A population of adult satellite-like cells in Drosophila is maintained through a switch in RNA-isoforms
Abstract
Adult stem cells are important for tissue maintenance and repair. One key question is how such cells are specified and then protected from differentiation for a prolonged period. Investigating the maintenance of Drosophila muscle progenitors (MPs) we demonstrate that it involves a switch in zfh1/ZEB1 RNA-isoforms. Differentiation into functional muscles is accompanied by expression of miR-8/miR-200, which targets the major zfh1-long RNA isoform and decreases Zfh1 protein. Through activity of the Notch pathway, a subset of MPs produce an alternate zfh1-short isoform, which lacks the miR-8 seed site. Zfh1 protein is thus maintained in these cells, enabling them to escape differentiation and persist as MPs in the adult. There, like mammalian satellite cells, they contribute to muscle homeostasis. Such preferential regulation of a specific RNA isoform, with differential sensitivity to miRs, is a powerful mechanism for maintaining a population of poised progenitors and may be of widespread significance.
https://doi.org/10.7554/eLife.35954.001Introduction
Growth and regeneration of adult tissues depends on stem cells, which remain undifferentiated while retaining the potential to generate differentiated progeny. For example, muscle satellite cells (SCs) are a self-renewing population that provides the myogenic cells responsible for postnatal muscle growth and muscle repair (Chang and Rudnicki, 2014). One key question is how tissue specific stem cells, such as satellite cells, are able to escape from differentiation and remain undifferentiated during development, to retain their stem cell programme though-out the lifetime of the animal.
It has been argued that the progenitors of Drosophila adult muscles share similarities with satellite cells and thus provide a valuable model to investigate mechanisms that maintain stem cell capabilities (Aradhya et al., 2015; Figeac et al., 2007). After their specification during embryogenesis, these muscle progenitors (MPs) remain undifferentiated throughout larval life before differentiating during pupal stages. For example, one population of MPs is associated with the wing imaginal disc, which acts as a transient niche, and will ultimately contribute to the adult flight muscles. These MPs initially divide symmetrically to amplify the population. They then enter an asymmetric division mode in which they self-renew and generate large numbers of myoblasts that go on to form the adult muscles (Gunage et al., 2014). In common with vertebrates, activity of Notch pathway is important to maintain the MPs in an undifferentiated state (Gunage et al., 2014; Mourikis and Tajbakhsh, 2014; Mourikis et al., 2012; Bernard et al., 2010). To subsequently trigger the muscle differentiation program, levels of Myocyte Enhancer factor 2 (Mef2) are increased and Notch signalling is terminated (Elgar et al., 2008; Bernard et al., 2006). Until now it was thought that all MPs followed the same fate, differentiating into functional muscles. However, it now appears that a subset persist into adulthood forming a population of satellite-like cells (Chaturvedi et al., 2017 and see below)). This implies a mechanism that enables these cells to escape from differentiation, so that they retain their progenitor-cell properties.
The Drosophila homologue of ZEB1/ZEB2, Zfh1 (zinc-finger homeodoman 1), is a candidate for regulating the MPs because this family of transcription factors is known to repress Mef2, to counteract the myogenic program (Siles et al., 2013; Postigo et al., 1999). Furthermore, zfh1 is expressed in the MPs when they are specified in the embryo and was shown to be up-regulated by Notch activity in an MP-like cell line (DmD8) (Figeac et al., 2010; Krejcí et al., 2009). In addition, an important regulatory link has been established whereby microRNAs (miRs) are responsible for down-regulating ZEB/Zfh1 protein expression to promote differentiation or prevent metastasis in certain contexts (Zaravinos, 2015; Vandewalle et al., 2009). For example, the miR-200 family is significantly up-regulated during type II cell differentiation in fetal lungs, where it antagonizes ZEB1 (Benlhabib et al., 2015). Likewise, miR-8, a miR-200 relative, promotes timely terminal differentiation in progeny of Drosophila intestinal stem cells by antagonizing zfh1 and escargot (Antonello et al., 2015). Conversely, down-regulation of miR-200 drives epithelial mesenchymal transition (EMT) to promote metastasis in multiple epithelial derived tumours (Korpal et al., 2008; Park et al., 2008). Such observations have led to the proposal that the ZEB/miR-200 regulatory loop may be important in the maintenance of stemness, although examples are primarily limited to cancer contexts and others argue that the primary role is in regulating EMT (Antonello et al., 2015; Brabletz and Brabletz, 2010). The MPs are thus an interesting system to investigate whether this regulatory loop is a gatekeeper for the stem cell commitment to differentiation.
To investigate the concept that ZEB1/Zfh1 could be important in sustaining progenitor-type status, we examined the role and regulation of zfh1 in Drosophila MPs/SCs. Our results show that zfh1 plays a central role in the maintenance of undifferentiated MPs and, importantly, that is expression is sustained in a population of progenitors that persist in adults (pMPs) through the activity of Notch. Specifically these pMPs express an alternate short RNA isoform of zfh1 that cannot be targeted by miR-8. In contrast, the majority of larval precursors express a long isoform of zfh1, which is subject to regulation by miR-8 so that Zfh-1 protein levels are suppressed to enable differentiation of myocytes. Expression of alternate zfh1-short isoform is thus a critical part of the regulatory switch to maintain a pool of progenitor ‘satellite-like’ cells in the adult. This type of regulatory logic, utilizing RNA isoforms with differential sensitivity to miRs, may be of widespread relevance for adult stem cell maintenance in other tissues.
Results
Zfh1 is required for maintenance of muscle progenitors
As zfh1 was previously shown to antagonize myogenesis (Siles et al., 2013; Postigo et al., 1999) it is a plausible candidate to maintain the muscle progenitor (MP) cells in Drosophila and prevent their differentiation. Its expression is consistent with this hypothesis as Zfh1 is present throughout the large group of MPs associated with the wing disc, which can be distinguished by the expression of Cut (Ct) (Figure 1A–A’’). At early stages Zfh1 expression is uniform (Figure 1—figure supplement 1), but at later stages the levels become reduced in the cells with high Cut expression (Figure 1A’’). These cells give rise to the direct flight muscles (DFMs), whereas the remaining MPs, where Zfh1 expression is high, give rise to the indirect flight muscles (IFMs) (Figure 1A’’; Sudarsan et al., 2001). Zfh1 expression in MPs is therefore regulated in a manner that correlates with different differentiation programs.
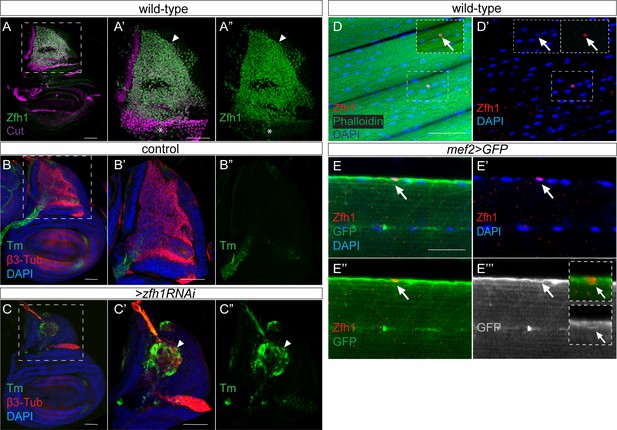
Zfh1 expression and function in MPs and in adult pMPs.
(A–A”) Zfh1 (Green) and Cut (Purple) expression in MPs associated with third instar wing discs, (A’–A’’) higher magnification (3X) of boxed region in A. Zfh1 is present in all MPs, but those with highest Cut expression have lower levels of Zfh1 (asterisk). Scale bars: 50 μM, (n > 30 wing discs from three biological replicates). (B–C) Down regulation of zfh1 induces premature differentiation of the MPs (arrowhead in C’-C’’). β3-Tubulin (β3-Tub, Red) and Tropomyosin (Tm, Green) expression in control (B, 1151-Gal4 > wRNAi) and Zfh1 depleted (C, 1151-Gal4 > zfh1 RNAi) third instar wing discs, (B’–C’’) higher magnification (3X) of boxed regions in B and C. (n > 20 wing discs; from three biological replicates). (D–D’) Zfh1 expression (red) indicates the existence of persistent muscle progenitors (pMPs; arrows) associated with the muscle fibres (Phalloidin (Green), DNA/Nuclei (Blue); n > 10 heminota; from three biological replicates). The immune cell marker P1 was included in the immunostaining and is absent from the pMPs (see Figure 1—figure supplement 2). Scale bars: 50 μM. (E–E’’’) Zfh1 (Red) expressing pMPs (e.g. arrows in E’’’) are closely embedded in the muscle lamina of the adult indirect flight muscles and express Mef2 (myogenic cells; Mef2-Gal4 >Src::GFP, green). Nuclei (Blue), Scale bars: 25 μM, (n > 10 heminota; from two biological replicates).
To determine whether Zfh1 is required in the MPs to antagonize myogenic differentiation we tested the consequences from silencing zfh1 specifically in MPs, using 1151-Gal4 to drive expression of interfering RNAs (RNAi). Two independent RNAi lines led to the premature expression of Tropomyosin (Tm), a protein normally expressed in differentiated muscles, in the most severe (KK103205 line)~80% of zfh1-depleted wing discs exhibited Tm expression (Figure 1B–C and Figure 1—figure supplement 1D–G). Similarly, expression of a Myosin Heavy Chain (MHC) reporter was detected in ~20% of zfh1 (KK103205) depleted wing discs (Figure 1—figure supplement 1H–I) indicating that small muscle fibers had formed precociously. Consistent with the premature expression of these muscle differentiation markers, decreased zfh1 led to abnormal β−3Tubulin staining, showing that the residual cells had altered cell morphology in 90% of zfh1-depleted wing discs, (Figure 1B’–C’). These results demonstrate that reduced zfh1 expression causes MPs to initiate the muscle differentiation program indicating that Zfh1 is required to prevent MP differentiation.
Lineage tracing experiments suggest that a subset of wing disc MPs have characteristics of muscle stem cells and remain undifferentiated even in adult Drosophila. Recently, these have been shown to express Zfh1 (Chaturvedi et al., 2017; Gunage et al., 2014), which is compatible with our observation that Zfh1 is necessary to prevent differentiation in MPs. In agreement, adult IFM muscle fibers were associated with sparse nuclei that retained high levels of Zfh1 expression whereas the differentiated muscle nuclei exhibited no detectable expression (Figure 1D–D’). To better characterise these Zfh1 positive (+ve) adult cells, we expressed a membrane-tagged GFP (UAS-Src::GFP) under the control of a specific muscle driver Mef2-Gal4 (Mef2 >GFP). This confirmed that Zfh1 was expressed in myogenic Mef2 >GFP expressing cells, and revealed that these cells were closely embedded in the muscle lamina (Figure 1E–E’’’). Although many of the Zfh1 expressing cells were clearly co-expressing mef2 >GFP, Zfh1 was also detected in another population that lacked Mef2 expression. Often clustered, these cells were co-labelled with a plasmatocyte marker P1/Nimrod indicating that they are phagocytic immune cells (Figure 1—figure supplement 2). A subset of the Zfh1 +ve cells are therefore myogenic and have characteristics of persistent muscle progenitors that likely correspond to the so-called adult satellite cells recently identified by others (Chaturvedi et al., 2017) (Figure 1D–E).
The results demonstrate that Zfh1 is expressed in MPs, where it is required for their maintenance, and that its expression continues into adult-hood in a small subset of myogenic cells. If, as these data suggest, Zfh1 is important for sustaining a population of a persistent adult progenitors, there must be a mechanism that maintains Zfh1 expression in these cells while the remainder differentiate into functional flight muscles.
zfh1 enhancers conferring expression in MPs
To investigate whether the maintenance of Zfh1 expression in larval and adult MPs could be attributed to distinct enhancers, we screened enhancer-Gal4 collections (Jenett et al., 2012; Jory et al., 2012; Manning et al., 2012) to identify zfh1 enhancers that were active in larval MPs. From the fifteen enhancers across the zfh1 genomic locus that were tested, (Figure 2 and Figure 2—figure supplement 1A) three directed GFP expression in the Cut expressing MPs at larval stages (Figure 2B–D). These all correlated with regions bound by the myogenic factor Twist in MP-related cells (Figure 2—figure supplement 1A; Bernard et al., 2010). Enhancer 1 (Enh1; VT050105) conferred weak expression in scattered progenitors (Figure 2B). Enhancer 2 (Enh2; VT050115) was uniformly active in all MPs and also showed ectopic expression in some non-Cut expressing cells (Figure 2C). Finally, Enhancer 3 (Enh3; GMR35H09) conferred expression in several MPs with highest levels in a subset located in the posterior (Figure 2D). Enh3 encompasses a region that was previously shown to be bound by Su(H) in muscle progenitor related cells, hence may be regulated by Notch activity (Figure 2—figure supplement 1A; Bernard et al., 2010; Krejcí et al., 2009). These results demonstrate that several enhancers contribute to zfh1 expression in the MPs.
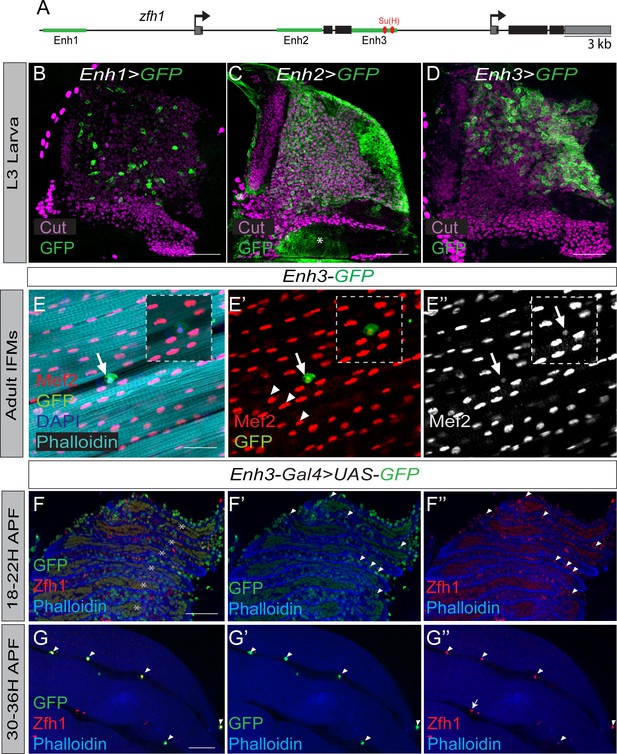
Regulation of zfh1 in MPs and adult pMPs.
(A) Schematic view of zfh1 genomic region, zfh1 regulatory enhancers are represented by green rectangles and arrows indicate transcription start-sites. Coding exons and untranslated regions are represented in black and grey boxes, respectively. (B-D) Three different zfh1 enhancers are active in the MPs (labelled with Cut, purple). Enh1 (VT050105, B) drives GFP (Green) in a subset of scattered MPs; Enh2 (VT050115, C) drives GFP throughout the MPs, and in some non-MP cells (asterisk); Enh3 (GMR35H09, D) is highly expressed in a subset of MPs located in the posterior region of the notum. Scale bars: 50 μM. (n = 30 wing discs). (E-E’’) Enh3-GFP (Green) expression is maintained in adult pMPs (characterised by low levels of Mef2, red; arrows E’-E’’) but not in differentiated muscle nuclei (high Mef2, red; arrowheads G’-G’’). Phalloidin marks muscles (Cyan) and DAPI labels all nuclei (Blue). Insets: boxed regions magnified 12.5 X. Scale bars 25 μM. (n = 10 heminota; from two biological replicates). (F-G) Muscle (IFM) preparation isolated from Enh3-Gal4 > UAS GFP pupae at 18–22 hr APF (F) or at 30–36 hr APF (G). Enh3-GFP (Green) and Zfh1 (Red) are detected in MPs. (F) At 18–22 hr Enh3-GFP activity is higher (arrowheads in E’) in some undifferentiated MPs located between muscle templates (muscles are labeled with Phalloidin, Blue, asterisks). (G) At 30–36 hr APF, Enh3-GFP (Green) activity and Zfh1 (Red) are detected in pMPs (arrowheads) and not in differentiated muscle nuclei. A few Zfh1 +ve cells do not express Enh3-GFP (Arrows G’’). Note: anti-P1, an immune cell marker, was included in the staining to exclude plasmatocytes from the analysis (see Figure 1—figure supplement 2).
To determine which enhancer(s) are also capable of conferring zfh-1 expression in adult pMPs we assessed their activity in adult muscle preparations. Only Enh3 exhibited any activity in these cells (Figure 2E), where it recapitulated well Zfh1 protein expression (Figure 2E–E’’). Thus, Enh3-GFP was clearly detectable in scattered cells, which were closely apposed to the muscle fibers and contained low levels of Mef-2 (Figure 2E’’), and was not expressed in differentiated muscle nuclei (Figure 2).
During pupal stages MPs migrate and surround a set of persistent larval muscles that act as scaffolds for the developing IFMs (Roy and VijayRaghavan, 1998; Fernandes et al., 1991). By 18–22 hr after puparium formation (APF), fusion of myoblasts is ongoing and by 30–36 hr APF, most myoblasts have fused and myogenesis is advanced. By this stage, Zfh1 expression is already restricted to single cells (Chaturvedi et al., 2017). We therefore examined Enh3 activity during the pupal period, using Enh3-Gal4 > UAS GFP, which yields a higher level of expression than the direct Enh3-GFP fusion. At 18–22 hr APF, Enh3 activity was detected in both differentiating myoblasts (inside muscle templates) and undifferentiated MPs (between and around muscle templates) (Figure 2F’–F’’). Importantly, a subset of MPs located between muscle templates exhibited higher levels of Enh3 expression and of Zfh1 levels (Figure 2F’), whereas lower levels were present in the differentiating myoblasts. By 30–36 hr APF Enh3 expression was restricted to pMPs, which expressed high level of Zfh1 and were closely apposed to the muscle fibers (Figure 2G–G’’). At the same stage, we consistently detected a small number of Zfh1 +ve cells that lack detectable Enh3 expression and we speculate that these are undergoing differentiation, since myogenesis is still ongoing at this stage (Figure 2G). In general however, there is a strong correlation between Enh3 expression and the establishment of the adult Zfh1 +ve pMPs, suggesting that Enh3 is responsible for maintaining zfh1 transcription in this progenitor population during the transitionary phase between 20 hr and 30 hr APF.
If Enh3 is indeed responsible for expression of zfh1 in MPs and pMPs, its removal should curtail zfh1 expression in those cells. To test this, Enh3 was deleted by Crispr/Cas9 genome editing (∆Enh3; see Materials and methods). ∆Enh3 homozygous flies survived until early pupal stages allowing us to analyze the phenotype at larval stages. As predicted, ∆Enh3 MPs exhibited greatly reduced Zfh1 protein expression (Figure 2—figure supplement 1B–D) that correlated with decreased zfh1 mRNA levels (Figure 2—figure supplement 1E). Although striking, the effects of ∆Enh3 did not phenocopy those of depleting zfh1 using RNAi, as no premature up-regulation of muscle differentiation markers (MHC, Tm) occurred in ∆Enh3 discs (data not shown). This is likely due to residual zfh1 mRNA/protein (Figure 2—figure supplement 1), brought about by the activity of other zfh1 enhancers (e.g. Enh1 and Enh2, Figure 2B–C). Nevertheless, it is evident that Enh3 has a key role in directing zfh1 expression in MPs/pMPs.
Adult Zfh1 +ve MP cells contribute to flight muscles
By recapitulating Zfh1 in adult MPs, Enh3 provides a powerful tool to investigate whether the persistent MPs are analogous to muscle satellite cells, which are able to divide and produce committed post-mitotic myogenic cells that participate in muscle growth and regeneration. To address this we used a genetic G-trace method, which involves two UAS reporters, an RFP reporter that directly monitors the current activity of the Gal4 and a GFP reporter that records the history of its expression to reveal the lineage (Evans et al., 2009). When Enh3-Gal4 was combined with the G-trace cassette RFP expression was present in the muscle-associated pMPs, which have low Mef2 expression (Figure 3A–A’’). Strikingly, most of the muscle nuclei expressed GFP (Figure 3A’) suggesting that they are derived from ancestral Enh3 expressing cells. Furthermore, close examination of the Enh3 driven RFP expression showed that it often persisted in two nearby muscle nuclei (Figure 3A–A’). This suggests that these nuclei are recent progeny of Enh3-expressing cells, indicating that these cells have retained the ability to divide, a characteristic of satellite cell populations (Figure 3). To further substantiate this conclusion, we verified that adult Zfh1 +ve cells were actively dividing cells, using the mitotic marker phosphohistone-3 (pH3) staining. Many Zfh1 +ve cells co-stained with pH3 indicating that these adult cells remain mitotically active (Figure 3B–B’’).
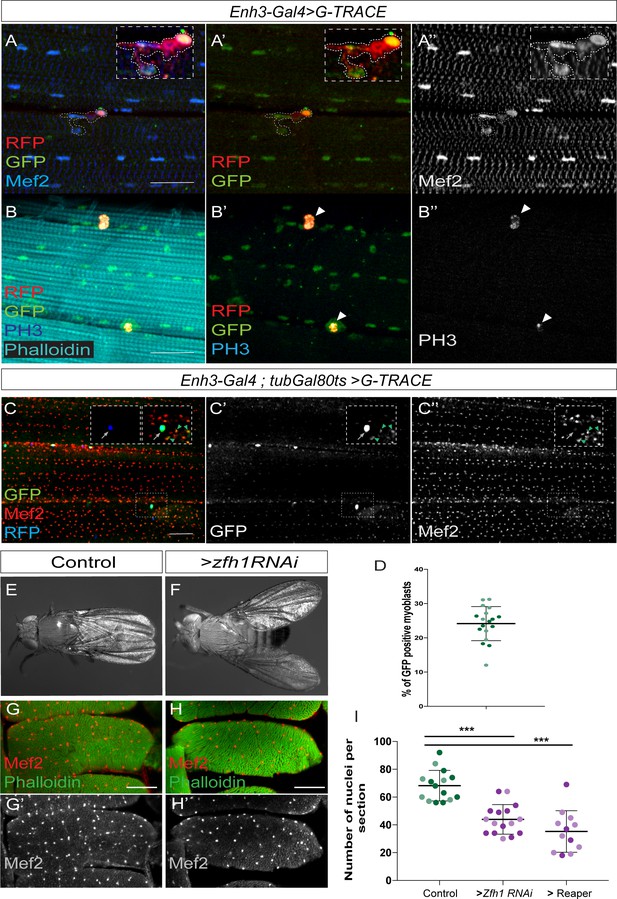
Adult pMPs contribute to muscle homeostasis.
(A-A’’) Lineage tracing shows that adult pMPs contribute to muscles. Cross-section of indirect flight muscles from adult flies where Enh3-Gal4 drives expression of the G-Trace cassette; GFP (Green) indicates myoblasts that have expressed Enh3-Gal4, RFP (Red) indicates myoblasts where Gal4 is still active, Mef2 labels muscle nuclei (Blue). Note that the RFP (Red, detected with anti-RFP in A’) persists in the recently born myoblasts (Mef2), which are closely localized to the pMPs. Insets: boxed regions magnified 20 X (n = 15 heminota; from three biological replicates). (B-B’’) pMPs are mitoticaly active, indicated by anti-phosphH3 (White in B’’). (PH3 detected in 47% of pMPs, n = 80; from three biological replicates). (C-C’’) Cross-section of indirect flight muscles from adult flies (Enh3-Gal4; tubGal80ts > G Trace) where Enh3-Gal4 directed G-Trace activity was induced for 10 days after animal hatching. GFP (Green, Arrowheads, C’) indicates descendants of pMPs (Blue, Arrows); Mef2 labels muscle nuclei (Red, White). (D) Proportion of newly born myoblasts (marked by GFP; e.g. C’) relative to total number of myoblasts (marked by Mef2; e.g. C’’) in muscle preparations. (n = 18 heminota; light and dark shading indicates data points collected from two independent replicates replicates). Scale bar: 60 μM. (E-F) Prolonged zfh1 depletion in pMPs (10 days after adult hatching) leads to a ‘held out’ wings posture; dorsal view of (E) control (Enh3-Gal4; tubGal80ts > UAS wRNAi;) and (F) zfh1 depletion (Enh3-Gal4; tubGal80ts > UAS-zfh1RNAi (KK 103205)) adult flies. (G-H) Transverse sections of DLM4 muscle stained with Phalloidin (Green) and Mef2 (Red, White) from the indicated genotypes. Fewer Mef2 +ve nuclei are present in muscles when zfh1 is depleted. Scale bars: 50 μM. (I) Similar reductions in muscle nuclei occur following zfh1 depletion (Enh3-Gal4; tubGal80ts > UAS-zfh1RNAi) or following genetic ablation of pMPs, via expression of the pro-apoptotic gene reaper (rpr; Enh3-Gal4;tubGal80ts > UAS rpr). The number of nuclei per section in the indicated conditions was significantly different, light and dark shading indicates data points collected from two independent replicates (>zfh1 RNAi ***p=0.0013, n = 16;>Rpr ***p<0.0001, n = 12).
If the mitotically active Zfh1 +ve cells are indeed important for muscle homoestasis, their progeny should become incorporated into the muscle fibres. We therefore quantified the proportion of muscle nuclei derived from the pMPs during the first 10 days of the adult life, by using a temperature sensitive Gal80 (tubGal80ts) to restrict Enh3-Gal4 until eclosion and combining it with the G-Trace cassette to mark the progeny (Figure 3C–D). Strikingly, the conditional activation of Enh3-Gal4 in adults resulted in GFP labeling of ~24% of muscle nuclei (Figure 3D) indicating a significant role of the pMPs in contributing to muscle maintenance. Indeed when we used a similar regime to deplete zfh1 in pMPs and examined flies at ten days (Figure 3E–I) we found that ~ 30% of adult flies had a ‘held out wing’ posture (n = 93) (Figure 3E–F), a phenotype often associated with flight muscle defects (Vigoreaux, 2001). The number of nuclei per muscle (DLM4) was also significantly reduced (~20% fewer nuclei) in the aged adults when zfh1 was specifically depleted in the pMPs (Figure 3G–H). Likewise, genetic ablation of pMPs (by expressing the pro-apoptotic gene reaper) led to a similar reduction in muscle nuclei (Figure 3I). No ‘held out wing’ phenotype or muscle defects were observed in adult flies within 24 hr of zfh1 knock-down, indicating that the phenotypes at 10 days are due to a defect in the homeostasis of the adult flight muscles. Taken together the results argue that the adult Zfh1 +ve myoblasts cells resemble mammalian satellite cells, retaining the capacity to divide and provide progeny that maintain the adult flight muscles.
Notch directly regulates zfh1 expression in muscle progenitors and adults pMPs
As mentioned above, zfh1 is regulated by Notch activity in Drosophila DmD8, MP-related, cells (Krejcí et al., 2009), where Enh3 was bound by Su(H) (Figure 2A and Figure 2—figure supplement 1). Furthermore, phenotypes from depletion of zfh1 in MPs, were reminiscent of those elicited by loss of Notch (N) signaling (Figure 1 and Krejcí et al., 2009). Notch activity is therefore a candidate to maintain Zfh1 expression in the adult pMPs, thereby preventing their premature differentiation. As a first step to test whether Notch activity contributes to zfh1 expression, we depleted Notch in muscle progenitors by driving Notch RNAi expression with 1151-Gal4 (Figure 4A–C). Under these conditions, Zfh1 levels were significantly reduced, consistent with Notch being required for zfh1 expression in MPs. Second, the consequences of perturbing Notch regulation by mutating the Su(H) binding motifs in Enh3 were analyzed. Two potential Su(H) binding sites are present in Enh3 and both are highly conserved across species (Figure 2A). Mutation of both motifs, Enh3[mut], resulted in a dramatic decrease of the enhancer activity in the MPs (Figure 4D–F). This supports the hypothesis that Notch directly controls zfh1 expression in MPs by regulating activity of Enh3.
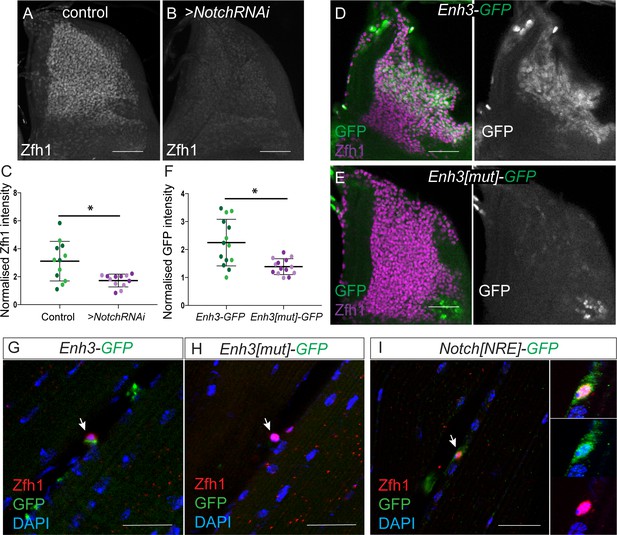
Notch directs Zfh1 expression in MPs and pMPs.
(A-C) Zfh1 level (White) is significantly reduced when Notch is down regulated. Expression of Zfh1 in MPs (A) is severely reduced in the presence of Notch RNAi (B, 1151-Gal4 > UAS NotchRNAi), Scale Bars: 50 μM. (C) Quantification of Zfh1 expression levels (*p<0.05, n = 12 wing discs in each condition, light and dark shading indicates data obtained from two independent replicates). (D-F) Enh3 (D, Enh3-GFP, Green) expression in MPs (Purple, Zfh1) is abolished when Su(H) motifs are mutated (E, Enh3[mut]-GFP). Scale bars: 50 μM. (F) Quantification of expression from Enh3 and Enh3[mut] (*p=0.022, n = 14 wing discs in each condition, light and dark shading indicates data obtained from two independent replicates). (G-H) Enh3 (G, Enh3-GFP, Green) expression in adult pMPs (red, Zfh1) is abolished when Su(H) motifs are mutated (H, Enh3[mut]-GFP, Green), DAPI (Blue) reveals all nuclei. (I) Notch[NRE]-GFP (Green) is co-expressed with Zfh1 (Red) in the pMPs associated with the indirect flight muscles; DAPI (Blue) detects all nuclei. (n = 12 heminota; from two independent replicates). In G-I anti-P1 was included to label immune cells and exclude them from the analysis. Scale bars: 25 μM.
Since Enh3 activity persists in the adult pMPs (Figure 2), we next analyzed whether mutating the Su(H) motifs impacted expression in these adult pMPs. Similar to larval stage MPs, Enh3[mut] had lost the ability to direct expression of GFP in the adult pMPs (Figure 4G–H). Thus, the Su(H) motifs are essential for Enh3 to be active in the adult pMPs. These data support the model that persistence of Zfh1 expression in adult MPs is likely due to Notch input, acting through Enh3.
The results imply that Notch should be expressed and active in the adult pMPs. To investigate this, we made use of a Notch[NRE]-GFP reporter line. Notch[NRE] is an enhancer from the Notch gene, and itself regulated by Notch activity, such that it is a read out both of Notch expression and of Notch activity (Simón et al., 2014). Robust expression of Notch[NRE]-GFP reporter was detected in Zfh1 +ve adult pMPs, confirming that Notch is active in these cells (Figure 4I) but not in the differentiated muscles. Together, the results show that zfh1 expression in the adult pMPs requires Notch activity acting through Enh3.
zfh1 is silenced by the conserved microRNA miR-8/miR-200 in MPs
Although transcriptional control of zfh1 by Notch explains one aspect of its regulation, since all larval MPs express Zfh1 it remained unclear how a subset maintain this expression and escape from differentiation to give rise to adult pMPs. A candidate to confer an additional level of regulation on zfh1 expression is the micro RNA miR-8/miR-200, which is important for silencing zfh1 (and its mammalian homologue ZEB1) in several contexts. The regulatory loop between miR-8/miR-200 and zfh1/ZEB has been extensively studied in both Drosophila and vertebrates and is mediated by a miR-8/miR-200 seed site located in the 3’ untranslated region (3’UTR) (Antonello et al., 2015; Vallejo et al., 2011; Brabletz and Brabletz, 2010). Moreover, miR-8 was previously reported to be involved in flight muscle development (Fulga et al., 2015).
To determine whether miR-8 could down-regulate zfh1 in muscle progenitors to promote their differentiation into muscles, we first examined the spatiotemporal expression pattern of miR-8 at larval, pupal and adult stages (Figure 5) using miR-8-Gal4, whose activity reflects the expression of the endogenous miR-8 promoter (Karres et al., 2007). Expression of miR-8-Gal4 was almost undetectable in the larval MPs, although it was highly expressed in the wing pouch (Figure 5). A low level of miR-8-Gal4 expression was also detected in a subset of the MPs where Zfh1 levels are slightly reduced (high Ct expressing DFM precursors; Figure 5). Thus, expression of miR-8 is inversely correlated with Zfh1; its overall expression is low in larval MPs where Zfh1 expression is important to prevent their differentiation (Figure 5B–B and Figure 1A).
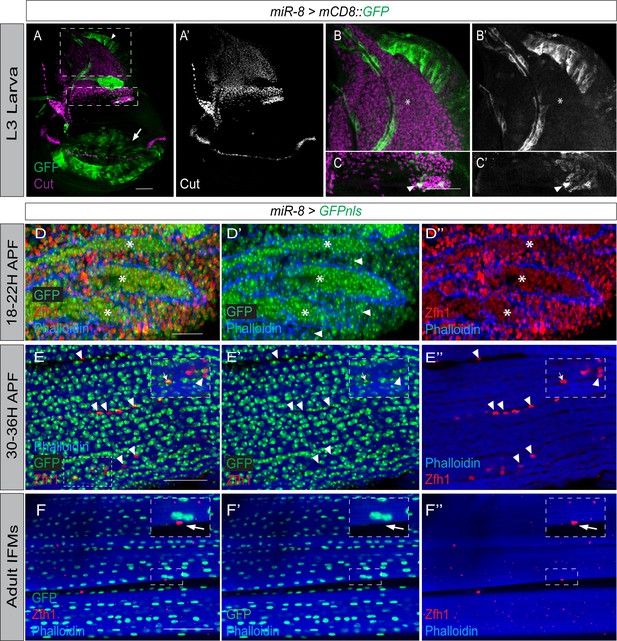
Expression dynamics of miR-8 and Zfh1 in MPs and pMPs during indirect flight muscle development.
(A-C) miR-8 (Green, miR-8-Gal4 > UAS-mCD8::GFP) is not highly expressed in MPs (Cut, Purple) but is prevalent in the wing disc pouch (Arrow), notum (Arrowhead) and air sac (Asterisk). Higher magnification shows that low level of miR-8 expression can be detected in the subset of MPs where Zfh1 is normally low (Arrowhead in C) but not in other MPs. Scale bars: 50 μM. (n > 20 wing discs; from three biological replicates). (D-D’’) IFM preparation isolated from miR-8-Gal4 > UAS-nlsGFP pupae at 18–22 hr APF. At this stage miR-8 (Green; D and D’) is co-expressed with Zfh1 (Red; D and D’’) in MPs but miR-8 is more highly expressed in differentiated myoblasts (Asterisks in D’), found within the muscles (labeled with Phalloidin, Blue), compared to the undifferentiated MPs, found between the muscles (Arrowheads in D’). In contrast, Zfh1 (D, D’) is detected at lower levels in the differentiated myoblasts compared to the undifferentiated MPs (Asterisks in D’). Scale bar: 25 μM. (E-E’’) IFM preparation isolated from miR-8-Gal4 > UAS-nlsGFP pupae at 30–36 hr APF. At this stage, mir-8 is highly detected in the differentiated muscle nuclei. The majority of the Zfh1 +ve pMPs (Red) do not express mir-8 (Green) (Arrowheads). Few Zfh1 +ve pMPs express mir-8 (Arrows). Scale bar: 50 μM. (F-F’’) In adult IFMs, mir-8 (Green) expression is absent from Zfh1 +ve (red) pMPs (Arrows, D-D’) but is present at uniformly high levels in IFMs, (Phalloidin, Blue). Scale bars: 50 μM. (n = 20 heminota; from three biological replicates).
We subsequently compared miR8-Gal4 and Zfh1 expression in 18–22 hr APF pupae (Figure 5D–D’’). At this stage, miR-8-Gal4 and Zfh1 expression overlapped in most, if not all, myogenic nuclei (Figure 5D). However, miR-8-Gal4 expression level was elevated in the differentiated myoblasts, which are located inside the muscle templates (Figure 5D–D’). Conversely, Zfh1 expression level was slightly lower in this population and higher in the undifferentiated MPs (Figure 5D–D’’). Thus miR-8 and Zfh1 have reciprocal low and high expression patterns in the MPs at this stage of myogenesis.
By 30–36 hr APF, Zfh1 expression was restricted to pMPs while miR-8-Gal4 was predominantly expressed throughout the differentiated myoblasts (Figure 5E). Notably, a few of the Zfh1 +ve cells at this stage retained miR-8-Gal4 expression (Figure 5E–E’). Similar to 30–36 hr APF, adult muscles had uniform and high levels of miR-8-Gal4 (Figure 5F–F’’). However, at this time, miR-8-Gal4 expression was totally absent from all Zfh1 +ve adult pMPs (Figure 5F–F’’). These data show that, during muscle formation, miR-8 expression level is inversely correlated to Zfh1; supporting the model that miR-8 negatively regulates zfh1.
Given their complementary expression patterns we next tested the impact of miR-8 overexpression on Zfh1 protein levels in larval MPs (Figure 6A–B). Zfh1 protein levels were significantly diminished under these conditions, in agreement with miR-8 regulating zfh1 post-transcriptionally (Figure 6C). Although this manipulation was not sufficient to cause premature up-regulation of muscle differentiation markers or associated morphological changes in MPs, the few surviving adults all displayed a held-out wing phenotype, which is often associated with defective flight muscles (Vigoreaux, 2001). Next we assessed whether miR-8 activity/expression changes in response to Mef2 levels, a critical determinant of muscle differentiation, using a miR-8 sensor (containing two miR-8 binding sites in its 3’UTR [Kennell et al., 2012]). Expression of the miR-8 sensor was specifically decreased when Mef2 was overexpressed in MPs, suggesting that miR-8 expression responds to high level of Mef2 (Figure 6—figure supplement 1).
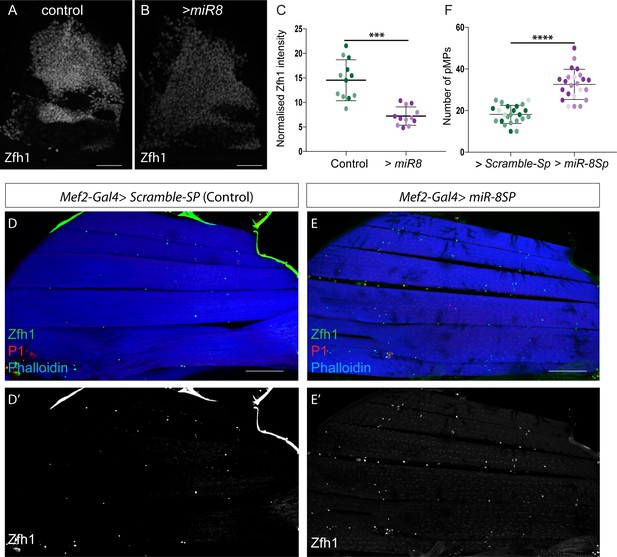
The conserved microRNA miR-8/miR-200 antagonizes zfh1 to promote muscle differentiation.
(A-C) Effect of miR-8 overexpression (1151-Gal4 > UAS-miR-8) on Zfh1 (White) protein level in MPs. Scale Bars: 50 μM. (C) Zfh1 expression is significantly reduced by miR-8 over-expression. (***p=0.0009, n = 12 wing discs in each condition, light and dark shading indicates data points from two independent replicates). (D-E) Sagittal sections of adult IFMs stained for Phalloidin (Blue), Zfh1 (Green) and P1 (Red). Down regulating miR-8 during muscle differentiation (Mef2-Gal4 > UAS-miR-8-Sp) increases the final number of adult pMPs. (F) The number of pMPs in adult IFMs in the indicated conditions was significantly different. (****p<0.0001, n = 18 adults for each genotype; light, dark and intermediate shading indicates data points from three independent replicates). Scale bars: 100 μM.
If down-regulation of zfh1 by miR-8 is important to allow muscle differentiation, selective depletion of miR-8 should allow more MPs to escape differentiation. To achieve this, a miR-8 sponge construct (UAS-miR-8Sp; Fulga et al., 2015) was expressed using Mef2-Gal4, so that it would decrease miR-8 activity in differentiating myoblasts. Adult muscles were still formed under these conditions. However the final number of pMPs was significantly increased (Figure 6D–F). Conversely ectopic expression of miR-8 in adult MPs (using Enh3-Gal4) led to a reduction in the number of muscle nuclei similar to that seen with zfh1 down-regulation (Figure 6—figure supplement 1D). Together, these data argue that miR-8 up-regulation during muscle differentiation blocks Zfh1 production to allow MPs to differentiate and if its expression is compromised, more MPs are permitted to escape differentiation. It is also possible that miR-8 has additional targets, besides Zfh1, that are involved in maintenance/differentiation of MPs.
An alternate short zfh1 isoform is transcribed in adult pMPs
To retain their undifferentiated state, the adult pMPs must evade miR-8 regulation and maintain Zfh1 expression. The zfh1 gene gives rise to three different mRNA isoforms; two long zfh1 isoforms (zfh1-long; zfh1-RE/RB) and one short zfh1 isoform (zfh1-short; zfh1-RA) (Figure 7A). Although zfh1-long isoforms have two additional N-terminal zinc fingers, all three RNA-isoforms produce proteins containing the core zinc finger and homeodomains needed for Zfh1 DNA-binding activity (Figure 7—figure supplement 1A; Postigo et al., 1999). Importantly, zfh1-short isoform has a shorter 3’UTR, which lacks the target site for miR-8 (Figure 7A; Antonello et al., 2015), as well as differing in its transcription start site (TSS) (Figure 7A; Flybase FBgn0004606). The lack of a seed site makes zfh1-short insensitive to miR-8 mediated down-regulation. This means that zfh1-short expression would enable cells to retain high level of Zfh1 protein, even in the context of miR-8 expression and, if present in a subset of MPs, could explaining how they can escape differentiation.
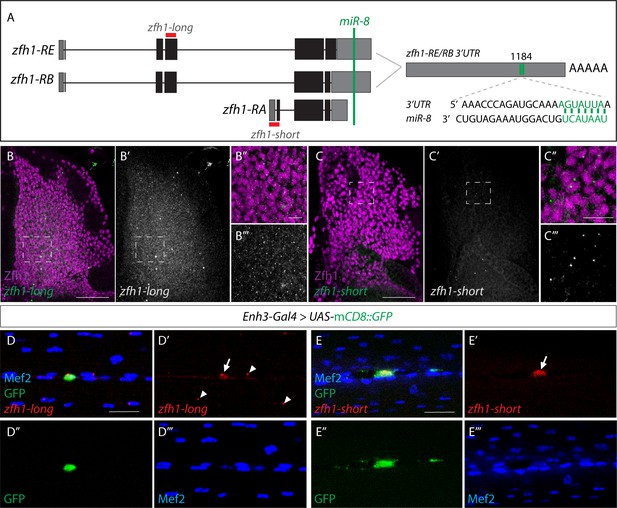
Transcriptional dynamics of zfh1 isoforms in MPs and pMPs.
(A) Schematic representation of zfh1 isoforms. zfh1-short (zfh1-RA) is initiated from a different transcription start site and has shorter 3’UTR that lacks the target site for miR-8 (Green; Antonello et al., 2015) present in zfh1-long isoforms (zfh1-RB, zfh1-RE); the position of the miR-8 seed sites in zfh1-long 3’ UTR are depicted. Non-coding exons and coding exons are depicted by grey and black boxes respectively, red lines indicate the probes used for FISH experiments in B-E. (B-C) zfh1-long is present uniformly in MPs. (n > 10 wing discs from two replicates; B, Green and B’, White) whereas zfh1-short is only detected in a few MPs (n > 15 wing discs from three replicates; C, Green and C’, White), detected by in situ hybridisation in wild type third instar wing discs stained for Zfh1 (Purple). Scale bars: 10 μM. (B’ B’’, C’ C’’) Higher magnifications of boxed regions (Scale bars: 50 μM). (D-E) In adult IFMs zfh1-long is detected in the pMPs (n = 11 pMPs from two replicates, arrow in D’) and in some differentiated nuclei located in their vicinity (arrowheads in D’) whereas zfh1-short is only present in pMPs (n = 15 pMPs from two replicates, arrow in E’). Enh3 expression (Green, Enh3-Gal4 > UAS-mCD8GFP) labels adult pMPs and Mef2 (Blue) labels all muscle nuclei. Scale bars: 20 μM.
To determine whether zfh1-short is indeed expressed in MPs, we designed fluorescent probes specific for the zfh1-long and zfh1-short isoforms and used them for in situ hybridization (FISH) at larval and adult stages (Figure 7A). In larval stages (L3), zfh1-long isoforms were present at uniformly high levels in the MPs (Figure 7B–B’’’) whereas zfh1-short was expressed at much lower levels and only detected in a few MPs in each disc (Figure 7C–C’’’). In adult muscles, where pMPs were marked by Enh3-Gal4 > GFP and low Mef2 expression (Figure 7D–E), high levels of zfh1-short and much lower levels of zfh1-long (Figure 7D–E’’’) were present in the pMPs. Indeed, zfh1-short was only present in the pMPs whereas dots of zfh1-long hybridization were also detected in some differentiated nuclei (with high level of Mef2) (Figure 7). Thus, zfh1-short is expressed in a few larval MPs and is then detected at highest levels specifically in the adult pMPs but is not transcribed in adult muscle nuclei. Since zfh1-short is not susceptible to regulation by miR-8, its specific transcription may therefore be determinant for maintaining high levels of Zfh1 in a subset of progenitors and enable them to escape differentiation.
The model predicts that Zfh1-short will counteract the myogenic program in a similar manner as previously described for Zfh1-long, which antagonizes Mef2 function (Siles et al., 2013). Premature expression of Mef2 (using 1151 Gal4) induces precocious differentiation of larval MPs, evident by ectopic expression of MHC-LacZ and a reduction of MPs (Figure 7—figure supplement 1 and ref). Expression of Zfh1-short was able to counteract the effects of Mef2, suppressing the precocious muscle differentiation phenotype and restoring the normal morphology of MPs. Thus, Zfh1-short retains the capacity to block Mef2 induced muscle differentiation (Figure 7—figure supplement 1).
zfh1-short isoform transcription requires Notch activity in adult pMPs
Expression of zfh1-short (zfh1-RA) is specifically retained in adult MPs (Figure 7E) where it may be critical for maintaining their progenitor status. Mechanisms that ensure this isoform is appropriately transcribed could involve Notch signaling, which is necessary for normal levels of Zfh1 expression in MPs (Figure 4). If this is the case, expression of a constitutively active Notch (Notch∆ECD) should up-regulate zfh1-short transcripts in the MPs at larval stages when their expression is normally low. In agreement, expression of active Notch in the progenitors (1151-Gal4 > Notch∆ECD) significantly increased the proportions of cells transcribing zfh1-short (Figure 8A–B’ and C).
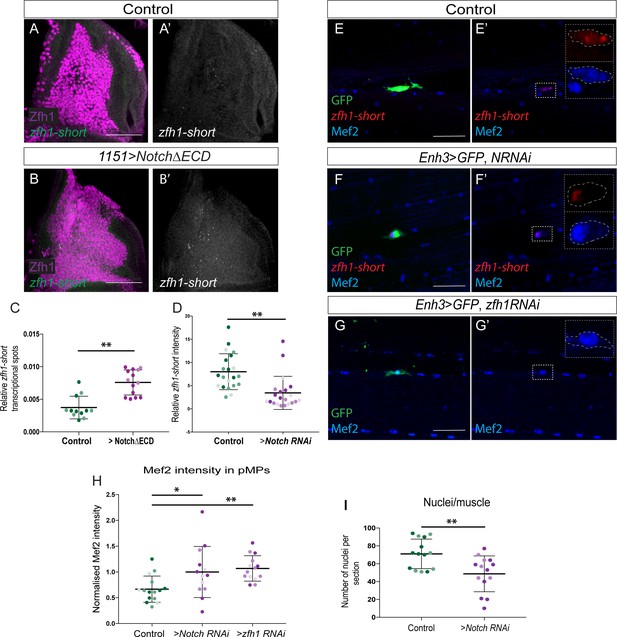
zfh1-short regulation by Notch is important to maintain muscle homeostasis.
(A-C) Expression of an activated Notch (1151-Gal4 > UAS-NΔECD) in MPs induces ectopic zfh1-short transcription. In situ hybridisation detecting zfh1-short (Green) in MPs (Zfh1, Purple) with wild type (A-A’) or elevated Notch activity (B-B’). Scale bars: 50 μM. (C) Quantification showing significant increase in zfh1-short transcriptional dots upon Notch up regulation, relative to total number of MPs (**p<0.01, Student t-test; n = 14 wing discs for each genotype, light and dark shading indicates data points from two independent replicates). (D-H) Notch depletion leads to a severe decrease in zfh1-short (Red) (E’-F’ and D) in pMPs (Green; Enh3-Gal4; UAS-mCD8GFP > UAS Notch-RNAi; tubGal80ts) and to an increase in Mef2 levels (Blue) (E-F’ and H). (G-G’ and H) zfh1 depletion in the pMPs (Enh3-Gal4; UAS-mCD8GFP > UASzfh1 RNAi; tubGal80ts) leads to an increase in Mef2 levels (Blue). Scale bars: 25 μM. Quantifications of zfh1-short (D) and Mef2 (H) in the indicated conditions show that the levels are significantly different (D, n = 21 pMPs for each genotype (**p<0.01); H, n = 15 pMPs for Control RNAi and n = 13 pMPs for Notch RNAi (*p<0.05) and n = 14 pMPs for zfh1 RNAi (**p<0.01)). In each condition light, dark and intermediate shading indicates data points from three independent replicates. (I). Prolonged Notch depletion in the pMPs (Enh3-Gal4; Gal80ts > UAS Notch RNAi) affects the muscle homeostasis. (**p<0.01, n = 14 for each genotype, light and dark shading indicates data points from two independent replicates).
To address whether Notch is necessary for zfh1-short transcription in adult pMPs, we specifically depleted Notch levels after eclosion (using Enh3-Gal4 in combination with tubGal80ts to drive Notch RNAi; Figure 8E–F). Consistent with expression of zfh1-short being dependent on Notch activity, the levels of zfh1-short were significantly reduced in adult pMPs when Notch was down-regulated in this way (Figure 8E–F and D). Conversely, expression of zfh1-long isoform was less affected (Figure 8—figure supplement 1), suggesting other inputs besides Notch help to sustain zfh1-long in pMPs. Nevertheless, Mef2 accumulated to higher levels than normal in the adult pMPs, under these Notch RNAi conditions, indicating that Notch activity helps prevent their differentiation (Figure 8E’, F’ and H), most likely through sustaining a higher level of Zfh1 expression via its regulation of zfh1-short.
The increased level of Mef2 in the pMPs following Notch-depletion suggests they are losing their progenitor status and becoming differentiated. This forced differentiation of the pMPs would deplete the progenitor population and so should compromise muscle maintenance and repair. In agreement there was a significant reduction of the number of nuclei per muscle after ten days of Notch down-regulation in the pMPs (Figure 8I). These results are reminiscent of the targeted zfh1 down-regulation in the adult pMPs (Figure 3G–H and I), where high level of Mef2 was also prematurely detected (Figure 8G–G’ and H). Taken together, the data indicate that persistent Notch activity is required to maintain zfh1-short expression in pMPs, which protects the pMPs from differentiating by ensuring that sufficient Zfh1 protein is present to prevent Mef2 accumulating.
Discussion
A key property of adult stem cells is their ability to remain in a quiescent state for a prolonged period of time (Li and Clevers, 2010). Investigating the maintenance of Drosophila MPs we have uncovered an important new regulatory logic, in which a switch in RNA isoforms enables a sub-population of cells to escape miRNA regulation and so avoid the differentiation program. At the same time, analyzing expression of a pivotal player in this regulatory loop, zfh1, has revealed how this mechanism sustains a population of persistent progenitors associated with adult muscles in Drosophila, that appear analogous to mammalian satellite cells (Figure 9).
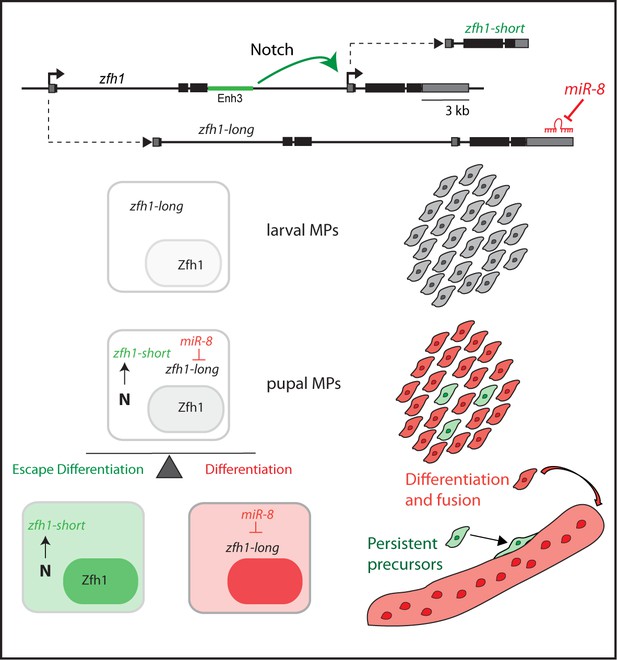
Model summarizing the role of alternate zfh1 isoforms in the maintenance of adult pMPs.
zfh1-long (Grey) is expressed in all MPs at larval stage. Silencing of zfh1-long by miR-8 (Red) facilitates the MPs differentiation. zfh1-short (Green) transcription is driven and maintained in pMPs by a Notch responsive element (Enh3, Green rectangle), which may also contribute to zfh1-long regulation. Because zfh1-short is insensitive to miR-8, Zfh1 protein is maintained in pMPs, enabling them to escape differentiation and persist as MPs in the adult.
Zfh1 maintains a population of satellite-like cells in adult drosophila
Until recently, the fly has been thought to lack a persistent muscle stem cell population, leading to speculation about how its muscles could withstand the wear and tear of its active lifestyle. Now it emerges that expression of Zfh-1 identifies a population of muscle-associated cells in the adult that retain progenitor-like properties (Figure 9 and Chaturvedi et al., 2017). Indeed we have found that Zfh-1 is critical to prevent these progenitor cells from differentiating. Its expression in the persistent adult ‘satellite-like’ cells is dependent on a specific Zfh1 enhancer, which is directly regulated by Notch. Activity of Notch is important for maintaining Zfh1 expression and hence is required to sustain the progenitor status of these cells, similar to the situation in mammalian satellite cells, which require Notch activity for their maintenance (Mourikis and Tajbakhsh, 2014; Bjornson et al., 2012).
Using lineage-tracing method we showed that adult Zfh1 +ve cells, in normal conditions, provide new myoblasts to the fibers. Furthermore, conditional down regulation of zfh1 led adult pMPs to enter differentiation and resulted in flight defects, evident by a held out wing posture. These results demonstrate that Zfh-1 is necessary to maintain these progenitors and that, similar to vertebrate satellite cells, the Zfh1 +ve progenitor cells contribute to the adult muscles homeostasis. Others have recently shown that the pMPs are expanded in conditions of muscle injury where they are likely to contribute to repair (Chaturvedi et al., 2017). Thus the retention of a pool of progenitor cells may be critical to maintain the physiological function of all muscles in all organism types, as also highlighted by their identification in another arthropod Parahyle (Alwes et al., 2016; Konstantinides and Averof, 2014). Drosophila notably differs because their satellite-like cells do not express the Pax3/Pax7 homologue (gooseberry; data not shown), considered a canonical marker in mammals and some other organisms (Chang and Rudnicki, 2014). Nor do they express the promyogenic bHLH protein Twist (data not shown), which is present in the muscle progenitors in the embryo (Bate et al., 1991). Instead, Zfh1 appears to fulfill an analogous function and it will be interesting to discover how widespread this alternate Zfh1 pathway is for precursor maintenance. Notably, the loss of ZEB1 in mice accelerates the temporal expression of muscle differentiation genes (e.g. MHC) suggesting that there is indeed an evolutionary conserved function of Zfh1/ZEB in regulating the muscle differentiation process (Siles et al., 2013). This lends further credence to the model that Zfh1 could have a fundamental role in preventing differentiation that may be harnessed in multiple contexts.
Switching 3' UTR to protect progenitors from differentiation
Another key feature of zfh-1 regulation that is conserved between mammals and flies is its sensitivity to the miR-200/miR-8 family of miRNAs (Antonello et al., 2015; Brabletz and Brabletz, 2010). This has major significance in many cancers, where loss of miR-200 results in elevated levels of ZEB1 promoting the expansion of cancer stem cells, and has led to a widely accepted model in which the downregulation of Zfh1 family is necessary to curb stem-ness (Brabletz and Brabletz, 2010). This fits with our observations, as we find that miR-8 is upregulated during differentiation of the MPs and suppresses Zfh1 protein expression. Critically however, only some RNA isoforms, zfh1-long, contain seed sites necessary for miR-8 regulation (Antonello et al., 2015). The alternate, zfh1-short, isoform has a truncated 3’UTR that lacks the miR-8 recognition sequences and will thus be insensitive to miR-8 regulation. Significantly, this zfh1-short isoform is specifically expressed in MPs that persist into adulthood and hence can help protect them from miR-8 induced differentiation during the pupal phases when both are co-expressed. However, the pMPs remain sensitive to forced miR-8 expression in the adult, suggesting the levels of Zfh1 are finely tuned by the expression of both zfh1-long and zfh1-short. This could be important to enable the differentiation of the MP progeny. Furthermore, the fact that Notch activity strongly promotes zfh1-short expression could explain how an elevated level of Notch signaling promotes expansion of pMPs following injury, as observed by others (Chaturvedi et al., 2017).
Together the data suggest a novel molecular logic to explain the maintenance of Drosophila satellite-like cells. This relies on the expression of zfh1-short, which, by being insensitive to miR-8 regulation, can sustain Zfh1 protein production to protect pMPs from differentiation (Figure 9). It also implies that Notch preferentially promotes the expression of a specific RNA isoform, most likely through the use of an alternate promoter in zfh1. Both of these concepts have widespread implications.
Alternate use of 3’UTRs, to escape miRNA regulation, is potentially an important mechanism to tune developmental decisions. Some tissues have a global tendency to favor certain isoform types, for example, distal polyadenylation sites are preferred in neuronal tissues (Zhang et al., 2005). Furthermore, the occurrence of alternate 3’UTR RNA isoforms is widespread (>50% human genes generate alternate 3’UTR isoforms) and many conserved miR target sites are contained in such alternate 3’UTRs (Tian and Manley, 2013; Sandberg et al., 2008). Thus, similar isoform switching may underpin many instances of progenitor regulation and cell fate determination. Indeed an isoform switch appears to underlie variations in Pax3 expression levels between two different populations of muscle satellite cells in mice, where the use of alternative polyadenylation sites resulted in transcripts with shorter 3’UTRs that are resistant to regulation by miR-206 (Boutet et al., 2012). The selection of alternate 3’-UTRs could ensure that protein levels do not fall below a critical level (Yatsenko et al., 2014), and in this way prevent differentiation from being triggered.
The switch in zfh1 RNA isoforms is associated with Notch-dependent maintenance of the persistent adult MPs. Notably, zfh1-short is generated from an alternate promoter, as well as having a truncated 3’UTR, which may be one factor underlying this switch. Studies in yeasts demonstrate that looping occurs between promoters and polyadenylation sites, and that specific factors recruited at promoters can influence poly-A site selection (Lamas-Maceiras et al., 2016; Tian and Manley, 2013). The levels and speed of transcription also appear to influence polyA site selection (Proudfoot, 2016; Tian and Manley, 2013; Pinto et al., 2011). If Notch mediated activation via Enh3 favors initiation at the zfh1-short promoter, this could in turn influence the selection of the proximal adenylation site to generate the truncated miR-8 insensitive UTR. The concept that signaling can differentially regulate RNA sub-types has so far been little explored but our results suggest that is potentially of considerable significance. In future it will be important to investigate the extent that this mechanism is deployed in other contexts where signaling coordinates cell fate choices and stem cell maintenance.
Materials and methods
Reagent type (species) or resource | Designation | Source or reference | Identifiers | Additional information |
---|---|---|---|---|
Gene (D. melanogaster) | zfh1 | NA | FLYB:FBgn0004606 | |
Gene (D. melanogaster) | Notch | NA | FLYB:FBgn0004647 | |
Gene (D. melanogaster) | miR-8 | NA | FLYB:FBgn0262432 | |
Genetic reagent (D. melanogaster) | Enh3-Gal4 | Janelia Research Campus | BDSC: 49924, FLYB: FBtp0059625 | FlyBase symbol: P{GMR35H09-GAL4}attP2 |
Genetic reagent (D. melanogaster) | zfh1 RNAi (kk 103205) | Vienna Drosophila RNAi Center | VDRC: 103205 | |
Genetic reagent (D. melanogaster) | miR-8-Gal4 | Kyoto Stock Center | DGRC: 104917 | Genotype: y[*] w[*]; P{w[+mW.hs]=GawB}NP5247/CyO, P{w[-]=UAS lacZ.UW14}UW14 |
Genetic reagent (D. melanogaster) | UAS-miR-8-Sp | Bloomington Stock Center | BDSC: 61374, FLYB: FBst0061374 | Genotype: P{UAS-mCherry.mir-8.sponge.V2}attP40/CyO; P{UAS-mCherry.mir-8.sponge.V2}attP2 |
Genetic reagent (D. melanogaster) | Enh3-GFP | This paper | ||
Genetic reagent (D. melanogaster) | ∆Enh3 | This paper | ||
Genetic reagent (D. melanogaster) | UAS-zfh1-short | This paper | UAS-Zfh1-short construct provided by BDGP, Clone # UF5607 | |
Genetic reagent (D. melanogaster) | Notch[NRE]-GFP | Sarah Bray (Cambridge, UK) | ||
Antibody | anti-Zfh1 | Ruth Lehmann (New York, USA) | ||
Antibody | anti-Mef2 | Eileen Furlong (Heidelberg, Germany) | ||
Recombinant DNA reagent | pCFD4 | Addgene | Addgene # 49411 | |
Recombinant DNA reagent | pDsRed-attP | Addgene | Addgene # 51019 |
Drosophila genetics
Request a detailed protocolAll Drosophila melanogaster stocks were grown on standard medium at 25°C. The following stains were used: w118 as wild type (wt), UAS-white-RNAi as control for RNAi experiments (BL35573), UAS-zfh1-RNAi (VDRC: KK103205, TRiP: BL29347), zfh1 deficiency (BL7917), Mef2-Gal4 (Ranganayakulu et al., 1996), UAS-Mef2 (Cripps et al., 2004), UAS-G-Trace (BL28281), UAS-Notch-RNAi (BL7078), Notch[NRE]-GFP (Simón et al., 2014), UAS-Notch∆ECD (Chanet et al., 2009; Fortini and Artavanis-Tsakonas, 1993; Rebay et al., 1993), miR-8-Gal4 (Karres et al., 2007), UAS-miR-8 (Vallejo et al., 2011), UAS-miR-8-Sp (BL61374 and Fulga et al., 2015), UAS-Scramble-SP (BL61501), UAS-mCD8::GFP (BL5137), UAS-GFPnls (BL65402), UAS-Src::GFP (Kaltschmidt et al., 2000), MHC-lacZ (Hess et al., 2007), 1151-Gal4 (Anant et al., 1998), miR-8-sensor-EGFP (Kennell et al., 2012), CG9650-LacZ (Ahmad et al., 2014), UAS-Reaper (BL5824). Enhancer-Gal4 lines described in Figure 2 and Figure 2—figure supplement 1 are either from Janelia FlyLight (http://flweb.janelia.org) or Vienna Tiles Library (http://stockcenter.vdrc.at/control/main).
RNAi experiments were conducted at 29°C. For adult specific manipulations in pMPs tubGal80ts (McGuire et al., 2003) was used to limit Enh3-Gal4 expression to a defined period of time. Crosses were kept at 18°C and eclosed adults were shifted to 29°C until dissection.
Immunohistochemistry and in situ hybridization
Request a detailed protocolImmunofluorescence stainings of wing discs were performed using standard techniques. Dissection and staining of the pupal muscles was performed according to (Weitkunat and Schnorrer, 2014). Adult muscles were prepared and stained as described in (Hunt and Demontis, 2013). The following primary antibody were used: Rabbit anti-Zfh1 (1:5000, a gift from Ruth Lehmann, New York, USA), Mouse anti-Cut (1:20, DSHB), Rabbit anti-β3-Tubulin (1:5000, a gift from Renate Renkawitz-Pohl, Marburg, Germany), Rat anti-Tropomyosin (1:1000, Abcam, ab50567), Goat anti-GFP (1:200, Abcam, ab6673), Rabbit anti-Ds-Red (1:25; Clontech, 6324496), Rabbit anti-Mef2 (1:200, a gift from Eileen Furlong, Heidelberg, Germany), Mouse anti-P1 (1:20, a gift from István Andó, Szeged, Hugary), Mouse anti-pH3 (1:100, Cell Signaling Technology, #9706), Mouse anti-β-Gal (1:1000, Promega, Z378A), Alexa-conjugated Phalloidin (1:200, Thermo fisher, Waltham/Massachusetts), Rat anti-Dcad2 (1:200, DSHB). In situ experiments were carried out according to Stellaris-protocols (https://www.biosearchtech.com/assets/bti_custom_stellaris_drosophila_protocol.pdf). Antibodies were included to the overnight hybridization step (together with the probes). zfh1 probes were generated by Bioresearch Technologies. The sequence used for zfh1-short probe span 393 bp of the first zfh1-RA exon, for zfh1-long probe, the sequence of the third exon (711 bp) common to both zfh1-RB and zfh1-RE was used (see Figure 5).
Construction of transgenic lines and mutagenesis
Request a detailed protocolFor Enh3-GFP reporter line, the genomic region chr3R: 30774595..30778415 (Enh3/GMR35H09) according to Flybase genome release r6.03 was amplified using yw genomic DNA as template. Enh3 fragment was then cloned into the pGreenRabbit vector (Housden et al., 2012). For Enh3[mut]-GFP line, two Su(H) biding sites were predicted within Enh3 sequence using Patser (Hertz and Stormo, 1999) and mutated by PCR based approach with primers overlapping the Su(H) sites to be mutated with the following sequence modifications: Su(H)1 AGTGGGAA to AGGTGTGA and Su(H) 2 TTCTCACA to TGTTTGCA. Both constructs were inserted into an AttP located at 68A4 on chromosome III by injection into nos-phiC31-NLS; attP2 embryos (Bischof et al., 2007). The UAS-zfh1-short transgenic line (Figure 7—figure supplement 1) was similarly generated using phiC mediated integration of an AttB plasmid carrying UAS-zfh1-short (BDGP Clone # UF5607) into an AttP site at position 25C7. The transgene produced detectable nuclear Zfh1 protein when crossed to Gal4 driver lines (data not shown). All constructs were fully sequenced and analyzed prior to injection.
CRISPR/Cas9 genome editing
Request a detailed protocolCRISPR mediated deletion of Enh3 was performed according to (Port et al., 2014). For generating guide RNAs, two protospacers were selected (sgRNA1 GCATTCCGCAGGTTTAGTCAC and sgRNA2 GCGATAACCCGGCGACCTCC) flanking 5’ and 3’ Enhancer-3 regions, (http://www.flyrnai.org/crispr/). The protospacers were cloned into the tandem guide RNA expression vector pCFD4 (Addgene #49411) (http://www.crisprflydesign.org/wp-content/uploads/2014/06/Cloning-with-pCFD4.pdf). For the homology directed repair step, two homology arms were amplified using yw genomic DNA as template with the following primers (Homology arm1: Fwd. 5’ GCGCGAATTCGGGCTAAACGCCAGATAAGCG 3’ Rev. 5’ TTCCGCGGCCGCCACTGGATTCCACGGCTTTTCG 3’– Homology arm 2: Fwd. 5’ GGTAGCTCTTCTTATATAACCCGGCGACCTCCTCG3’- Rev. 5’GGTAGCTCTTCTGACC GGACGAAAAACTAGCGACC) and cloned into the pDsRed-attP (Addgene #51019) vector (http://flycrispr.molbio.wisc.edu/protocols/pHD-DsRed-attP). Both constructs were injected into nos-Cas9 (BL54591) embryos. Flies with ∆Enh3 were identified via the expression of the Ds-Red in the eyes and confirmed with sequencing of PCR fragment spanning the deletion. ∆Enh3 flies were then crossed to strains carrying a deletion (BL7917), which removes zfh1. None of the tranheterozygote animals survived to adults confirming that ∆Enh3 lethality maps to the zfh1 locus.
Microscopy and data analysis
Request a detailed protocolSamples were imaged on Leica SP2 or TCS SP8 microscopes (CAIC, University of Cambridge) at 20X or 40X magnification and 1024/1024 pixel resolution. Images were processed with Image J and assembled with Adobe Illustrator. Quantification of fluorescence signal intensities was performed with Image J software. In each case the n refers to the number of individual specimens analyzed, which were from two or more independent experiments. For experiments to compare and measure expression levels, samples were prepared and analyzed in parallel, with identical conditions and the same laser parameters used for image acquisition. For each confocal stack a Sum slices projections was generated. Signal intensities were obtained by manually outlining the regions of interest, based on expression of markers, and measuring the average within each region. The values were then normalized to similar background measurements for each sample. In Figure 8 the number of transcriptional zfh1-short dots was counted manually with Image J and normalized to the total number of nuclei (Zfh1 staining), which was determined by a Matlab homemade script. Graphs and statistical analysis were performed with Prism seven using unpaired t-test. Error bars indicate standard error of the mean. Further statistical details of experiments can be found in the figure legends.
Quantitative RT PCR
Request a detailed protocol30 Wing Imaginal discs from each genotype were dissected and RNA isolated using TRIzol (Life technologies). Quantitative PCR were performed as described (Krejcí and Bray, 2007). Values were normalized to the level of Rpl32. The following primers were used. Rpl32, Fwd 5’-ATGCTAAGCTGTCGCACAAATG-3’ and Rev 5’-GTTCGATCCGTAACCGATGT-3’. zfh1 Fwd 5’- GTTCAAGCACCACCTCAAGGAG-3’ and Rev 5’- CTTCTTGGAGGTCATGTGGGAGG-3’. (Product common to all three zfh1 isoforms).
Data availability
All data generated or analysed during this study are included in the manuscript and supporting files.
References
-
Twist and Notch negatively regulate adult muscle differentiation in DrosophilaDevelopment 125:1361–1369.
-
Cells with persistent twist expression are the embryonic precursors of adult muscles in DrosophilaDevelopment 113:79–89.
-
The miR-200 family and its targets regulate type II cell differentiation in human fetal lungJournal of Biological Chemistry 290:22409–22422.https://doi.org/10.1074/jbc.M114.636068
-
Satellite cells: the architects of skeletal muscleCurrent Topics in Developmental Biology 107:161–181.https://doi.org/10.1016/B978-0-12-416022-4.00006-8
-
G-TRACE: rapid Gal4-based cell lineage analysis in DrosophilaNature Methods 6:603–605.https://doi.org/10.1038/nmeth.1356
-
Development of the indirect flight muscles of DrosophilaDevelopment 113:67–77.
-
Muscle stem cells and model systems for their investigationDevelopmental Dynamics 236:3332–3342.https://doi.org/10.1002/dvdy.21345
-
Transcriptional regulation of the Drosophila melanogaster muscle myosin heavy-chain geneGene Expression Patterns : GEP 7:413–422.https://doi.org/10.1016/j.modgep.2006.11.007
-
Whole-mount immunostaining of Drosophila skeletal muscleNature Protocols 8:2496–2501.https://doi.org/10.1038/nprot.2013.156
-
The microRNA miR-8 is a positive regulator of pigmentation and eclosion in DrosophilaDevelopmental Dynamics 241:161–168.https://doi.org/10.1002/dvdy.23705
-
Notch activation stimulates transient and selective binding of Su(H)/CSL to target enhancersGenes & Development 21:1322–1327.https://doi.org/10.1101/gad.424607
-
Promoter-Terminator gene loops affect alternative 3'-end processing in yeastJournal of Biological Chemistry 291:8960–8968.https://doi.org/10.1074/jbc.M115.687491
-
Distinct contextual roles for Notch signalling in skeletal muscle stem cellsBMC Developmental Biology 14:2.https://doi.org/10.1186/1471-213X-14-2
-
RNA polymerase II kinetics in polo polyadenylation signal selectionThe EMBO Journal 30:2431–2444.https://doi.org/10.1038/emboj.2011.156
-
zfh-1, the Drosophila homologue of ZEB, is a transcriptional repressor that regulates somatic myogenesisMolecular and Cellular Biology 19:7255–7263.https://doi.org/10.1128/MCB.19.10.7255
-
Myoblast diversification and ectodermal signaling in DrosophilaDevelopmental Cell 1:829–839.https://doi.org/10.1016/S1534-5807(01)00089-2
-
Alternative cleavage and polyadenylation: the long and short of itTrends in Biochemical Sciences 38:312–320.https://doi.org/10.1016/j.tibs.2013.03.005
-
The role of the ZEB family of transcription factors in development and diseaseCellular and Molecular Life Sciences 66:773–787.https://doi.org/10.1007/s00018-008-8465-8
-
The regulatory role of MicroRNAs in EMT and cancerJournal of Oncology 2015:865816.https://doi.org/10.1155/2015/865816
Article and author information
Author details
Funding
Medical Research Council (MRL007177/1)
- Sarah Bray
European Molecular Biology Organization (ALTF-325-2013)
- Hadi Boukhatmi
The funders had no role in study design, data collection and interpretation, or the decision to submit the work for publication.
Acknowledgements
We acknowledge the Bloomington Stock Center, the VDRC Stock Center and the Developmental Studies Hybridoma Bank for Drosophila strains and antibodies. We thank Ruth Lehmann, Renate Renkawitz-Pohl, Eileen Furlong and István Andó for antibodies. We thank Eva Zacharioudaki, Alain Vincent and Michalis Averof for critical reading of the manuscript and other members of SJB lab for valuable discussion; and we are grateful to Hannah Green and Juanjo Perez-Moreno for advice on muscle preparations. This work was funded by a program grant from the MRC to SJB and by an EMBO Long Term Fellowship for HB.
Copyright
© 2018, Boukhatmi et al.
This article is distributed under the terms of the Creative Commons Attribution License, which permits unrestricted use and redistribution provided that the original author and source are credited.
Metrics
-
- 4,423
- views
-
- 592
- downloads
-
- 43
- citations
Views, downloads and citations are aggregated across all versions of this paper published by eLife.
Citations by DOI
-
- 43
- citations for umbrella DOI https://doi.org/10.7554/eLife.35954