UAS-RFP-Syx4 constructs for both protein isoforms, in order to overexpress untagged or tagged Syx4 at the NMJ. Expression of RFP-Syx4A (Figure 2E) or RFP-Syx4B (data not shown) with the postsynaptic muscle driver 24B-GAL4 showed a similar distribution to the endogenous protein. Thus, Syx4 is expressed in the postsynaptic cell and accumulates at the synaptic membrane.
We also tested the Syx473 allele for its effect on the distribution of Syt4-pH. Similar to our RNAi knockdown results, Syx4 mutants exhibited a decrease in Syt4-pH intensity at the NMJ, accompanied by a redistribution of Syt4-pH to large cytoplasmic accumulations (Figure 2F,G,F′,G′).
To test whether Syx4 regulates the localization of endogenous Syt4, we used CRISPR/CAS9 to generate a C-terminal GFP knock-in line, Syt4GFP-2M. We produced a transgenic stock expressing custom guide RNAs (gRNAs) targeting the Syt4 locus. These animals were crossed to transgenic flies expressing germline-specific Cas9, and embryos from the cross were injected with a donor plasmid for homology-directed repair to insert GFP into the Syt4 genomic locus (Gokcezade et al., 2014; Kondo and Ueda, 2013; Port et al., 2014). The Syt4GFP-2M line is homozygous viable and fertile, and does not exhibit synaptic defects that have been previously described in animals lacking Syt4 (Figure 1—figure supplement 1), indicating that Syt4GFP-2M protein is functional.
Syt4GFP-2M shows synaptic localization at the NMJ (Figure 2H,H′), and this localization is lost in the Syx4 null mutant background (Figure 2I,I′). These findings indicate that the distribution of Syt4-pH reported in our screen is recapitulated by endogenous Syt4 protein. As we do not observe large cytoplasmic accumulations of Syt4GFP-2M in Syx4 mutants, this feature likely results from overexpression of Syt4-pH protein using the 24B-GAL4 driver.
Syntaxin 4 is required postsynaptically to regulate synaptic growth
Because Syx4 mutants have a defect in Syt4 localization, we investigated whether Syx4 impacts synaptic development in a similar manner to Syt4. Syt4 null mutants show abnormal development and function of the NMJ, including a decrease in the number of synaptic boutons, and a failure to express several forms of synaptic plasticity (Barber et al., 2009; Korkut et al., 2013; Piccioli and Littleton, 2014; Yoshihara et al., 2005). We first quantified the number of boutons per NMJ at muscle 6/7 in hemisegment A3. The null allele Syx473 exhibited a strong reduction in the number of synaptic boutons compared to control animals (Figure 3A,B,G). When Syx473 was placed in trans with a large chromosomal deficiency that removed the entire Syx4 locus, a similar phenotype was observed compared to Syx473 mutants alone (Figure 3G), consistent with Syx473 being a null allele. Syx448 animals also exhibited a decrease in bouton number compared to controls (Figure 3C,G), which was less severe than the null mutant. The smallest deletion, Syx439, had no change in bouton number compared to controls (Figure 3D,G). These results indicate that Syx4 is required for normal synaptic bouton number. Furthermore, as the Syx439 allele lacks the start site for Syx4A and does not exhibit any phenotype, we hypothesize that expression of Syx4B from the second start site is sufficient for normal Syx4 function with respect to bouton number.
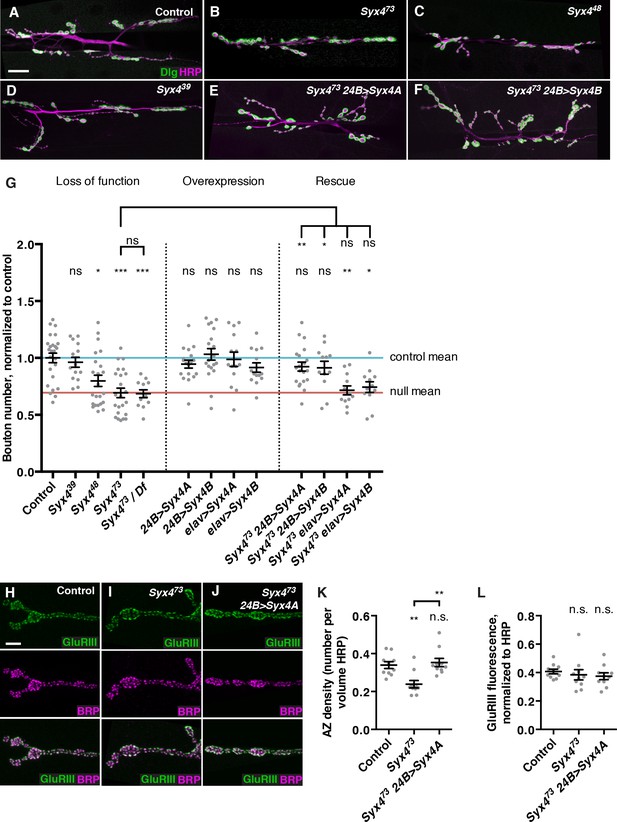
Syntaxin 4 regulates synaptic growth at the NMJ.
(A–F) Representative images of NMJs stained with antibodies to the postsynaptic marker Dlg (green) and the neuronal membrane marker HRP (magenta) to highlight the number of synaptic boutons; images are shown from precise excision control (A), Syx473 (B), Syx448 (C), Syx439 (D), Syx473 24B>Syx4A (E), and Syx473 24B>Syx4B (F) animals. (G) Quantification of bouton number, normalized to controls. Blue line indicates the control mean. Red line indicates Syx473 null mean. Data are presented as mean ± SEM. (H–J), Representative images of NMJs stained with antibodies to GluRIII (green) and the AZ marker Brp (magenta); images are shown from precise excision control (H), Syx473 (I), and Syx473 24B>Syx4A (J) animals. (K), Quantification of AZ density, calculated as the number of AZs per volume HRP. Data are presented as mean ± SEM. (L) Quantification of GluRIII fluorescence per HRP fluorescence. Data are presented as mean ± SEM. Scale bars = 20 μm (A–F), 5 μm (H–J). Statistical comparisons are fully described in Figure 3—source data 1, and are indicated here as ***p<0.001, **p<0.01, *p<0.05, ns = not significant; comparisons are with control unless indicated.
-
Figure 3—source data 1
Statistical data for Figure 3.
Sample size (n), mean, SEM, and pairwise statistical comparisons are presented for the data in Figure 3G,K, and L.
- https://doi.org/10.7554/eLife.13881.010
We next overexpressed UAS-Syx4A or UAS-Syx4B, in the presynaptic neuron (with elav-GAL4) or the postsynaptic muscle cell (with 24B-GAL4). None of these overexpressions resulted in any change in synaptic bouton number compared to control animals (Figure 3G). Thus, overexpression of Syx4 is not detrimental to synaptic growth.
We next attempted to rescue Syx4 mutant defects by expressing either isoform in the null mutant background with either pre- or postsynaptic-specific drivers at the NMJ. When expressed in the postsynaptic cell, both Syx4A and Syx4B were able to rescue the Syx473 decrease in bouton number compared to Syx473 alone (Figure 3E–G), restoring bouton number to control levels. In contrast, expressing either of these constructs presynaptically did not produce any rescue of the null mutant phenotype compared to Syx473 alone (Figure 3G). These findings indicate that Syx4 is required postsynaptically to regulate bouton number. Furthermore, either isoform of Syx4 is sufficient for Syx4 function.
Syx4 regulates active zone number
We also examined the organization of neurotransmitter release sites in Syx4 mutants by staining for Bruchpilot (Brp), a marker of the presynaptic AZ, and GluRIII, an obligate subunit of the postsynaptic glutamate receptor. By counting Brp+ puncta, we detected a significant decrease in the density of AZs per unit volume in Syx4 mutants compared to controls (Figure 3H,I,K). The AZ density defect was rescued by postsynaptic overexpression of Syx4 (Figure 3J,K). The amount of GluRIII fluorescence was unchanged in Syx4 mutants compared to controls, indicating normal amounts of GluRIII were present at the postsynaptic membrane (Figure 3H–J,L). In addition, the apposition of Brp and GluRIII was unaffected (Figure 3H–J). Thus, Syx4 mutants have a decrease in the density of AZs and a reduction in the total number of boutons, but no defects in the organization of individual release sites. The observation that postsynaptic Syx4 can regulate AZs in the presynaptic cell supports the hypothesis that Syx4 participates in retrograde signaling.
Genetic interactions between Synaptotagmin 4 and Syntaxin 4
Syx4 regulates the membrane localization of Syt4, and loss of either gene leads to a reduction in the number of synaptic boutons at the larval NMJ (Figure 3; Barber et al., 2009). To further investigate the relationship between Syt4 and Syx4 in synaptic development, we tested for genetic interactions between the null allele Syx473 and the null allele Syt4BA1 (Adolfsen et al., 2004). Single heterozygotes of Syx4 (Syx473/+) or Syt4 (Syt4BA1/+) had no bouton number phenotype compared to control animals (Figure 4A,B,F). Strikingly, double heterozygotes (Syx473/+ Syt4BA1/+) had a strong decrease in bouton number compared to control animals and compared to single Syx473/+ or Syt4BA1/+ heterozygotes (Figure 4C,F). This finding is consistent with Syx4 and Syt4 acting together to regulate bouton number and synaptic growth.
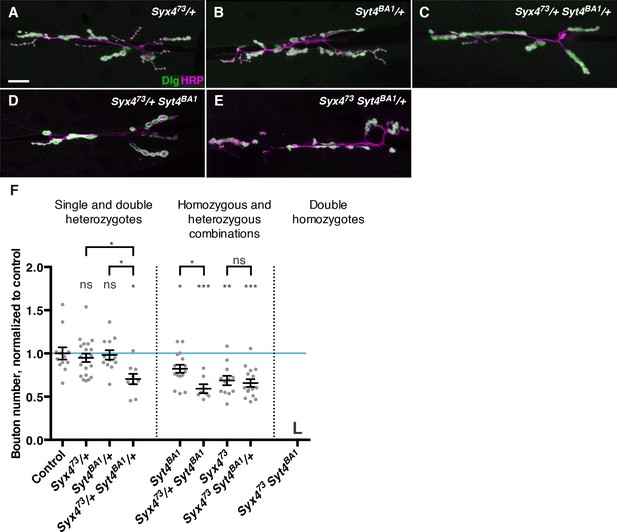
Genetic interactions between Syntaxin 4 and Synaptotagmin 4.
(A–E) Representative images of NMJs stained with antibodies to the postsynaptic marker Dlg (green) and the neuronal membrane marker HRP (magenta) to highlight the number of synaptic boutons; images are shown from Syx473/+ (A), Syt4BA1/+ (B), Syx473/+ Syt4BA1/+ (C), Syx473/+ Syt4BA1 (D), and Syx473 Syt4BA1/+ (E) animals. (F) Quantification of bouton number, normalized to controls. Blue line indicates the control mean. Data are presented as mean ± SEM. L = lethal. Scale bars = 20 μm (A–E). Statistical comparisons are fully described in Figure 4—source data 1, and are indicated here as ***p<0.001, **p<0.01, *p<0.05, ns = not significant; comparisons are with control unless indicated.
-
Figure 4—source data 1
Statistical data for Figure 4.
Sample size (n), mean, SEM, and pairwise statistical comparisons are presented for the data in Figure 4F.
- https://doi.org/10.7554/eLife.13881.012
To investigate the epistatic relationship between Syx4 and Syt4, we produced animals that are hemizygous for Syx473, which is on the X chromosome, and homozygous for Syt4BA1 (Syx473 Syt4BA1). These animals die during the 2nd larva instar, precluding analysis at the 3rd larval instar NMJ. As Syx473 hemizygotes die during the pupal stage, and Syt4BA1 homozygotes survive to adulthood, the early lethality observed in the double mutants reveals a synergistic interaction between the genes. This indicates that Syx4 and Syt4 act in parallel pathways, rather than a single epistatic pathway.
We also produced animals that were heterozygous for one gene and homo/hemizygous for the other. All of these animals survived to the 3rd larval instar, allowing us to assess bouton number. Removing one copy of Syt4BA1 in the Syx473 hemizygous background did not modify the Syx473 hemizygous phenotype (Figure 4E,F). In contrast, removing one copy of Syx473 in the Syt4BA1 homozygous background resulted in a further reduction in bouton number compared to Syt4BA1 homozygotes alone (Figure 4D,F). Taken together with the observation that Syx4 regulates membrane localization of Syt4, we conclude that Syx4 and Syt4 interact in one pathway, and also in parallel pathways, to regulate synapse development at the Drosophila NMJ.
Syntaxin 4 interacts with Neurexin and Neuroligin
Based on the following observations, we hypothesize that Syx4 regulates the release of retrograde signals to control synaptic development: 1) Syx4 localizes to the postsynaptic membrane; 2) Syx4 regulates the membrane localization of Syt4; 3) Syx4 regulates bouton number both with and independently of Syt4; 4) postsynaptic Syx4 regulates presynaptic AZ density; and 5) Syx4 proteins have a conserved function as plasma membrane t-SNAREs. To identify retrograde signals potentially regulated by Syx4, we tested for genetic interactions between Syx473 and components of characterized retrograde signaling pathways that affect bouton number at the NMJ. We failed to detect any dosage-dependent genetic interactions between Syx4 and components of the retrograde BMP signaling pathway that affects arbor size, neurotransmitter release, and synaptic plasticity (Figure 5—figure supplement 1; Aberle et al., 2002; Marqués et al., 2002; McCabe et al., 2003; Piccioli and Littleton, 2014; Rawson et al., 2003).
In contrast, we detected strong genetic interactions between Syx4 and the genes encoding the adhesion proteins Neurexin 1 (Nrx-1) and Neuroligin 1 (Nlg1) (Figure 5). Neurexins and Neuroligins form transsynaptic adhesion complexes, with a Neurexin typically the presynaptic partner and a Neuroligin the postsynaptic partner. At the Drosophila NMJ, Nrx-1 and the three characterized Nlgs (Nlg1-3) have been shown to play several roles in synaptic growth and organization, including regulation of bouton number, GluR subunit composition, and AZ size, spacing, and apposition (Banovic et al., 2010; Chen et al., 2010, 2012; Li et al., 2007b; Sun et al., 2011; Xing et al., 2014).
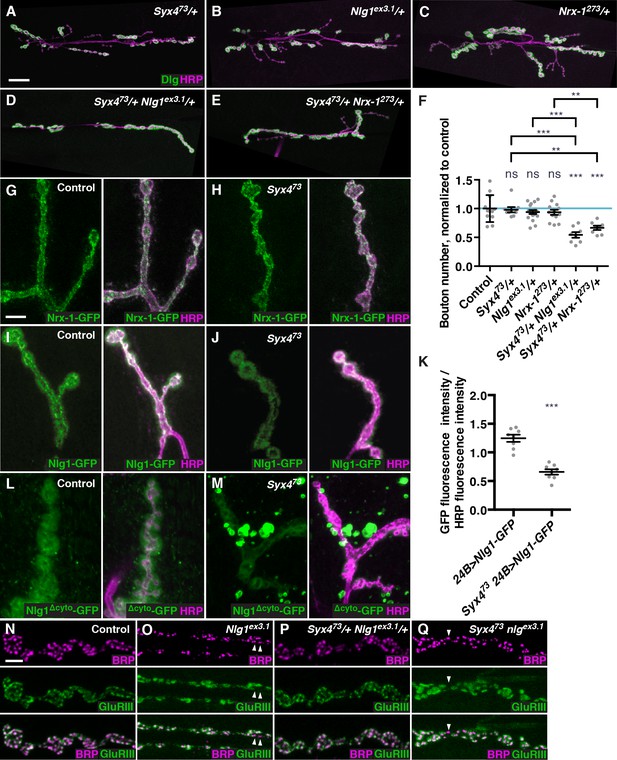
Syntaxin 4 interacts with Neuroligin 1 and regulates its membrane localization.
(A–E), Representative images of NMJs stained with antibodies to the postsynaptic density marker Dlg (green) and the neuronal membrane marker HRP (magenta) to highlight the number of synaptic boutons; images are shown from Syx473/+ (A), Nlg1ex3.1/+ (B), Nrx-1273/+ (C), Syx473/+ Nlg1ex3.1/+ (D), and Syx473/+ Nrx-1273/+ (E) animals. (F) Quantification of bouton number, normalized to controls. Blue line indicates the control mean. Data are presented as mean ± SEM. (G–H), Representative images of NMJs stained with antibodies against HRP (magenta) and expressing Nrx-1-GFP in a control (G) or Syx473 mutant (H) background. (I–J) Representative images of NMJs stained with antibodies against HRP (magenta) and expressing Nlg1-GFP in a control (I) or Syx473 mutant (J) background. (K) Quantification of GFP fluorescence per HRP fluorescence from animals expressing Nlg1-GFP in a control or Syx473 mutant background. Data are presented as mean ± SEM. (L–M) Representative images of NMJs stained with antibodies against HRP (magenta) and expressing Nlg1Δcyto-GFP in a control (L) or Syx473 mutant (M) background. (N–Q) Representative images of NMJs stained with antibodies against Brp (magenta) and GluRIII (green), from precise excision control (N), Nlg1ex3.1 (O), Syx473/+ Nlg1ex3.1/+ (P), and Syx473 Nlg1ex3.1 (Q) animals. Arrowheads indicate AZs lacking an apposed GluR field. Scale bars = 20 μm (A–E), 5 μm (I,J,L–Q). Statistical comparisons are fully described in Figure 5—source data 1, and are indicated here as ***p<0.001, **p<0.01, *p<0.05, ns = not significant; comparisons are with control unless indicated.
-
Figure 5—source data 1
Statistical data for Figure 5.
Sample size (n), mean, SEM, and pairwise statistical comparisons are presented for the data in Figure 5F and K.
- https://doi.org/10.7554/eLife.13881.014
We used the null alleles Nrx-1273 (Li et al., 2007b) and Nlg1ex3.1 (Banovic et al., 2010) to test for interactions with Syx4. Single heterozygotes of Nlg1 (Nlg1ex3.1/+), Nrx-1 (Nrx-1273/+), and Syx4 (Syx473/+) all had a normal number of boutons compared to control animals (Figure 5A–C,F). However, the double heterozygotes Syx473/+ Nlg1ex3.1/+ (Figure 5D) and Syx473/+ Nrx-1273/+ (Figure 5E) exhibited strong reductions in bouton number compared to controls and compared to each single heterozygote (Figure 5F). Thus, Syx4, Nrx-1, and Nlg1 cooperate to regulate bouton number at the NMJ.
We next tested whether the localization of Nrx-1 or Nlg1 was perturbed upon loss of Syx4. We expressed GFP-tagged forms of Nrx-1 and Nlg1 (Banovic et al., 2010) at the synapse and measured fluorescence intensity in control and Syx4 mutant backgrounds. When we expressed Nrx-1-GFP in the presynaptic cell using elav-GAL4, we did not detect any change in fluorescence intensity of GFP in Syx473 mutants compared to controls (Figure 5G,H). However, when we expressed Nlg1-GFP in the postsynaptic cell using 24B-GAL4, we detected a significant reduction in GFP signal at the synapse in the Syx473 null mutant background compared to controls (Figure 5I–K). This result indicates that Syx4 regulates the levels of Nlg1 at the postsynaptic membrane.
If the amount of Nlg1 at the synapse depends on Syx4, it is possible that the cytoplasmic domain of Nlg1 is involved in its delivery or retention. To test this hypothesis, we examined the localization of a tagged Nlg1 construct lacking the cytoplasmic domain (Nlg1Δcyto-GFP; Banovic et al., 2010). This construct was previously shown to localize to the NMJ, and to produce a dominant negative decrease in bouton growth (Owald et al., 2010). Like the full-length construct, Nlg1Δcyto-GFP localizes to the postsynaptic membrane when expressed in a control background (Figure 5L). Interestingly, this localization pattern is strikingly different when Nlg1Δcyto-GFP is expressed in a Syx473 mutant background. While some tagged protein is observed at the synapse, Nlg1Δcyto-GFP also appears in prominent bright clusters seen both near the NMJ (Figure 5M) and throughout the muscle (data not shown). This finding indicates a strong effect of Syx4 on Nlg1 localization, which is enhanced when the cytoplasmic domain of the protein is absent.
To further investigate the relationship between Syx4, Nrx-1, and Nlg1, we produced Syx473 Nlg1ex3.1 and Syx473 Nrx-1273 double mutant animals. Analysis of the double mutants revealed a strong reduction in bouton number compared to controls, and double mutants were not significantly different from single Nlg-1 or Nrx-1 mutants (Figure 5—figure supplement 2). Thus, complete loss of Syx4 does not enhance the bouton formation defects seen in Nlg1 or Nrx-1 homozygous mutants. This observation suggests that Nlg1 and Nrx-1 are downstream of Syx4 with respect to bouton number.
We next examined the organization of postsynaptic densities, which is perturbed in Nlg1 mutants (Banovic et al., 2010), but not in Syx473 (Figure 3). Consistent with previous studies (Banovic et al., 2010), we detected irregular and enlarged GluR fields in Nlg1 mutants, as well as defects in apposition between AZs and GluR fields, compared to controls (Figure 5N,O, arrowheads). We then tested whether loss of Syx4 could modify the Nlg1 AZ/GluR phenotypes. We first tested animals that were double heterozygotes for Syx473 and Nlg1ex3.1, and observed normal GluR field size and apposition (Figure 5P). Thus, the dosage-dependent genetic interactions we detected with respect to bouton number are not seen in the case of AZ/GluR organization. Double mutant animals (Syx473 Nlg1ex3.1) looked qualitatively similar to single Nlg1ex3.1 mutants (Figure 5Q, arrowheads), indicating that loss of Syx4 did not modify this aspect of the Nlg1 phenotype.
In summary, Syx4 and Nlg1 mutants have phenotypes that partially overlap (bouton number), but Nlg1 mutants have additional defects not seen with loss of Syx4 (organization of AZs/GluRs). Our genetic interaction data are consistent with Syx4 and Nlg1 cooperating to regulate bouton number, but not AZ/GluR organization. Taken together with the observation that loss of Syx4 leads to a partial reduction of Nlg1 at the membrane, one model is that minimal levels of Nlg1 are sufficient for AZ/GluR organization, but higher Syx4-dependent surface expression is required for regulating synaptic growth and bouton number.
Neuroligin 1 mobility is not affected by loss of Syntaxin 4
How does loss of Syx4 result in lower levels of Nlg1 at the postsynaptic membrane? One possibility is that less Nlg1 is delivered, and another is that Nlg1 is less stable or more mobile once it gets to the membrane. To investigate these possibilities, we measured the mobility of Nlg1 in vivo by tagging it with a photoconvertible fluorophore, Dendra2 (Adam et al., 2009; Gurskaya et al., 2006; Figure 6A). We added the Dendra2 tag in a juxta-membrane position, as previously described for Nlg1-GFP (Banovic et al., 2010). When expressed in the postsynaptic compartment using the muscle driver 24B-GAL4, Nlg1-Dendra2 localized to the synapse similarly to Nlg1-GFP (Figure 6B). We then used a 405 nm laser to convert approximately 50% of the fluorescent signal in a single bouton (Figure 6C,C′), and followed the fluorescence intensity of the green (non-photoconverted; NPC) and red (photoconverted; PC) signals over a 10 min period (Figure 6D and Video 1). We measured mobility by calculating the relative change in fluorescence (ΔF/F) in both channels from immediately after PC (t1 min) to 9 min after PC (t10 min), in the PC bouton (ROI1) or adjacent NPC boutons (ROI2 and ROI3), after correcting for photobleaching (Figure 6C′–E). If Nlg1-Dendra2 moved laterally in the membrane, we would expect to see a decrease in red/PC signal in ROI1 and an increase in red/PC signal in ROIs 2 and 3. If Nlg1-Dendra2 was internalized from the membrane, we would expect to see a decrease in red/PC signal in ROI1 without any increase in red/PC signal in ROIs 2 and 3. Interestingly, we measured extremely small ΔF/F values (< 0.02%) for the red/PC signal in all ROIs, reflecting very little change in fluorescence over the time course of the experiment (Figure 6D,E). We performed the same experiment by expressing Nlg1-Dendra2 in the Syx473 mutant background and observed a similar effect, with small ΔF/F values in the red/PC channel, and no significant change compared to control values (Figure 6E and Video 2). Thus, over the time course measured, Nlg1 is immobile at the synapse, and this is not changed by loss of Syx4. We also monitored the fluorescence of green/NPC molecules, and measured very small ΔF/F values (< 0.02%) in all ROIs for both controls and Syx473 mutants (Figure 6D,E). It is more difficult to interpret the movement of NPC molecules in this experiment; however, given the conclusion from the red/PC channel data that Nlg1 does not move laterally in the membrane or get internalized from the membrane over the time course of the experiment, the stable fluorescence of NPC molecules allows us to infer that very little new unconverted Nlg1 is delivered to the synapse. Thus, our analysis of photoconvertible Nlg1 reveals that Nlg1 is stable at the synapse over a short time course and this stability is not compromised by loss of Syx4. Based on these results, we hypothesize that the lower plasma membrane level of Nlg1 in Syx4 mutants is the result of changes in the delivery or removal of Nlg1 over a developmental time scale, or over a longer time course than our experimental paradigm.
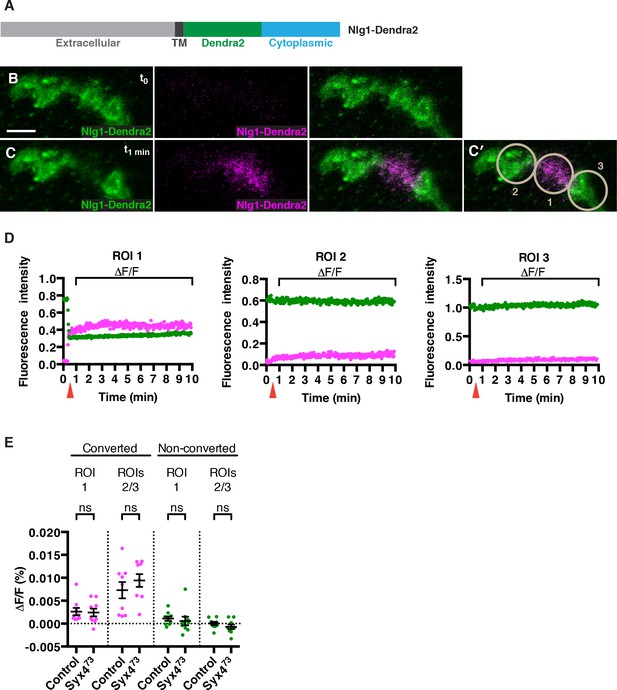
No change in mobility of Neuroligin 1 is observed in Syntaxin 4 mutants.
(A) Nlg1-Dendra2 construct. The Dendra2 tag was placed between the transmembrane domain and the cytoplasmic tail. (B–C) Representative image from a single animal expressing Nlg1-Dendra2 in the postsynaptic cell. One bouton (ROI1) was targeted with a 405 nm laser for photoconversion of the Dendra2 tag after 1 min. Non-photoconverted Nlg1-Dendra2 is shown in green and photoconverted Nlg1-Dendra2 is shown in magenta before (B) and immediately after (C) photoconversion. (C′) Regions of interest: ROI1, photoconverted region; ROIs 2 and 3, adjacent regions. (D) Fluorescent intensity over time for photoconverted and non-photoconverted molecules in all three ROIs. Red arrows indicate time of photoconversion. (E) Quantification of ΔF/F of both photoconverted and non-photoconverted molecules, in all three ROIs, in both the control and Syx473 mutant backgrounds. Data are presented as mean ± SEM. Scale bars = 5 μm. Statistical comparisons are fully described in Figure 6—source data 1; no significant differences found (ns = not significant).
-
Figure 6—source data 1
Statistical data for Figure 6.
Sample size (n), mean, SEM, and pairwise statistical comparisons are presented for the data in Figure 6E.
- https://doi.org/10.7554/eLife.13881.018
Photoconversion of Nlg1-Dendra2 in control animals.
Visualization of a synaptic arbor expressing postsynaptic Nlg1-Dendra2 at muscle 4 in a dissected third instar larva. One bouton is photoconverted after 20 sec, with about 50% of the green molecules converted to red (shown here as magenta). Over the next 9 min of imaging, very little movement of photoconverted molecules is observed. Scale bar = 2.5 μm.
Photoconversion of Nlg1-Dendra2 in Syx473 animals.
Visualization of a synaptic arbor expressing postsynaptic Nlg1-Dendra2 at muscle 4 in a dissected third instar larva mutant for Syx4. One bouton is photoconverted after 20 sec, with about 50% of the green molecules converted to red (shown here as magenta). Over the next 9 min of imaging, very little movement of photoconverted molecules is observed. Scale bar = 2.5 μm.
Syntaxin 4, Synaptotagmin 4, and Neuroligin 1 regulate acute structural plasticity at the NMJ
In addition to synaptic growth during development, the Drosophila NMJ displays acute structural plasticity where new boutons bud rapidly in response to strong neuronal stimulation (Ataman et al., 2008). Newly formed boutons, called ghost boutons (GBs), are readily identifiable as round structures containing neuronal membrane, but without any postsynaptic apparatus. The activity-dependent budding of GBs requires retrograde BMP signaling (Piccioli and Littleton, 2014), as well as retrograde signaling mediated by Syt4 (Korkut et al., 2013; Piccioli and Littleton, 2014).
We investigated whether Syx4 regulates acute structural plasticity in vivo using a high K+ stimulation protocol (Ataman et al., 2008; Piccioli and Littleton, 2014). As previously described, control animals exhibited robust GB budding in response to spaced incubations in high K+ over a 30 min period (Figure 7A,A′,K). However, budding was strongly suppressed in Syx4 null mutant animals compared to controls (Figure 7E,E′,K). Thus, like Syt4, Syx4 regulates rapid activity-induced synaptic growth. Furthermore, Syx4 and Syt4 interact genetically with respect to GB budding, as budding was strongly suppressed in Syx473/+ Syt4BA1/+ double heterozygotes compared to normal robust budding in either single heterozygote (Figure 7B,B′,C,C′,H,H′,K).
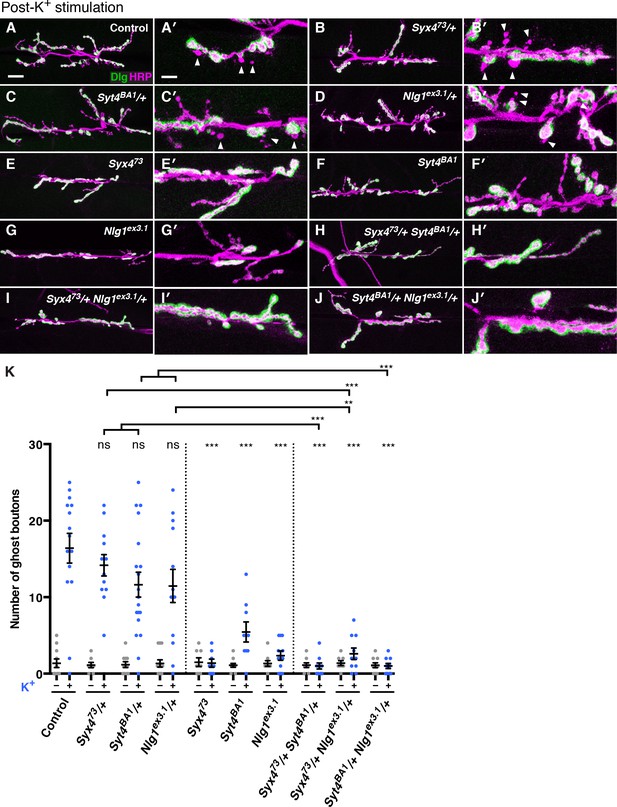
Syntaxin 4, Synaptotagmin 4, and Neuroligin 1 regulate acute structural plasticity at the NMJ.
(A–J) Representative images of NMJs stained with antibodies to HRP (magenta) and the postsynaptic marker Dlg (green) to highlight synaptic boutons. Ghost bouton budding was stimulated with spaced incubations in high K+. Ghost boutons are identified as round HRP+ structures lacking Dlg signal (arrowheads); images are shown from precise excision control (A), Syx473/+ (B), Syt4BA1/+ (C), Nlg1ex3.1/+ (D), Syx473 (E), Syt4BA1 (F), Nlg1ex3.1 (G), Syx473/+ Syt4BA1/+ (H), Syx473/+ Nlg1ex3.1/+ (I), and Syt4BA1/+ Nlg1ex3.1/+ (J) animals. (K) Quantification of ghost bouton number per NMJ from animals without (−) or with (+) high K+ stimulation. Data are presented as mean ± SEM. Scale bars = 20 μm (A–J), 6.7 μm (A′–J′). Statistical comparisons are fully described in Figure 7—source data 1, and are indicated here as ***p<0.001, **p<0.01, *p<0.05, ns = not significant; comparisons are with control unless indicated.
-
Figure 7—source data 1
Statistical data for Figure 5.
Sample size (n), mean, SEM, and pairwise statistical comparisons are presented for the data in Figure 7K.
- https://doi.org/10.7554/eLife.13881.022
We next tested whether GB budding is impaired in Nlg1 mutants, and whether Syx4 and Nlg1 interact in this context. Indeed, Nlg1ex3.1 mutants exhibited a strong suppression of GB budding compared to controls (Figure 7G,G′,K). Also, GB budding was strongly suppressed in Syx473/+ Nlg1ex3.1/+ double heterozygotes compared to single heterozygotes (Figure 7C,C′,D,D′,I,I′,K). These results indicate that Syx4 and Nlg1 interact to regulate activity-dependent formation of GBs.
Because Syx4 regulates the levels of both Syt4 and Nlg1 at the postsynaptic membrane, and all of these proteins are involved in regulating bouton number and rapid activity-dependent bouton formation, we investigated whether Syt4 and Nlg1 interact with each other. Indeed, we observed dosage-dependent genetic interactions between Syt4 and Nlg1 with respect to GB formation, as Syt4BA1/+ Nlg1ex3.1/+ double heterozygotes had a strong reduction in GB budding compared to single heterozygotes (Figure 7C,C′,D,D′,J,J′,K).
However, we were not able to detect an interaction between Syt4 and Nlg1 with respect to bouton number. The double heterozygotes Syt4BA1/+ Nlg1ex3.1/+ had a normal number of boutons compared to controls and compared to either single heterozygote (Figure 7—figure supplement 1), in contrast to the strong interactions we detected between Syx4/Syt4 (Figure 4) and Syx4/Nlg1 (Figure 5). We also expressed both Nlg1-GFP and Nlg1Δcyto-GFP in the Syt4BA1 null background and did not observe any change in Nlg1 localization compared to controls (Figure 7—figure supplement 1). Thus, our data are consistent with Syx4, Syt4, and Nlg1 cooperating to regulate acute synaptic structural plasticity. With respect to bouton number, the data support Syx4 interacting with Nlg1 and Syt4 in separate pathways. Taken together, Syx4 acts postsynaptically to regulate multiple parameters of synaptic biology by interacting with Nlg1 and Syt4 and regulating their membrane localization.
Discussion
To identify regulators of postsynaptic exocytosis, we conducted a screen for gene products regulating Syt4 plasma membrane accumulation, resulting in the identification of the plasma membrane t-SNARE Syx4. Analysis of a Syx4 null mutant indicates that Syx4 is essential for development of the Drosophila NMJ and regulates the membrane delivery of at least two proteins that are important for synaptic growth and plasticity: the postsynaptic Ca2+ sensor Syt4 and the transsynaptic adhesion protein Nlg1.
RNAi screen candidates suggest novel pathways that regulate retrograde signaling
Our screen identified 15 candidate gene products that altered the localization of Syt4-pH. In addition to Syx4, several other candidates motivate interesting hypotheses about regulatory pathways for postsynaptic exocytosis. MyoV is a Ca2+-sensitive unconventional myosin that regulates polarized traffic (Krauss et al., 2009; Li et al., 2007a) and the release of exosomes from motorneurons (Koles et al., 2012). Thus, MyoV could play a role linking Ca2+ influx to vesicle delivery or release at the synapse. Indeed, MyoV homologs have been implicated in regulated AMPA trafficking in mammalian dendrites (Correia et al., 2008; Wang et al., 2008). Two Rab regulators (Gdi and Rabex) suggest that key vesicle trafficking steps en route to the synapse are modulated by Rab activation states. Also, two cell adhesion molecules (Neuroglian and Contactin) indicate potential transsynaptic mechanisms regulating retrograde signaling. Neuroglian has been shown to be required for synaptic stability (Enneking et al., 2013) and it is possible that Syt4-mediated retrograde signaling plays some role in this process.
Syt4 has also been shown to be transferred transsynaptically from the presynaptic terminal to the postsynaptic terminal on exosomes (Korkut et al., 2013). Thus, our approach of expressing Syt4-pH postsynaptically may not reveal components for the biosynthetic synthesis and transport of presynaptic Syt4. Nevertheless, the requirement for Syt4 in the postsynaptic cell for retrograde signaling is clear (Barber et al., 2009; Korkut et al., 2013; Piccioli and Littleton, 2014; Yoshihara et al., 2005), and the results of our screen highlight regulators of Syt4 trafficking to and from the postsynaptic membrane where Syt4 vesicles fuse in an activity-dependent manner (Yoshihara et al., 2005). The observation that endogenously expressed Syt4-GFP (Syt4GFP-2M) shows a similar distribution to Syt4-pH supports the biological relevance of the screen data for identifying regulators of Syt4 trafficking in the postsynaptic cell.
Syntaxin 4 regulates the localization of Syt4-pH and interacts with Syt4 to regulate bouton number
Our Syx4 null allele phenocopies the Syx4-RNAi knockdown, reducing the delivery of Syt4-pH to the postsynaptic membrane. Consistent with this finding, loss of Syx4 produces similar phenotypes to loss of Syt4. Both null mutants exhibit a reduction in the total number of boutons at the NMJ, indicating a defect in synaptic growth. Moreover, genetic interaction experiments clearly indicate that Syx4 and Syt4 interact with respect to synaptic growth. A strong genetic interaction between Syx4 and Syt4 is also evident at the level of lethality, as double mutant animals are lethal at a much earlier stage than either single mutant alone. Thus, even though Syx4 affects the localization of Syt4, suggesting they act in the same pathway, the genetic interaction data do not support a simple epistatic relationship. The difference in phenotypic severity, with the Syx4 bouton number defect being significantly stronger than the Syt4 defect, also points to Syt4 not being absolutely required for Syx4 signaling. A similar phenomenon is observed presynaptically where the t-SNARE Syx1 is indispensible for synaptic vesicle fusion, while fusion is only reduced in the absence of the synaptic vesicle Ca2+ sensor Syt1. Taken together, we hypothesize that 1) Syx4 and Syt4 act together in a single pathway where Syx4 regulates the exocytosis of vesicles containing Syt4, and 2) Syx4 and Syt4 also act in divergent pathways, where Syt4 cooperates with other t-SNARES, and Syx4 mediates the exocytosis of vesicles in a Syt4-independent manner. This model allows for multiple possible postsynaptic SNARE complexes, regulating distinct release events. Dissecting the other components of these fusion machineries, and distinguishing activity-dependent from constitutive release events, will be important to build our understanding of how retrograde signaling is regulated.
Syt4 regulates membrane levels of Neuroligin
In addition to affecting the localization of Syt4, Syx4 mutants also exhibit a decrease in the amount of Nlg1 at the postsynaptic membrane. Nlg1 has several functions at the synapse, along with its presynaptic binding partner Nrx-1. Together they regulate bouton number as well as the size and spacing of active zones and glutamate receptors (Banovic et al., 2010; Li et al., 2007b; Owald et al., 2010), though some aspects of Nlg1 signaling appear to be independent of Nrx-1 (Banovic et al., 2010). Mutations in Nrx and Nlg family genes are also linked to ASD, highlighting the importance of Nrx-Nlg signaling in neuronal development (Bottos et al., 2011; Südhof, 2008). Consistent with a reduction of Nlg1 levels at the synapse, we observed strong genetic interactions between Syx4, Nlg1 and Nrx-1 with respect to bouton number. However, the prominent AZ/GluR defects seen in Nlg1 and Nrx-1 mutants were not observed in Syx4 mutants, and heterozygous combinations did not produce these defects. It is likely that Syx4 mutants exhibit a partial loss of function of Nlg1, and that bouton number is sensitive to this loss while AZ/GluR organization can be maintained with low levels of Nlg1.
A dramatic change in distribution of Nlg1Δcyto is observed in the Syx4 mutant background, providing further evidence that Syx4 regulates the localization of Nlg1. The redistribution of Nlg1Δcyto to large accumulations is striking compared to full-length Nlg1, which is simply reduced at the synapse in the Syx4 mutant background. This observation points to complex Syx4-dependent regulation of Nlg1 localization. One model is that trafficking of Nlg1 involves both a Syx4-dependent pathway and a second pathway that depends on an interaction with the Nlg1 C-terminus, which includes a PDZ-domain-binding motif. In this scenario, a severe Nlg1 trafficking defect is revealed only when both pathways are compromised. A second possibility is that in the absence of Syx4, a portion of the Nlg1 content in the cell is degraded, but that this degradation step depends on the presence of the Nlg1 cytoplasmic tail, leading to the observed aggregation of Nlg1Δcyto in Syx4 mutants.
Our analysis of Nlg1 trafficking in live animals reveals that Nlg1 is strikingly stable, in both control and Syx4 mutant backgrounds. Our motivation in performing these experiments was to test possible mechanisms underlying the decrease in Nlg1 levels in Syx4 mutants. It is possible that some Nlg1 mobility would be observed over a longer time course. Mammalian Nlg has been shown to undergo significant turnover at postsynaptic sites under LTP conditions in neuronal cell culture (Schapitz et al., 2010). Also, synaptic activity has been shown to induce cleavage of Nlg and the subsequent destabilization of the Nrx-Nlg complex (Peixoto et al., 2012). Thus, it remains a possibility that Nlg1 would be mobilized in response to activity in our preparation; however, we have not observed any increased mobility in response to high K+ incubations in preliminary tests (data not shown). Our data are most consistent with Syx4 regulating Nlg1 over a developmental time course. A detailed examination of the relationship between Syx4 and Nlg1 dynamics will be crucial to understand how Syx4 contributes to this important pathway in synaptic development.
Syx4, Syt4, and Nlg1 regulate synaptic plasticity
We observed a strong suppression of acute structural plasticity in null mutants of Syx4, Syt4 and Nlg1. Double heterozygous combinations also indicated strong genetic interactions between all three of these genes with respect to plasticity. GB budding is regulated by both acute and developmental signaling. Because Syt4 postsynaptic vesicles fuse in an activity-dependent manner (Yoshihara et al., 2005), it is possible that Syt4-dependent signaling releases an acute instructive cue for GB budding. Thus, one attractive model is that Nlg1 is delivered to the membrane in response to stimulation, depending on the Ca2+ sensitivity of Syt4 and the presence of the t-SNARE Syx4 at the membrane. It is also possible that Syx4-Syt4-Nlg1 signaling is required throughout development to potentiate the synapse to respond to strong neuronal stimulation. In conclusion, Syx4, Syt4, and Nlg1 interact to regulate several aspects of synaptic biology. Our data support multiple overlapping signaling pathways regulated by these proteins, reflecting a complex modulation of retrograde signaling to control synaptic growth and plasticity at the Drosophila NMJ.
Materials and methods
Drosophila stocks
Request a detailed protocolAll Drosophila strains were cultured on standard media at 25°C. The following stocks were used: 24B-GAL4 (BDSC 1767; Brand and Perrimon, 1993); elav-GAL4[2] (BDSC 8765; Luo et al., 1994); Df(1)ED6630 (BDSC 8948; Ryder et al., 2007); witA12, witB11 (Marqués et al., 2002); UAS-Syt4-pHluorin (Yoshihara et al., 2005); Syt4BA1 (Adolfsen et al., 2004); gbb1(Wharton et al., 1999); Nlg1ex3.1, UAS-Nlg1-GFP, UAS-Nlg1Δcyto-GFP (Banovic et al., 2010); Nrx-1273 (Li et al., 2007b); UAS-Syx4-RNAi (TRiP JF01714; Perkins et al., 2015), UAS-Syx4-RNAi (VDRC 32413; Dietzl et al., 2007).
Transgenics
Request a detailed protocolFull-length Syntaxin 4 was obtained from the Drosophila Genomics Resource Center (DGRC RE02884; Stapleton et al., 2002). Three point mutations in the cDNA were corrected with a Quickchange Lightning Multi site-directed mutagenesis kit (Agilent Technologies, Santa Clara, CA) (pos 71: G to A; pos 496: A to C; pos 693: T to A). The sequence listed in Flybase, and several other ESTs covering parts of the Syx4 sequence, agree that these changes reflect the correct sequence. UAS-Syx4 was produced by PCR-amplifying Syx4 using ExTaq (ClonTech Laboratories, Mountain View, CA), and adding a 5’NdeI site and a 3’XbaI site. The PCR product was subsequently digested and subcloned into pValum (Ni et al., 2008). The construct was injected into a third chromosome docking strain (y1 w67c23;P{CaryP}attP2) by Best Gene Inc (Chino Hills, CA). UAS-RFP-Syx4 was produced by PCR-amplifying Syx4 and subcloning into pENTR/D-TOPO (Thermo Fisher Scientific, Waltham, MA). Syx4 was then moved into the destination vector pPRG using the Gateway system (Thermo Fisher Scientific; Gateway vectors developed by T. Murphy, The Carnegie Institution of Washington, Baltimore, MD). The construct was injected into w1118, along with a P-element helper plasmid, for random insertion by Best Gene Inc. Nlg1-Dendra2 was synthesized and subcloned into PBID-UASc (Wang et al., 2012) by Epoch Life Sciences (Sugar Land, TX). The Dendra2 tag (Adam et al., 2009; Gurskaya et al., 2006) was inserted between A865 and L866, about 11 aa downstream of the TM domain. These 11 aa were then repeated at the end of Dendra2, as previously described (Banovic et al., 2010). The construct was injected into a second chromosome docking strain (y1 w67c23; P{CaryP}attP40) by Best Gene Inc.
Syx4 antibody production
Request a detailed protocolFull length Syx4A was subcloned into pGEX-2T (GE Healthcare, UK) and GST-Syx4A protein was expressed and purified from OneShot BL21 cells (Thermo Fisher Scientific) as previously described (Frangioni and Neel, 1993). Rabbit immunosera were produced by SDIX (Newark, DE).
Syx4 mutagenesis
Request a detailed protocolThe P element line P{EPgy2}Syx4[EY0005] (BDSC 14995; Bellen et al., 2011), carrying an insertion in the 5’-UTR of the Syx4 locus, was crossed to Tft/CyO, Δ2–3 (BDSC 8201) to mobilize the insertion. Single mosaic male progeny were then crossed to 2–4 females from the 1st chromosome balancer stock Df(1)ED6630/FM7i (BDSC 8948; Ryder et al., 2007). In the next generation, single white-eyed balanced females were crossed to 2–3 FM7i males. Approximately 150 lines were tested by PCR to detect deletions of the Syx4 locus. Three Syx4 alleles were identified and sequenced to determine the deletion breakpoints. Syx439: X:2743312..2743832 deleted and >1 kb of P-element sequence inserted; Syx448: X:2742469..2743832 deleted and ~690 bp of P-element sequence inserted; Syx473: X:2738999..[2750535–2752545] deleted. A precise excision was also identified and was used as a control line in all experiments unless otherwise indicated.
Construction of the Syt4GFP-2M transgenic line
Request a detailed protocolHomology-directed repair (HDR) following CRISPR/Cas9-induced double strand break was used to generate C-terminally tagged Syt4 knock-in lines. To construct the HDR donor plasmid pDsRed-Attp-syt4-DNA-eGFP, 1.1 kb of genomic DNA downstream of the Syt4 stop codon was inserted at the BglII site of pDsRed-Attp (Addgene #51019, gift from Melissa Harrison, Kate O’Connor-Giles, & Jill Wildonger), producing the plasmid pDsRed-Attp-Syt4-p2. Then 1.3 kb of genomic DNA upstream of the Syt4 stop codon was fused with eGFP coding sequence and inserted at the NheI site of pDsRed-Attp-syt4-p2, producing pDsRed-Attp-Syt4-DNA-eGFP-pre. Finally, the gRNA binding sites in this plasmid were mutated, resulting the final donor plasmid. All cloning steps were performed using Gibson Assembly (New England Biolabs, Ipswich, MA, #E5510).
Two gRNA sequences were designed according to Gokcezade et al., 2014 and inserted into pCFD4-U6:1_U6:3tandemgRNAs (Addgene #49411; Port et al., 2014). The plasmid was injected into y1 w67c23; P{CaryP}attP40 embryos by Best Gene Inc. to generate the Syt4-gRNA stock.
To generate the GFP-tagged Syt4 flies, yw; nos-Cas9 flies (Kondo and Ueda, 2013) were crossed with Syt4-gRNA flies, and the embryos from the cross were injected with the donor plasmid. Successful transformants were screened for the presence of 3XP3-DsRed in the flies. The nos-Cas9 and Syt4-gRNA expression cassettes were crossed out in the next generation. In the final stock, PCR and sequencing were performed to confirm the insertion and verify that no mutation was present. Several independent lines were generated and validated. One of the homozygous viable and fertile lines, Syt4GFP-2M, was used for all experiments. Homozygous animals were stained with antibodies against GFP to visualize Syt4GFP-2M protein.
Immunostaining
Request a detailed protocolLarvae were reared at 25°C and dissected at the third wandering instar stage. Larvae were dissected in HL3.1 solution (in mM, 70 NaCl, 5 KCl, 10 NaHCO3, 4 MgCl2, 5 trehalose, 115 sucrose, 5 HEPES, pH 7.2) and fixed in 4% paraformaldehyde or as otherwise indicated. Following washes in PBT (PBS containing 0.3% Triton X-100), larvae were blocked for one hour in PBT containing 2% normal goal serum, incubated overnight with primary antibody at 4°C, washed, incubated with secondary antibodies for 2 hr at room temperature, washed, and mounted in Vectashield (Vector Laboratories, Burlingame, CA) for imaging. For Syx4 stainings, Syx4 antibody was preabsorbed on Syx4 null mutant tissue to reduce background staining. Antibodies were as follows: mouse anti-Dlg, 1:1000 (DSHB 4F3; Parnas et al., 2001); anti-Brp, 1:500 (DSHB nc82; Wagh et al., 2006); anti-GluRIII, 1:500 (Marrus et al., 2004); anti-GluRIII-488, 1:500 (Blunk et al., 2014; Marrus et al., 2004); anti-Syx4, 1:500; rabbit anti-Lva, 1:500 (Sisson et al., 2000); DyLight 649 conjugated anti-horseradish peroxidase, 1:1000 (Jackson ImmunoResearch, West Grove, PA); Alexa Fluor 488 goat anti-mouse, Alexa Fluor 488 goat anti-rabbit, and Alexa Fluor 546 goat anti-mouse, 1:400 (Thermo Fisher Scientific). Images were acquired with a 40 × 1.3 NA oil-immersion objective (Carl Zeiss, Germany).
RNAi screen
Request a detailed protocolThe recombinant stock UAS-Syt4-pHluorin, 24B-GAL4 was produced and used for the screen. Females from this line were crossed to UAS-RNAi males, and 3 progeny were dissected per RNAi line tested. Larvae were dissected in HL3.1 buffer, fixed in 4% paraformaldehyde, washed in PBT, incubated overnight at 4°C with antibodies against HRP, washed in PBT, and mounted in Vectashield (Vector Laboratories). Syt4-pH distribution at the NMJ was analyzed in hemisegment A3 at muscle 4. A control cross was included in every batch, where UAS-Syt4-pHluorin, 24B-GAL4 was crossed to a UAS line that had no effect on Syt4-pH distribution (UAS-FLP; BDSC 4540; Duffy et al., 1998). A list of all RNAi stocks screened is found in Supplementary file 1.
High K+ stimulation of larval NMJs
Request a detailed protocolThe acute structural plasticity assay was performed as previously described (Piccioli and Littleton, 2014). Wandering third instar larvae were dissected in HL3 solution (in mM, 70 NaCl, 5 KCl, 0.2 CaCl2, 20 MgCl2, 10 NaHCO3, 5 trehalose, 115 sucrose, and 5 HEPES, pH7.2). Dissecting pins were then moved inward to 60% of the original size for each larva. Relaxed fillets were subjected to three 2 min incubations in high K+ solution (in mM, 40 NaCl, 90 KCl, 1.5 CaCl2, 20 MgCl2, 10 NaHCO3, 5 trehalose, 5 sucrose, and 5 HEPES, pH 7.2), spaced by 10 min in HL3. After the third high K+ incubation, larvae were returned to HL3 solution for 2 min and then stretched to their original size and fixed.
Quantification of confocal images
Request a detailed protocolAnalyses were conducted using Volocity (version 6.3) or FIJI / ImageJ (version 2.0.0-rc-32/1.49v; Schindelin et al., 2012). Ghost boutons were identified by the presence of a presynaptic bouton (HRP–labeled) that lacked Dlg staining in fixed preparations. Counting of boutons and GBs was conducted at hemisegment A3 at muscle 6/7, and n refers to the number of NMJs analyzed, with no more than two NMJs analyzed per animal, and with animals derived from at least three independent experiments. Measurements of AZ density and GluR intensity were conducted on 12 1b boutons per animal, using 1 terminal bouton and 5 adjacent non-terminal boutons, on two different branches; n refers to the number of animals analyzed. AZ density was quantified manually by counting Brp–labeled puncta and dividing by the volume of HRP. GluR intensity was quantified by measuring the fluorescence intensity of GluRIII signal within an ROI defined by the HRP signal, and the average intensity within the ROI was divided by the average HRP intensity. All analyses were performed blind to genotype.
Photoconversion of Nlg1-Dendra2
Request a detailed protocolWandering third instar larvae expressing postsynaptic Nlg1-Dendra2 were dissected in HL3.1 saline at room temperature. Images were acquired with a Carl Zeiss LSM 700 with a 40× 0.8 NA water-immersion objective using Zen software (Zeiss). A single confocal plane of a muscle 4 NMJ in hemisegment A3 was continuously imaged for 10 min in the red and green channels. After the first 20 s, a single bouton was targeted with pulses of the 405 nm laser at 100% power until ~50% of the green signal was converted. Images were stabilized using the Image stabilizer plug-in from FIJI / Image J (K. Li, The image stabilizer plugin for ImageJ, http://www.cs.cmu.edu/~kangli/code/Image_Stabilizer.html, February, 2008). Fluorescence intensity was measured in the photoconverted ROI, two adjacent ROIs, and a 4th distant ROI (photobleaching ROI). Each intensity measurement was divided by the photobleaching ROI measurement at that time point to correct for photobleaching. ΔF/F was calculated on corrected measurements as (FT10min-FT1min)/FT1min*100.
Statistical analyses
Request a detailed protocolStatistical analyses were conducted using GraphPad Prism. Statistical significance in two-way comparisons was determined by a Student’s t-test, while ANOVA analysis was used when comparing more than two datasets. The P values associated with ANOVA tests were adjusted P values obtained from a Tukey’s post hoc test. In all figures, the data is presented as mean ± SEM; *** p<0.001, ** p<0.01, * p<0.05, n.s. not significant. Statistical comparisons are with control unless noted. Sample size (n), mean, SEM, and pairwise statistical comparisons are presented in figure supplements.
RT-PCR analysis of Syntaxin 4
Request a detailed protocolRNA was extracted from 5 larvae per sample using an RNease Mini kit (Qiagen Sciences, Germantown, MD) and treated with DNAse I (Qiagen). RT-PCR was carried out using a SuperScript One-Step RT-PCR System with Platinum Taq (Thermo Fisher Scientific). Forward primers were designed to bind to unique 5′-UTR sequences of the Syx4A and Syx4B transcripts.
References
-
Synaptotagmins are trafficked to distinct subcellular domains including the postsynaptic compartmentThe Journal of Cell Biology 166:249–260.https://doi.org/10.1083/jcb.200312054
-
A matter of balance: Role of neurexin and neuroligin at the synapseNeurochemical Research 38:1174–1189.https://doi.org/10.1007/s11064-013-1029-9
-
Postsynaptic regulation of synaptic plasticity by synaptotagmin 4 requires both C2 domainsThe Journal of Cell Biology 187:295–310.https://doi.org/10.1083/jcb.200903098
-
Postsynaptic actin regulates active zone spacing and glutamate receptor apposition at the Drosophila neuromuscular junctionMolecular and Cellular Neurosciences 61:241–254.https://doi.org/10.1016/j.mcn.2014.07.005
-
Neurexins and neuroligins: Synapses look out of the nervous systemCellular and Molecular Life Sciences : CMLS 68:2655–2666.https://doi.org/10.1007/s00018-011-0664-z
-
Targeted gene expression as a means of altering cell fates and generating dominant phenotypesDevelopment 118:401–415.
-
The role of AMPA receptors in postsynaptic mechanisms of synaptic plasticityFrontiers in Cellular Neuroscience 8:.https://doi.org/10.3389/fncel.2014.00401
-
Identifying loci required for follicular patterning using directed mosaicsDevelopment 125:2263–2271.
-
Solubilization and purification of enzymatically active glutathione S-transferase (pGEX) fusion proteinsAnalytical Biochemistry 210:179–187.https://doi.org/10.1006/abio.1993.1170
-
Postsynaptic membrane addition depends on the Discs-Large-interacting t-SNARE GtaxinThe Journal of Neuroscience 27:1033–1044.https://doi.org/10.1523/JNEUROSCI.3160-06.2007
-
Transsynaptic modulation of the synaptic vesicle cycle by cell-adhesion moleculesJournal of Neuroscience Research 86:223–232.https://doi.org/10.1002/jnr.21484
-
The role of nitric oxide in pre-synaptic plasticity and homeostasisFrontiers in Cellular Neuroscience 7:.https://doi.org/10.3389/fncel.2013.00190
-
Snares--engines for membrane fusionNature Reviews. Molecular Cell Biology 7:631–643.https://doi.org/10.1038/nrm2002
-
Unconventional GABA release: Mechanisms and functionCurrent Opinion in Neurobiology 19:305–310.https://doi.org/10.1016/j.conb.2009.03.006
-
Mechanism of evenness interrupted (evi)-exosome release at synaptic boutonsThe Journal of Biological Chemistry 287:16820–16834.https://doi.org/10.1074/jbc.M112.342667
-
Myosin-V regulates oskar mRNA localization in the Drosophila oocyteCurrent Biology 19:1058–1063.https://doi.org/10.1016/j.cub.2009.04.062
-
Myosin V, rab11, and drip11 direct apical secretion and cellular morphogenesis in developing drosophila photoreceptorsThe Journal of Cell Biology 177:659–669.https://doi.org/10.1083/jcb.200610157
-
Quantitative proteomics and protein network analysis of hippocampal synapses of camkiialpha mutant miceJournal of Proteome Research 6:3127–3133.https://doi.org/10.1021/pr070086w
-
A genomic analysis of membrane trafficking and neurotransmitter release in drosophilaThe Journal of Cell Biology pp. F77–F82.https://doi.org/10.1083/jcb.150.2.F77
-
Differential localization of glutamate receptor subunits at the drosophila neuromuscular junctionThe Journal of Neuroscience 24:1406–1415.https://doi.org/10.1523/JNEUROSCI.1575-03.2004
-
Drosophila neuroligin 1 regulates synaptic growth and function in response to activity and phosphoinositide-3-kinaseMolecular and Cellular Neurosciences 51:89–100.https://doi.org/10.1016/j.mcn.2012.08.010
-
Endocannabinoid-mediated retrograde modulation of synaptic transmissionCurrent Opinion in Neurobiology 29:1–8.https://doi.org/10.1016/j.conb.2014.03.017
-
A Syd-1 homologue regulates pre- and postsynaptic maturation in DrosophilaThe Journal of Cell Biology 188:565–579.https://doi.org/10.1083/jcb.200908055
-
A postsynaptic spectrin scaffold defines active zone size, spacing, and efficacy at the Drosophila neuromuscular junctionThe Journal of Cell Biology 175:491–503.https://doi.org/10.1083/jcb.200607036
-
Growth factors in synaptic functionFrontiers in Synaptic Neuroscience 5:.https://doi.org/10.3389/fnsyn.2013.00006
-
Optimized crispr/cas tools for efficient germline and somatic genome engineering in DrosophilaProceedings of the National Academy of Sciences of the United States of America 111:E2967.https://doi.org/10.1073/pnas.1405500111
-
Retrograde signalling at the synapse: A role for Wnt proteinsBiochemical Society Transactions 33:1295–1298.https://doi.org/10.1042/BST20051295
-
Neuroligin 1 is dynamically exchanged at postsynaptic sitesThe Journal of Neuroscience 30:12733–12744.https://doi.org/10.1523/JNEUROSCI.0896-10.2010
-
Fiji: An open-source platform for biological-image analysisNature Methods 9:676–682.https://doi.org/10.1038/nmeth.2019
-
Lava lamp, a novel peripheral golgi protein, is required for Drosophila melanogaster cellularizationThe Journal of Cell Biology 151:905–918.https://doi.org/10.1083/jcb.151.4.905
-
Wnts: Up-and-coming at the synapseTrends in Neurosciences 30:268–275.https://doi.org/10.1016/j.tins.2007.04.003
-
Neuroligin 2 is required for synapse development and function at the drosophila neuromuscular junctionThe Journal of Neuroscience 31:687–699.https://doi.org/10.1523/JNEUROSCI.3854-10.2011
-
Genetic analysis of the bone morphogenetic protein-related gene, gbb, identifies multiple requirements during Drosophila developmentGenetics 152:629–640.
-
Drosophila neuroligin3 regulates neuromuscular junction development and synaptic differentiationThe Journal of Biological Chemistry 289:31867–31877.https://doi.org/10.1074/jbc.M114.574897
-
Functions and mechanisms of retrograde neurotrophin signallingNature Reviews. Neuroscience 6:615–625.https://doi.org/10.1038/nrn1727
Article and author information
Author details
Funding
National Institutes of Health (NS40296)
- J Troy Littleton
The funders had no role in study design, data collection and interpretation, or the decision to submit the work for publication.
Acknowledgements
This work was supported by NIH grant NS40296. We thank S Sigrist, H Aberle, M Bhat, O Papoulas, and A Vasin for sharing reagents. We thank the Bloomington Drosophila Stock Center (Indiana University, Bloomington, IN; NIH P40OD018537), the Drosophila Genome Resource Center (Indiana University, Bloomington, IN; NIH 2P40OD010949-10A1), the TRiP at Harvard Medical School (Boston, MA; NIH/NIGMS R01-GM084947), the Developmental Studies Hybridoma Bank (University of Iowa, Iowa City, IA), and the Vienna Drosophila Resource Center (Austria), for providing materials used in this study.
Copyright
© 2016, Harris et al.
This article is distributed under the terms of the Creative Commons Attribution License, which permits unrestricted use and redistribution provided that the original author and source are credited.
Metrics
-
- 3,126
- views
-
- 558
- downloads
-
- 45
- citations
Views, downloads and citations are aggregated across all versions of this paper published by eLife.
Citations by DOI
-
- 45
- citations for umbrella DOI https://doi.org/10.7554/eLife.13881