Target DNA bending by the Mu transpososome promotes careful transposition and prevents its reversal
Figures
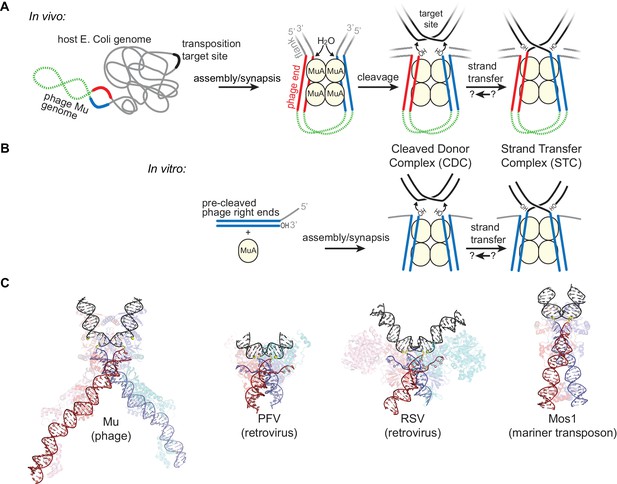
Transposition by Bacteriophage Mu and Available Structures of DDE family members.
(A) Diagram of replicative transposition. The transposable element (here, the phage genome) is in green with transposase binding sites in red and blue. Transposase subunits (here, MuA, in light yellow circles) synapse element ends and catalyze their nicking and subsequent joining to target DNA (black). In vivo, the flanking DNA (grey) is double stranded and may represent the entire host chromosome, which may also provide the target site. (B) The in vitro Mu transposition system used here utilizes short linear fragments for both the target DNA and phage ends, with 3 nt of single stranded flanking DNA. (C) Strand transfer complex structures. DNA is colored as in (A), with protein components partially transparent and colored according to their bound DNA. Generated from PDB IDs 4FCY (Mu), 3OS0 (PFV), 5HOO (Mos1), and 5EJK (RSV).
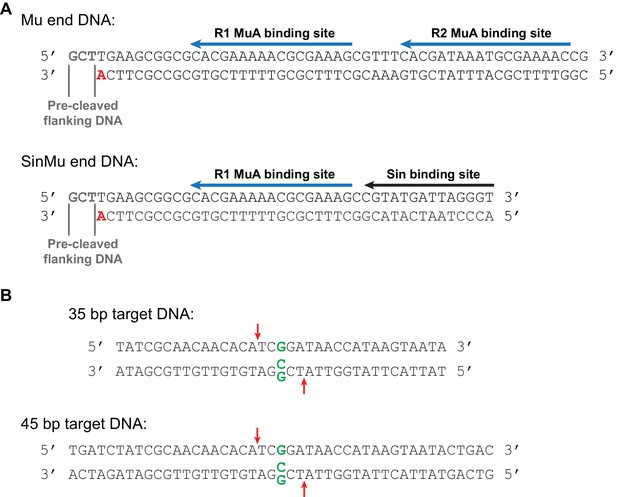
Mu end and target DNA fragments.
(A) The Mu end DNA fragments used for transposition reactions. The bottom strand becomes joined to the target DNA during strand transfer. The bold red 3’ adenine provides the 3’ hydroxyl group that is the nucleophile for this reaction. In fluorescence anisotropy and some FRET experiments (where indicated in the text), this adenine lacked a 3’ hydroxyl group so as to suppress strand transfer. Blue arrows mark the binding sites for MuA; the R2 binding site is replaced with a binding site for the Sin recombinase in the SinMu system. The Mu end DNA is identical to that used to generate the Mu STC crystal structure (Montaño et al., 2012). (B) Linear target DNA substrates. The G:G mismatch, when used, was placed in the center of the sequence by replacing a C in the bottom strand with a G, indicated in green. Red arrows mark the position of the predominant strand transfer product when the mismatch is present. The 45 bp target DNA was used in the experiments in Figure 5 to discourage melting of the strand transfer products during heating, and is identical in sequence to the 35 bp target used elsewhere except for 5 bp added at each end. Target DNAs were labeled at the 5’ end of the top strand with 32P for all radiographic experiments, and with the Atto565 fluorophore for fluorescence anisotropy and FRET. FRET substrates included an additional Atto647N fluorophore at the 5’ end of the bottom strand. The 35 bp target (with a mismatched base pair) is also identical to that used to generate the Mu STC crystal structure (Montaño et al., 2012).
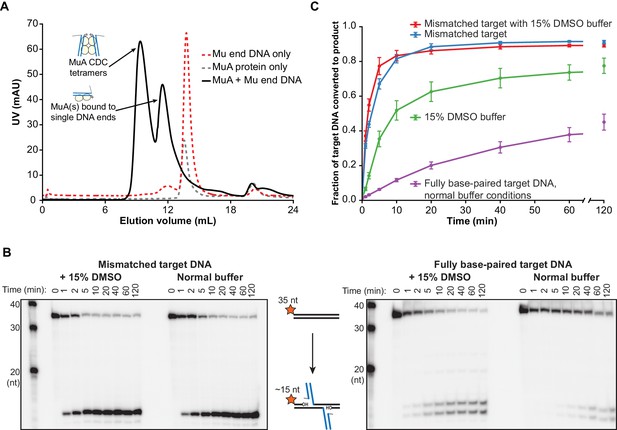
Target DNA bending during Mu transposition affects strand transfer kinetics.
(A) Purification of transpososome tetramers constructed in vitro by gel filtration. Full transpososomes (black line) are separable from lower molecular weight complexes Also shown are MuA protein and phage end DNAs alone (dashed lines). The contents of only the tetramer peak were used in our assays here. (B) Strand transfer kinetics visualized by denaturing gel electrophoresis and 5’-32P-labeled target DNA. Where indicated, the 35 bp target DNA sequence includes a G:G base-pairing mismatch and/or the reaction buffer was supplemented with 15% DMSO. Strand transfer results in cleavage of the labeled strand. (C) Quantification of the strand transfer kinetics experiments described in (B). The vertical axis represents the fraction of total lane signal present in product band(s). The X-axis is broken between 60 and 120 min to enhance the readability of early timepoints. Error bars represent mean ± standard error of the mean (SEM), n = 4 independent CDC preparations, one of which is shown in (B).
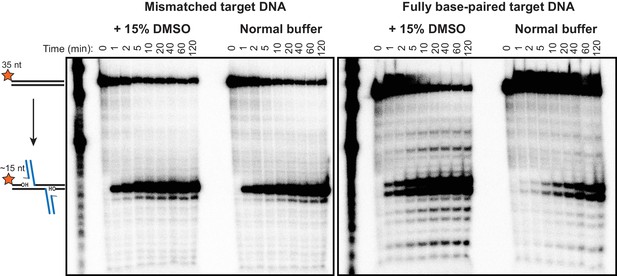
Minor strand transfer products viewed at high contrast.
Pictured are the same radiographic gel images given in Figure 2B, but with the cropping and contrast modified to highlight minor strand transfer products. Note that the mismatch drives strand transfer to occur predominantly at a single position, which is centered around the mismatch, at the expense of the numerous minor products that result from fully base-paired DNA.
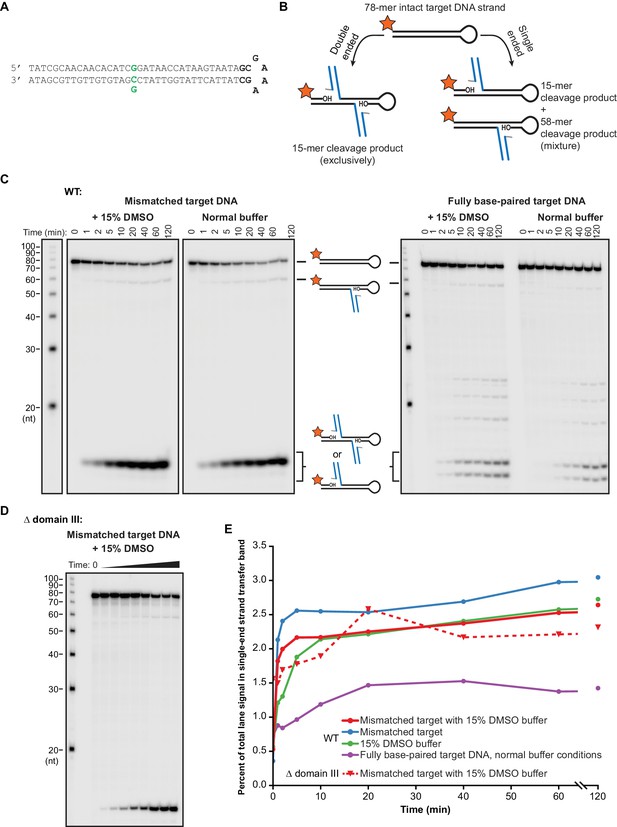
Modifying target DNA flexibility or removing domain III do not alter the concerted nature of MuA-catalyzed strand transfer.
(A) The sequence of the hairpin target DNA fragment used here to detect single ended strand transfer events. This differs from the target DNA used throughout this work (Figure 1—figure supplement 1) only in the addition of the hairpin turn sequence shown in bold. As in that Figure, green indicates the nucleotide position that can be changed to generate a base-pairing mismatch. (B) Schematic for the experiment to detect single ended events. Single-ended strand transfer can cleave the 5’-labeled hairpin target DNA to create a novel 58 nt product. (C) The same experimental protocol as described for Figure 2 was used with the hairpin target DNA to monitor for single-ended events. A faint 58 nt band for these events appears across all conditions. (D) As in (C), except Δ domain III CDCs (as in the experiments in Figure 4) were used. (E) Quantification of the images in (C) and (D). The vertical axis represents the percent of the total radioactivity contained in all bands that exists in the 58 nt single ended product band. Across all conditions, single ended events accumulate rapidly to a level ≤3% of total target DNA and remain nearly constant after 10–20 min of reaction time. Because levels remain constant, we interpret this to be the result of a tiny population of defective MuA protein that is capable of participating in assembly but not strand transfer.
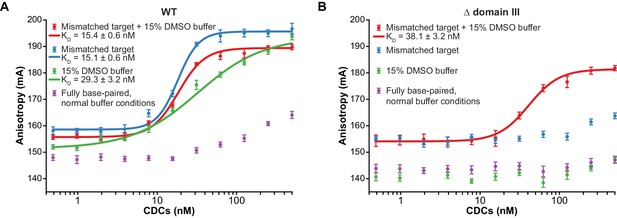
Enhanced flexibility triggers tight target DNA binding.
Fluorescence anisotropy measurements of transpososome binding to Atto565-labeled target DNAs. Dots are experimental data, with error bars representing 95% confidence intervals derived from 15 measurements (see Materials and methods). Solid lines represent fits to the data to obtain the KD values indicated in the legends. (A) Wild-type transpososomes, (B) Δ domain III transpososomes (see Figure 4A).
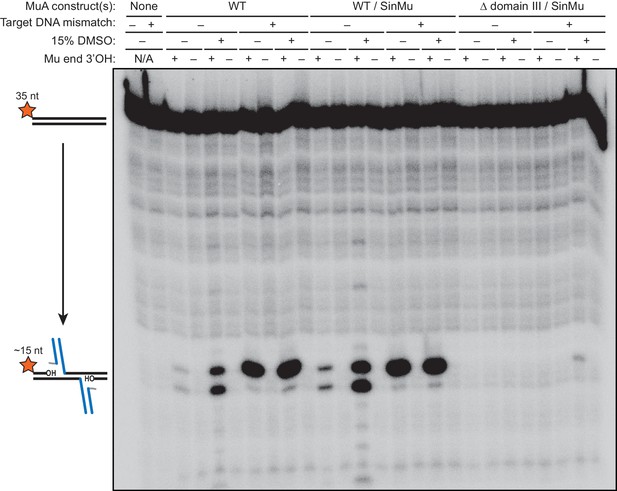
Strand transfer and/or target DNA nicking are blocked by terminating the transferred strand with dideoxy-adenosine.
The results of a 1 hr reaction between 200 nM 5’-32P-labeled target DNA and a pre-formed transpososome mixture using the indicate protein construct(s). The first two lanes are from reactions in which MuA protein and Mu end DNA were omitted. Across all conditions, the reactions in which the transferred strand of the Mu end DNA ended in a 3’ dideoxy-A do not show any evidence of strand transfer or other breaks in the target DNA.
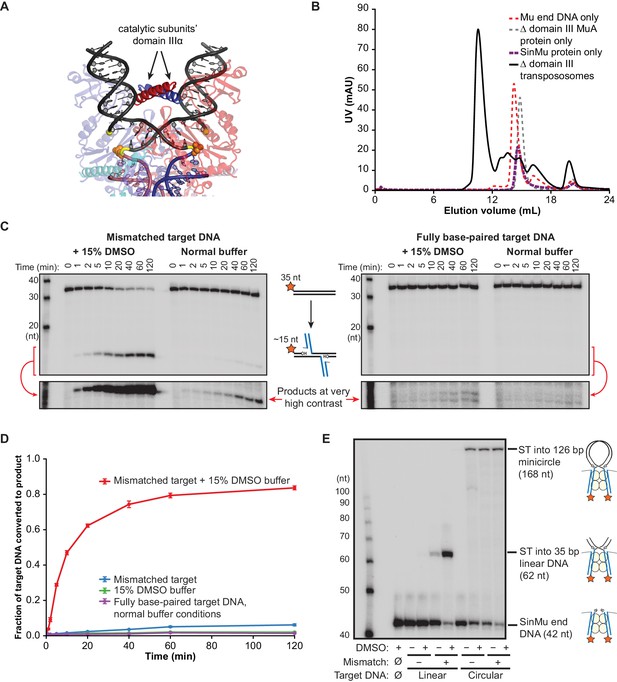
Δ Domain III Transpososomes are rescued by enhanced target DNA bending.
(A) Domain III from the catalytic MuA subunits is used to make contacts to the bent target DNA. The solid (non-transparent) alpha helices indicated with arrows are the portions domain III resolved in the MuA STC crystal structure. Colors as in Figure 1C, with MuA active site residues as orange spheres. (B) Gel filtration chromatography of Δ domain III CDCs. Moving to the chimeric SinMu system and truncating the catalytic MuA subunits to remove domain III does not hinder formation and subsequent purification of CDCs. (C) Strand transfer kinetics visualized by denaturing gel electrophoresis and 5’-32P-labeled target DNA, as in Figure 1D except using Δ domain III transpososomes. Lower panels are the product band(s) from the upper panels at greatly increased contrast. (D) Quantification of the strand transfer kinetics experiments described in (C). The vertical axis represents the fraction of total lane signal present in product band(s). Error bars represent mean ± SEM, n = 4 CDC preparations, one of which is shown in (C). (E) Rescue of the strand transfer activity of truncated transpososomes by circular DNAs. 32P-labeled Mu ends increase in size as a result of strand transfer. Samples were taken 2 hr after transpososomes were mixed with Mg2+ and indicated target DNAs.
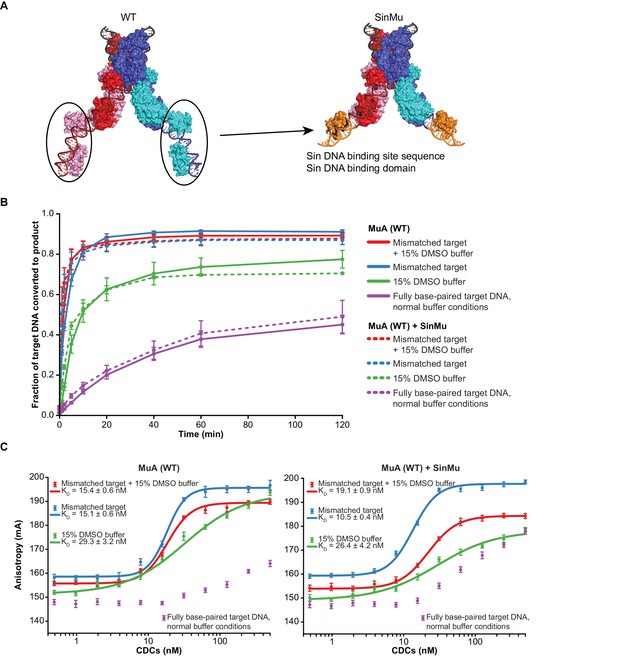
The SinMu system does not inherently alter interactions with target DNA.
(A) A molecular model of the SinMu system. We use this to direct removal of domain III to only the catalytic (upper) subunits in the transpososome. Because the DNA binding domains from non-catalytic subunits (circled) do not contact any other component of the transpososome, they can be altered to be specified by an alternate DNA binding domain and DNA sequence from the Sin recombinase. Colors are as in Figure 1B, with the modeled Sin substitutions in orange. (B) Quantification of strand transfer kinetics experiments, performed as described for Figure 2. Solid lines represent the activity of wild type MuA transpososomes, and are the same data plotted in Figure 2C. Dashed lines represent SinMu transpososomes in which the catalytic subunits are WT (not truncated), under identical conditions. Error bars represent the mean ± the standard error of the mean (SEM) from four independent time-series. (C) Fluorescence anisotropy measurements of target DNA binding. The left panel is the same data as in Figure 3A. The results of identical experiments performed with SinMu transpososomes are shown in the right panel. Error bars represent 95% confidence intervals derived from five technical replicates of 3 independent concentration series (15 total data points, see Materials and methods).
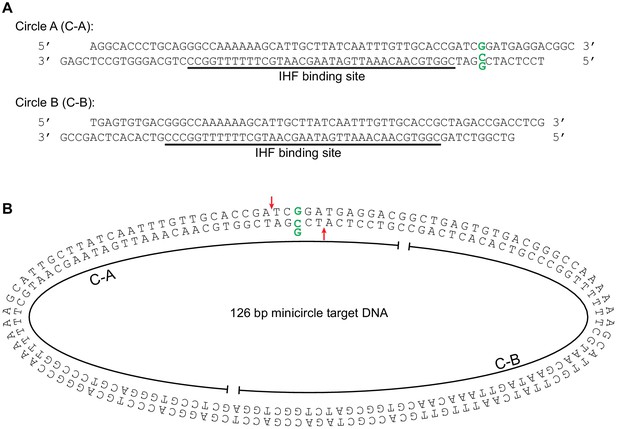
Minicircle target DNA.
(A) Minicircle DNA was constructed from the two linear DNA fragments shown: Circle A (C–A) and Circle B (C–A). Each piece included an approximately centered high affinity binding site for the DNA bending protein IHF. These fragments end in reciprocal complementary four nt overhangs for annealing and ligation.The C-A fragment was designed to optionally include a G:G base-pairing mismatch at the position indicated by the bold green residues. (B) Minicircle target DNA. This is the 126 bp circular target DNA used in Figure 4. The component linear fragments from (A) are indicated. The position of the optional G:G mismatched base pair is marked again in bolded green, and the strand transfer attack positions it would produce are marked again with red arrows. The oblong shape is given for illustrative convenience and is not meant to have any correlation the actual physical conformation of the minicircle DNA molecule.
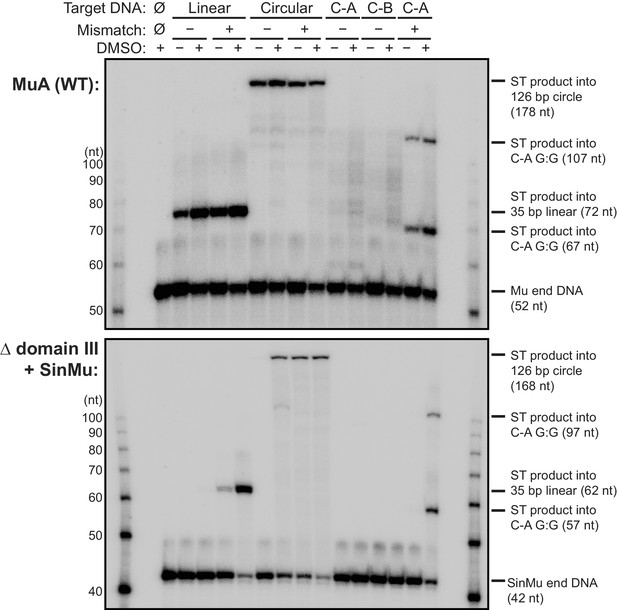
Minicircle rescue of Δ domain III transpososome strand transfer activity is not an artifact of the sequences of the DNAs used to form the minicircles.
In these experiments, the Mu end DNA used to form transpososomes was labeled with 32P. For each lane, the target DNA substrate, presence of a G:G mismatched base-pair in that substrate, and the presence of DMSO in the reaction buffer are indicated above the images. The left half of the bottom panel is the same image as in Figure 4E. C-A and C-B target DNAs are the linear fragments that were ligated to form the circular target (given in Figure 4—figure supplement 2); C-A included the G:G mismatch where indicated. Note that the mismatch-bearing C-A is the only circle component able to rescue Δ domain III strand transfer activity on its own, and only in the presence of DMSO, which is the same behavior as for the primary linear fragment used throughout this work.
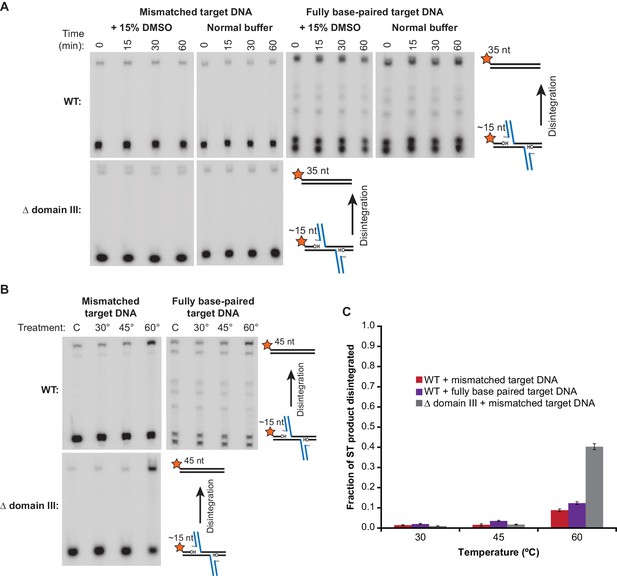
Strand Transfer Complex Disintegration.
(A) Disintegration is not detectable under normal reaction conditions. Substrates and potential products were visualized by denaturing gel electrophoresis and 5’-32P-labeled target DNA. STCs were rapidly purified by immobilization on magnetic beads into normal reaction buffer (see Materials and methods), which included DMSO where indicated, and incubated at 30°C. (B) Disintegration under modified reaction conditions. The same procedure as in (A), but STCs were purified into a modified buffer (see text) and held for 1 hr at the indicated temperatures. Target DNAs in these experiments have 5 bp added to each end to prevent melting during heating. Lanes labeled C are a sample taken immediately after purification, as in the 0 min timepoint in (A). (C) Quantification of replicates of the experiment shown and described in (B). The vertical axis represents the fraction of strand transfer product present immediately after purification that became re-ligated after treatment. Error bars represent mean ± SEM, n = 4 independent STC purifications.
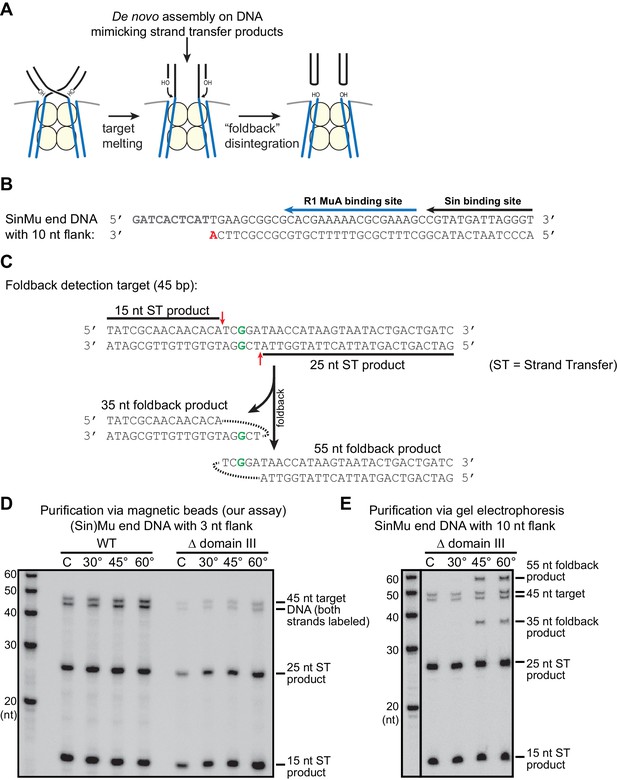
Pseudo-disintegration of strand transfer products by the ‘foldback’ pathway.
(A) A schematic of foldback disintegration. Mu STCs react to the melting of the 5 bp overlap in the target DNA by catalyzing cleavage of the Mu end-target DNA junctions using a target DNA 3’OH as the nucleophile. The difference between foldback, shown here, and a true reversal of the strand transfer reaction lies in the choice of which target 3’OH is used in the reaction. Although the Mu end DNA products are the same, the target site becomes split into two hairpin ends. (B) The sequence of a SinMu end DNA fragment with 10 nt of flanking DNA past the cleavage site (compare to Supplemental Figure 1A). We find that Mu end DNAs with this extended single stranded flank can trigger foldback disintegration under certain conditions. As in Supplemental Figure 1A, the nucleophile for strand transfer is shown in bold and red, and protein binding sites are indicated by black and blue arrows. (C) A target DNA fragment designed for simple detection of foldback products. The products of foldback disintegration from target DNA fragments where strand transfer occurs approximately in the center of the sequence (as is the case elsewhere in this work) are the same length (once denatured) as the input target DNA fragment, which makes the products of true vs. foldback disintegration difficult to differentiate. The target DNA fragment here uses an off-center mismatched base pair (bold green) to direct strand transfer to positions (red arrows) where it is possible to differentiate the 5’-labeled intact target DNA (45 nt) from strand transfer products (15 and 25 nt) and from foldback products (35 and 55 nt). (D) Our disintegration assay does not trigger foldback disintegration. Here, the same protocol as in Figure 5 was used, but the target DNA was that from (C) and labeled at both 5’ ends, and incubation time was 25 min. The expected strand transfer products are present, but foldback products are not. (E) Foldback disintegration is triggered under certain conditions. Here, we used the Mu end DNA described in (B) to form reversal-prone (see Figure 5) Δ domain III strand transfer complexes. Purification of these STCs by gel electrophoresis followed by supplementation of MgCl2 and heating for 25 min. using the protocol from (Au et al., 2004) does trigger foldback disintegration at high temperatures (note the new 35 and 55 nt products in the 60° lane). This demonstrates our ability to detect foldback if it occurs.
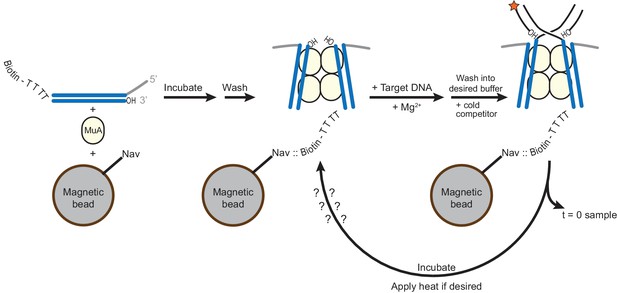
Method for generating STCs for disintegration experiments.
A schematic for the experiments performed in Figure 5. CDCs are assembled on Mu end DNAs in which the transferred strand is labeled at the 5’ end with biotin (see also Figure 1—figure supplement 1). Four unpaired thymidine residues separate the biotin label from the Mu end DNA sequence. Magnetic beads coated in Neutravidin (Nav) are present during CDC assembly. Excess MuA and Mu end DNA is then washed away, and replaced by buffer containing Mg2+ and the intended 32P-labeled target DNA to permit strand transfer to occur. Excess labeled target DNA is then also washed away, the buffer (optionally) exchanged to increase pH and glycerol content, and cold mismatch-bearing target DNA competitor is added. Immediately, a sample is taken to record the baseline level of residual unreacted labeled target DNA, and then samples can be taken to detect disintegration as a function of time and/or incubation temperature.
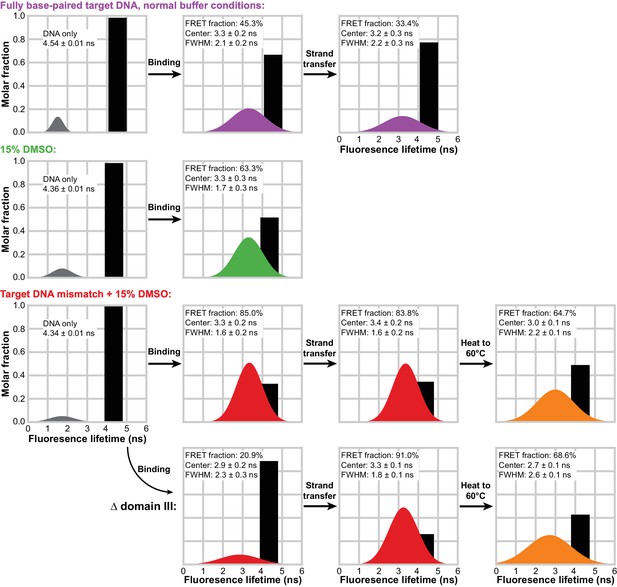
Target DNA bending measured by TCSPC FRET.
The measured fluorescence decay for each condition was fit with a model combining a discrete lifetime (black bars) and a distribution of lifetimes (colored gaussian distributions). In each plot, the area under each component represents its molar fraction in the fit; the center, full width at half maximum (FWHM) and relative abundance is given for each FRET distribution. In the absence of transpososomes (left-most column), little to no FRET can be detected and the vast majority of fluorophores decay with a discrete lifetime. This discrete lifetime was held constant as CDCs lacking the strand transfer nucleophile (second column) and active CDCs (third column) were added. Δ domain III transpososomes were used for the bottom row. In the fourth column, the STC samples from the bottom two rows were heated to 60°C for 5 min and measured again.
-
Figure 6—source data 1
Time correlated single photon counting FRET data.
TCSPC FRET data are organized into folders by date of collection so that the data can be paired with their proper instrument response function (IRF). For each measurement taken, three files are given: (1). ifx: The raw output data from the Vinci fluorometer control software (2). txt: A tab delimited text representation of the data, first column is time (ns) and the second is photon counts (3). pdf: The output of the lifetime fitting done in the Vinci control software and represented in Figure 6. Filenames are descriptive of the sample and conditions: WT or D3: Wild-type transpososomes, or SinMu transpososomes lacking domain III on the catalytic subunits. DMSO: Where present, indicates 15% (v/v) DMSO in the buffer. Mismatch: Where present, indicates that the labeled target DNA contained the central G:G base pairing mistmatch. DO or DA: Target DNA was singly labeled with donor Atto565 fluorophore, or labeled with both donor Atto565 and acceptor Atto647N at opposite ends. 60c: Where present, indicates that the sample and vessel were heated to 60 degrees celsius prior to measurement.
- https://doi.org/10.7554/eLife.21777.018
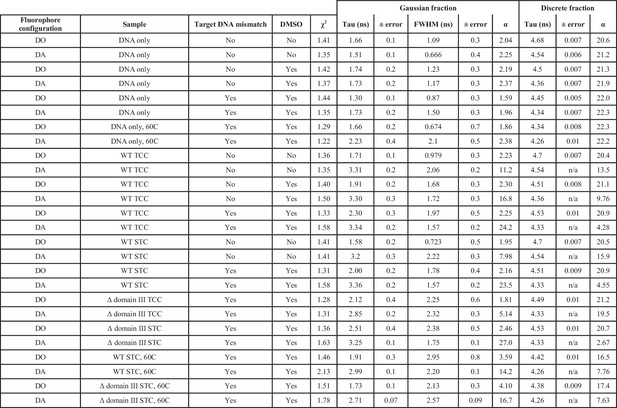
Fluorescence lifetime fits across all samples.
Each sample was fit as the sum of one gaussian and one discrete lifetime. A donor only fluorophore configuration indicates that the Atto647N FRET acceptor fluorophore was omitted from the target DNA. In the case of DNA only and Donor only samples, no variables were held constant. In samples with transpososomes present, the discrete lifetime from the matching DNA-only sample was held constant (and thus has no associated error) in order to account for an unbound/unbent/singly-labeled fraction. FWHM: Full width at half maximum. TCC: target capture complex, CDCs lacking the 3’OH strand transfer nucleophile were used. STC: strand transfer complex, CDCs fully capable of strand transfer were used. α refers to the pre-exponential factor for the decay component. DO, Donor fluorophore only; DA, Donor and Acceptor fluorophores present.
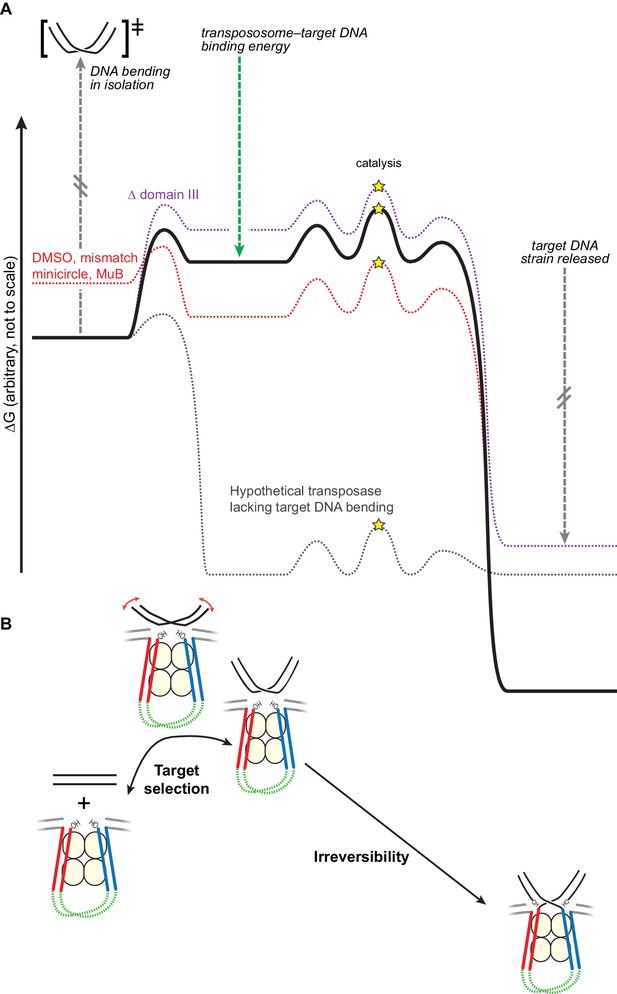
Bending the target DNA slows target capture and resists disintegration.
(A) Cartooned as a simplified free energy diagram, with the unmodified system as the bold black line. Target DNA capture is energetically uphill because the target DNA must be bent. This equilibrium can be altered to favor target capture by factors that promote DNA flexibility (here, mismatched base-pairs, DMSO buffer, or mini-circularization, dashed red line), or to make target capture even more uphill by removing some contacts between the transpososome and the target DNA (here, deleting domain III, dashed purple line). Strand transfer interrupts the target DNA backbone, thus releasing the conformational strain induced by the bend while preserving or solidifying the protein-DNA contacts between the transpososome and target. This leaves the strand transfer products in a deep thermodynamic optimum that prevents disintegration. Our results indicate that domain III stabilizes the target DNA conformation to a greater degree after strand transfer. The dashed grey line represents a hypothetical transposase that binds to target DNA favorably and without inducing any conformational strain. (B) The features of the energy landscape outlined in (A) have the effect of resisting target DNA capture until an outside factor (e.g., MuB) can compensate for the uphill free energy difference. After strand transfer, the release of bending strain combined with product binding energy ensure that strand transfer is effectively irreversible.