EFHC1, implicated in juvenile myoclonic epilepsy, functions at the cilium and synapse to modulate dopamine signaling
Abstract
Neurons throughout the mammalian brain possess non-motile cilia, organelles with varied functions in sensory physiology and cellular signaling. Yet, the roles of cilia in these neurons are poorly understood. To shed light into their functions, we studied EFHC1, an evolutionarily conserved protein required for motile cilia function and linked to a common form of inherited epilepsy in humans, juvenile myoclonic epilepsy (JME). We demonstrate that C. elegans EFHC-1 functions within specialized non-motile mechanosensory cilia, where it regulates neuronal activation and dopamine signaling. EFHC-1 also localizes at the synapse, where it further modulates dopamine signaling in cooperation with the orthologue of an R-type voltage-gated calcium channel. Our findings unveil a previously undescribed dual-regulation of neuronal excitability at sites of neuronal sensory input (cilium) and neuronal output (synapse). Such a distributed regulatory mechanism may be essential for establishing neuronal activation thresholds under physiological conditions, and when impaired, may represent a novel pathomechanism for epilepsy.
https://doi.org/10.7554/eLife.37271.001Introduction
Juvenile myoclonic epilepsy (JME) is the most common form of idiopathic epilepsy in humans, making up 10–30% of cases (Delgado-Escueta, 1984). Most genes implicated in idiopathic epilepsies encode ion channels with predictable consequences on neuronal excitability, yet disruption of EFHC1, a non-ion channel protein, has been reported as the most frequent cause of JME (Delgado-Escueta, 2007). While several neuronal roles have been proposed for EFHC1 (de Nijs et al., 2012; de Nijs et al., 2006; de Nijs et al., 2009; Rossetto et al., 2011; Suzuki et al., 2004), it remains unclear how mutations in EFHC1 lead to epilepsy. Interestingly, EFHC1 is also specifically associated with motile cilia that project from specialized cells to enable fluid flow (Conte et al., 2009; Suzuki et al., 2008; Suzuki et al., 2009), but a role for EFHC1 in non-motile cilia that emanate from most cell types to allow for sensory and signaling functions largely remains unexplored (Zhao et al., 2016).
In the human brain, there are both motile cilia, which enable cerebrospinal fluid flow, and non-motile cilia, which project from the cell bodies of all or most neurons (Bishop et al., 2007). Although EFHC1 dysfunction impairs motility of ependymal cilia in mice, the accompanying ventricle enlargement does not correlate with epilepsy (Suzuki et al., 2009). Furthermore, although several neuronal functions have been attributed to EFHC1, including regulation of ion channels, apoptosis, cell division, neuronal migration, neurite architecture and neurotransmitter release (de Nijs et al., 2012; de Nijs et al., 2006; de Nijs et al., 2009; Rossetto et al., 2011; Suzuki et al., 2004), a possible role for EFHC1 in non-motile cilia of neuronal cells has not been explored. As a core component of the protofilament ribbon structure of motile cilia thought to dictate the ordered attachment/arrangement of proteins required for motility (Ikeda et al., 2003; Linck et al., 2014), EFHC1 may play a similar role in neuronal cilia to anchor/regulate signaling molecules and modulate neuronal excitability.
Here we demonstrate that rather than being a core component of ciliary motility-associated machinery, the C. elegans orthologue of EFHC-1 is required for mechanosensation in a class of non-motile cilia, where it regulates neuronal activation and dopamine signaling. Interestingly, EFHC-1-mediated signaling also occurs at the synapse in cooperation with a known EFHC1-interaction partner, an R-type voltage-gated calcium channel. Our work highlights the importance of exaptation (functional adaptation) of a cilium motility protein in non-motile sensory cilia. Moreover, our findings reveal a previously undescribed dual-regulation of neuronal excitability at the site of sensory neuron input (cilium) and sensory neuron output (synapse) and suggest an important correspondance between dopamine neurotransmission and epilepsy.
Results
EFHC-1 localizes to cilia and synapses of mechanosensory dopaminergic neurons and to the distal regions of male-specific dopaminergic ray neurons
To isolate possible non-motile ciliary functions of EFHC1, we took advantage of Caenorhabditis elegans, a nematode which only has non-motile sensory cilia. We generated a GFP reporter of C. elegans EFHC-1 driven by its own promoter, and found that the efhc-1::gfp fusion construct is specifically expressed in a small subset of ciliated mechanosensory neurons: the dopaminergic CEP, ADE and PDE neurons, and the glutamatergic OLQ neurons (Figure 1A). Both classes of neurons terminate in cilia embedded in the cuticle, ideally positioned to mediate mechanosensation at various locations along the body: four CEP neurons paired with four OLQ neurons project cilia in the nose, two ADE neurons position cilia in the anterior body, and two PDE neurons localize cilia to the posterior body (Inglis et al., 2007; Sulston et al., 1975; White et al., 1986) (Figure 1A). Additionally, EFHC-1::GFP is found in the distal regions of sex-specific dopaminergic ray neurons in the male tail (Figure 1B, Figure 1—figure supplement 1). Importantly, these expression patterns were confirmed by tagging endogenous EFHC-1 with GFP using the CRISPR/Cas9 technique (Figure 1—figure supplement 2A–B).
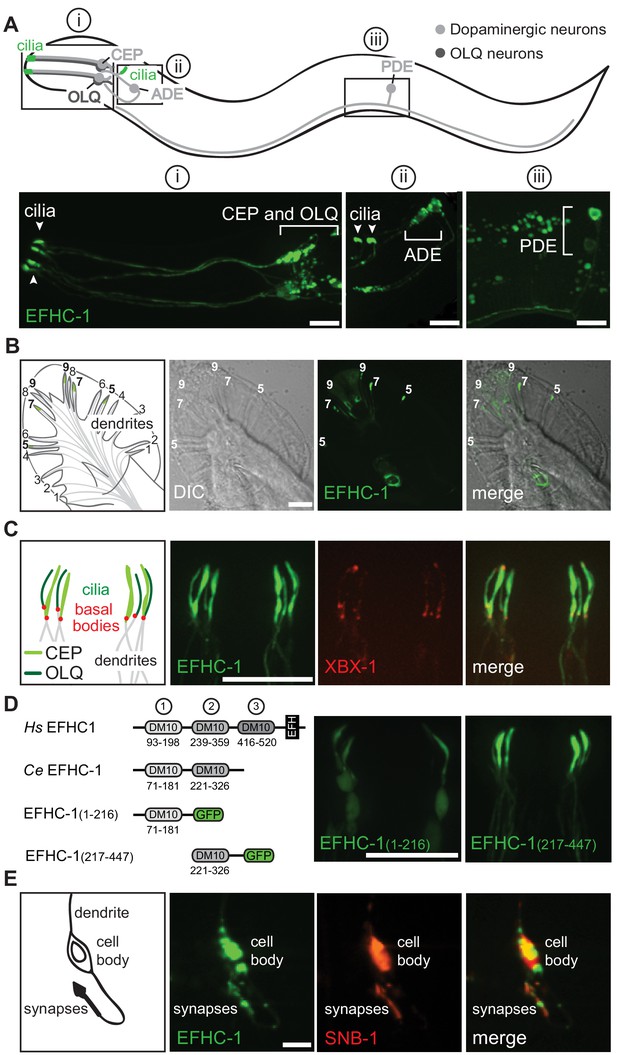
EFHC-1 localizes to cilia and synapses of mechanosensory dopaminergic neurons and to the distal regions of male-specific dopaminergic ray neurons.
(A) EFHC-1::GFP protein marker is expressed in all classes of dopaminergic neurons shared by both hermaphrodites and males (CEP, ADE and PDE), as well as in the mechanosensory OLQ neurons. Localization is enriched in cilia (arrowheads). Scale bar, 10 μm. (B) EFHC-1::GFP is also expressed in dopaminergic ray RnA neurons in the male tail (rays 5, 7 and 9) distal to dendrites. Scale bar, 10 μm. (C) In CEP and OLQ cilia, EFHC-1::GFP localization surrounds the axoneme marked by the intraflagellar transport protein XBX-1::tdTomato. Scale bar, 10 μm. (D) Human (Hs) EFHC1 is composed of three DM10 domains of unknown function and a C-terminal EF-hand (EFH) domain that is thought to bind calcium. In C. elegans (Ce), only the first two DM10 domains are conserved. A truncation protein harboring the first DM10 domain shows non-specific localization, while a truncation protein containing only the second DM10 domain shows specific ciliary localization in OLQ and CEP neurons. Scale bar, 10 μm. (E) EFHC-1::GFP also localizes to synaptic regions in CEP neurons, partially overlapping with the synaptic protein, SNB-1::tdTomato. Scale bar, 10 μm.
The ciliated dopaminergic neurons mechanically sense bacteria to modulate behaviors based on food availability (Ezcurra et al., 2011; Kindt et al., 2007a; Sanyal et al., 2004; Sawin et al., 2000), while OLQ neurons sense light touch to the nose and control the rate and bending angle of foraging (side-to-side head movements) (Hart et al., 1995; Kaplan and Horvitz, 1993). In these ciliated neurons, the generated EFHC-1::GFP fusion protein is enriched within cilia, alongside the intraflagellar transport protein (IFT) XBX-1 (DLIC orthologue) which marks ciliary basal bodies and axonemes (Figure 1C). Interestingly, while endogenously GFP-tagged EFHC-1 mostly accumulates in both OLQ and ADE cilia, it is weakly present in CEP cilia (Figure 1—figure supplement 2A), possibly reflecting a lower abundance and/or a tight incorporation with the microtubules at regular intervals within the CEP cilia that limits/masks the fluorescence associated with endogenously GFP-tagged EFHC-1. Ciliary localization of EFHC-1 is further supported by the ability of a truncated protein (C-terminal fragment containing the second of two evolutionarily conserved DM10 domains of unknown function) to specifically localize to OLQ, CEP, ADE and PDE cilia in a manner indistinguishable from the full-length protein (Figure 1D and Figure 1—figure supplement 3). This is in contrast to a second truncated protein (N-terminal fragment containing the first DM10 domain) that displays non-specific localization throughout all neuronal processes of glutamatergic (OLQ) and dopaminergic (CEP, ADE and PDE) neurons (Figure 1D and Figure 1—figure supplement 3).
Given that EFHC-1 is a core component of the protofilament ribbon structure of motile cilia that closely associates with microtubules and is ideally positioned to anchor proteins at specific locations along cilia, we hypothesized that ciliary localization of EFHC-1 is largely static. Consistent with this, when either the distal or middle portions of CEP and OLQ cilia are photobleached, the EFHC-1::GFP signal does not rapidly recover within 30 s, as would be expected for proteins dependent on diffusion to maintain their proper ciliary localizations, such as ARL-13 (Cevik et al., 2013) (Figure 1—figure supplement 4A–B). Moreover, kymograph analyses suggest that EFHC-1 proteins present in CEP and OLQ cilia are not associated with IFT (Figure 1—figure supplement 4C). EFHC-1 localization is also not dependent on UNC-101, a protein required for dendritic transport and proper ciliary localization of a set of ciliary proteins (Dwyer et al., 2001) (Figure 1—figure supplement 4D). For example, ODR-10 expressed in AWB olfactory neurons of wild-type animals predominantly accumulates in cilia, but in unc-101 mutant animals it no longer concentrates inside cilia and diffuses to dendrites, cell bodies, and axons (Figure 1—figure supplement 4D). Interestingly, ciliary expression of EFHC-1 is not dependent on DAF-19 (Figure 1—figure supplement 4E), a transcription factor required for the expression of many ciliary genes, again suggesting the unique nature of this ciliary protein, typically associated with motility, which has been retained in C. elegans to facilitate ciliary mechanosensation.
In addition to EFHC-1’s ciliary localization, we find that the EFHC-1::GFP fusion protein is present at presynaptic regions in dopaminergic neurons, partially overlapping with the synaptic vesicle protein, synaptobrevin (SNB-1) (Figure 1E). By tagging endogenous EFHC-1 with GFP, we confirm that EFHC-1 localizes to these presynaptic regions, where it partially overlaps with a second synaptic vesicle protein (RAB-3) (Figure 1—figure supplement 5A). More specifically, EFHC-1 is found to partially colocalize with the active zone protein (ELKS-1) (Figure 1—figure supplement 5B–C), where it is ideally positioned to regulate synaptic release in C. elegans. Since the presynaptic localization of synaptic vesicle proteins such as RAB-3, but not some active zone proteins like ELKS-1, depends on the kinesin-3 motor, UNC-104 (Deken et al., 2005; Nonet et al., 1997), we examined whether EFHC-1 also requires UNC-104 for its synaptic localization in CEP neurons. While RAB-3 localization is abrogated in unc-104 mutants, accumulating in cell bodies and diffusing to dendrites/axons of CEP neurons, UNC-104 is dispensable for both ELKS-1 and EFHC-1 localization (and consequently their partial colocalization pattern) to presynaptic regions (Figure 1—figure supplement 5A–C). The presence of EFHC-1 at both the cilium and presynaptic regions of dopaminergic neurons suggests that it may play important roles in both sensation and regulation of neuronal output.
EFHC-1 influences habituation to a mechanical stimulus by modulating dopamine signaling
We first investigated a possible role for EFHC-1 in dopamine signaling. In the presence of food, ciliary TRP-4 channels (orthologous to TRPN/NOMPC) are mechanically activated, resulting in the release of dopamine that modulates several behaviors through dopamine receptors (DOP-1, DOP-3 and DOP-4) on various postsynaptic/target cells (Chase et al., 2004; Ezcurra et al., 2011; Sanyal et al., 2004) (Figure 2A). One such behavior is the basal slowing response, where wild-type animals slow in the presence of food. Disrupting tyrosine hydroxylase, CAT-2, a rate-limiting enzyme for dopamine synthesis, impairs dopamine signaling and prevents slowing in the presence of food (Sawin et al., 2000) (Figure 2A). In contrast, loss-of-function efhc-1(gk424336) mutants are able to slow in the presence of food (Figure 2—figure supplement 1A), suggesting that they can release dopamine in the presence of food. Given that EFHC-1 also localizes to the distal regions of male dopaminergic neurons that are required for the coordination of locomotory events that enable male mating behvaiours (Correa et al., 2012), we assessed if efhc-1 mutants are able to release dopamine to facilitate male mating. As expected, while cat-2 mutant males show reduced mating potency due to impaired dopamine signaling, efhc-1 mutant males show mating potency similar to wild-type animals (Figure 2—figure supplement 1B). Together, these results show that efhc-1 mutants are able to release dopamine in appropriate behavioural contexts.
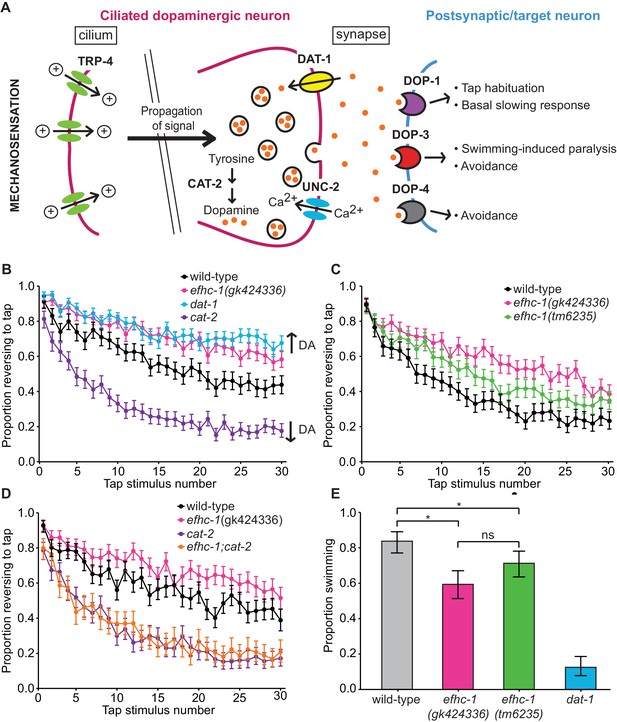
EFHC-1 influences habituation to a mechanical stimulus by modulating dopamine signaling.
(A) The molecular components of dopamine signaling in C. elegans. Food (bacteria) mechanically stimulates the ciliated dopaminergic neurons (dark pink), resulting in the release of dopamine that modulates several behaviors through dopamine receptors on various postsynaptic/target cells (blue). (B) In the presence of food, efhc-1 mutants habituate slower than wild-type animals, and similar to dat-1 mutants that have increased dopamine signaling (DA: dopamine). At each tap, the points represent the number of worms reversing over the total number of worms tracked per strain, and the error bars represent the binomial confidence intervals. Binomial logistic regression followed by Tukey’s HSD (honest significant difference) test was used to determine significance of the habituated level (proportion reversing at tap 30) for each pair of strains. All strains are statistically different from each other (p < 0.001). (C) A second allele of efhc-1, tm6235, also leads to a slow habituation phenotype that is statistically different from wild-type (p < 0.001). All strains are statistically different from each other (p < 0.001). (D) The habituation phenotype seen in efhc-1 mutants is abolished in efhc-1;cat-2 double mutants, suggesting that the slow habituation phenotype of efhc-1 mutants depends on dopamine. All strains are statistically different from each other (p < 0.001) except for cat-2 and efhc-1;cat-2 (p = 0.69). (E) efhc-1 mutants also show defects in the dopamine-dependent swimming-induced paralysis phenotype, with an intermediate level of paralysis after 10 min compared to wild-type and dat-1 mutants. Each bar represents the number of worms swimming after 10 min over the total number of worms per strain, and the error bars represent the binomial confidence intervals (n = 160 for each strain). Binomial logistic regression followed by Tukey’s HSD (honest significant difference) test was used to determine significance for each pair of strains. All strains are statistically different from each other except for the two efhc-1 strains (ns = not significant, p = 0.115). For sample sizes (n) for tap habituation experiments and complete statistical comparisons (p values) see Supplementary files 1 and 2, respectively.
To investigate the possibility that dopamine signaling may be increased in efhc-1 mutants, we assessed their behavior in tap habituation, a form of non-associative learning and memory (Rankin et al., 1990) modulated by dopamine (Kindt et al., 2007a; Sanyal et al., 2004). When animals experience a non-localized mechanical stimulus (tap), the touch receptor neurons are stimulated, and worms execute an escape response most often seen as reversals (Rankin et al., 1990) (Figure 2—figure supplement 2A). The proportion of animals responding with a reversal decreases with repeated taps (Rankin et al., 1990), and dopamine modulates this habituation (Kindt et al., 2007a; Sanyal et al., 2004). Decreased dopamine signaling in cat-2 mutants results in fast habituation, while increased dopamine signaling in mutants that lack the dopamine re-uptake transporter DAT-1 results in slow habituation (Kindt et al., 2007a; Sanyal et al., 2004) (Figure 2B). Habituation in efhc-1 mutants closely resembles that of dat-1 mutants, suggesting that abrogating EFHC-1 increases dopamine signaling (Figure 2B). A second efhc-1 allele (tm6235) lacking most of the gene also presents a slow habituation phenotype (Figure 2C), confirming that slow habituation is a result of EFHC-1 dysfunction. Furthermore, introducing the cat-2 mutation into the efhc-1 genetic background abolishes the slow habituation phenotype, resulting in fast habituation indistinguishable from cat-2 (Figure 2D). This indicates that the slow habituation phenotype in efhc-1 mutant animals requires (stems from) increased dopamine signaling.
To further investigate the dopamine signaling defect in efhc-1 mutants, we analysed their behavior in the absence of food, and found that they again show slow habituation compared to wild-type animals (Figure 2—figure supplement 2B). Interestingly, efhc-1 mutants off food mimic the habituation phenotype of wild-type animals on food, while dat-1 mutants habituate slightly slower than efhc-1 mutants off food Figure 2—figure supplement 2C). This suggests that although both mutants increase dopamine signaling, they likely do so in different ways, and to different extents. We further confirm this possibility by exploiting the dopamine-dependent swimming-induced paralysis (SWIP) phenotype seen in dat-1 mutants, which is attributed to excessive dopamine signaling (McDonald et al., 2007) (Figure 2A). Whereas most wild-type animals continue swimming 10 min after being submersed in water, most dat-1 animals are paralysed (Figure 2E), exhibiting an average thrashing frequency consistently lower than wild-type animals (Figure 2—figure supplement 2D). In this assay, both efhc-1 alleles show intermediate levels of paralysis at 10 min compared to wild-type and dat-1 mutants (Figure 2E), and corresponding intermediate thrashing frequencies (Figure 2—figure supplement 2D). Together, our data are consistent with efhc-1 mutants having increased dopamine signaling compared to wild-type animals, but less than dat-1 mutants.
EFHC-1, similar to TRP-4, is required for mechanosensation in non-motile CEP cilia
A possible explanation for the increased dopamine signaling in efhc-1 mutants is increased ciliary activation, even in the absence of food. To directly interrogate mechanosensory activation in efhc-1 mutants, we used a genetically-encoded calcium indicator (cameleon) specifically expressed in dopaminergic neurons (Kindt et al., 2007a). Two different mechanical stimuli to the nose were applied using a glass probe: a gentle stimulus consisting of vibrations to the nose for 3.7 s, expected to mimic the presence of food (buzz), and a stronger stimulus of a firm press to the nose for 1 s (press) (Kindt et al., 2007a). Wild-type animals show a calcium response to each stimulus ~50% of the time, as measured by a change in the YFP/CFP ratio (Figure 3A). In contrast, efhc-1(gk424336) mutants rarely respond upon gentle stimulation but respond at wild-type levels to the stronger stimulation (Figure 3A). Interestingly, when efhc-1 mutants do exhibit calcium responses to either stimulus, they are of a comparable amplitude and shape as wild-type responses (Figure 3B and Figure 3—figure supplement 1). This behavior is remarkably similar to that seen in animals lacking the cilium-localized mechanosensitive TRP-4 channel, which is required for food sensation (Kang et al., 2010; Kindt et al., 2007a). This suggests that like TRP-4, EFHC-1 has a specific role in mediating the likelihood of mechanosensory transduction upon gentle stimulation, as seen when an animal encounters food. In contrast, mechanosensory transduction in non-dopaminergic OLQ neurons is not impaired in efhc-1 mutants and OLQ-mediated foraging behaviors are similarly unaffected (Figure 3—figure supplement 2A–E). This implies a specific mechanosensory function for EFHC-1 in CEP dopaminergic neurons.
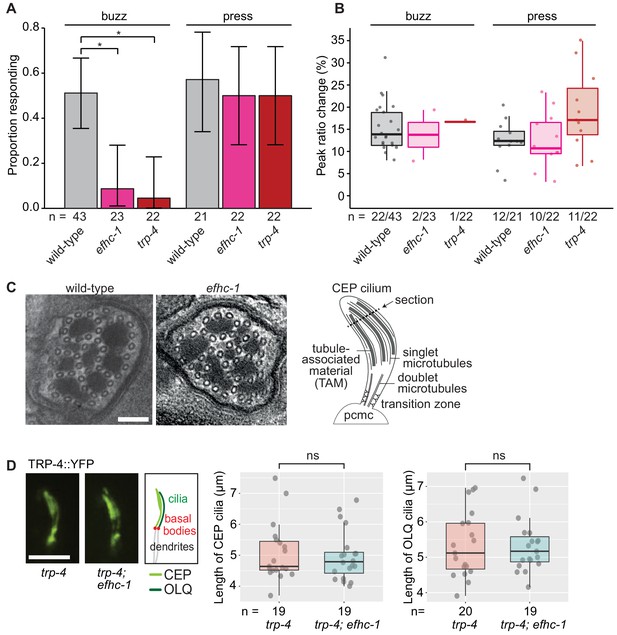
EFHC-1, similar to TRP-4, is required for mechanosensation in non-motile CEP cilia.
(A) Loss of EFHC-1 or TRP-4 impairs activation of CEP neurons upon gentle mechanical stimulation (buzz), resulting in fewer animals responding to the stimulus compared to wild-type animals. In contrast, efhc-1 or trp-4 mutants can respond to stronger stimulation (press). In this panel, n represents the total number of animals assessed. Each bar represents the number of worms responding to mechanical stimulation over the total number of worms assessed per strain, and the error bars represent the binomial confidence intervals. Binomial logistic regression followed by Tukey’s HSD (honest significant difference) test was used to determine significance for each pair of strains. Differences between all strains subjected to the press stimulus are not significant, but differences between wild-type and efhc-1 (p < 0.01) or trp-4 (p < 0.05) mutants subjected to the buzz stimulus are significant. (B) The peak ratio change (%), representing the amplitude of the neuronal response, is similar between strains for animals responding to each stimulus. In this panel, n represents the number of animals responding to the stimulus over the total number of animals assessed. (C) The axonemal structure of CEP neurons by TEM cross-section (refer to schematic) is not abrogated in efhc-1 mutants. Scale bars, 100 nm. (D) The localization of the mechanosensory channel, TRP-4, which is comparable to that of EFHC-1, is unaffected in efhc-1 mutants. Lengths of CEP and OLQ cilia measured in trp-4 and trp-4;efhc-1 mutants are indistinguishable. Each dot represents one cilium. Kruskal-Wallis rank sum test (CEP cilia lengths) and Tukey's Honest Significant Differences (OLQ cilia lengths) were used for calculating the statistical significance. ns, not significant. Scale bar, 3 μm. For complete statistical comparisons (p values) see Supplementary file 2.
Given the hypothesis that EFHC-1 helps to anchor, position, and/or regulate ciliary proteins along the ciliary axoneme in motile cilia, it is possible that it has been adapted in non-motile cilia to anchor sensory proteins such as TRP-4. We first confirmed by transmission electron microscopy (TEM) that 'conventional' cilia not harboring EFHC-1::GFP (as determined by our localization analyses) are structurally normal in efhc-1 mutants, displaying nine outer doublet microtubules that become singlets in more distal regions (Figure 3—figure supplement 3). Notably, EFHC-1-containing CEP and OLQ cilia—which have supernumerary singlet microtubules interspersed with tubule-associated material or unique arrangement of doublets in their distal regions, respectively— also appear structurally normal in efhc-1 mutants (Figure 3C and Figure 3—figure supplement 2F). Hence, EFHC-1 does not play an essential role in specifying, or maintaining, non-motile cilia ultrastructure in this metazoan. Furthermore, TRP-4::YFP localization along the axoneme of CEP cilia is retained in efhc-1 mutants (Figure 3D), suggesting that in the absence of EFHC-1, ciliary localization of TRP-4 still occurs, but its function might be perturbed. For example, EFHC-1 may be required for regulating TRP-4 activity, or anchoring/regulating other signaling molecules required for mechanosensory transduction.
EFHC-1-mediated regulation of dopamine signaling occurs, in part, downstream of cilia
Given that mechanical activation by food is required for dopamine release and that EFHC-1 is required for efficient mechanosensation, efhc-1 mutants were expected to show phenotypes consistent with decreased dopamine signaling. However, the increased dopamine signaling in efhc-1 mutants suggests that EFHC-1 may regulate dopamine release at the synapse, independent of its role in ciliary-based signal transduction. Specifically, while loss of EFHC-1 function at the cilium reduces the likelihood of mechanical activation by food, its loss at the synapse may stimulate the release of dopamine in the absence of mechanosensory stimuli and/or amplify infrequent mechanosensory stimuli. We first confirmed that synaptic regions are intact in efhc-1 mutants (Figure 4—figure supplement 1). Next, we asked if the fast habituation seen in trp-4 mutants, due to an inability to mechanically sense bacteria at the cilium and subsequently release dopamine (Figure 2A) (Kindt et al., 2007a), is affected by loss of EFHC-1 function. Interestingly, efhc-1;trp-4 double mutants show slower habituation than trp-4 mutants (Figure 4A), consistent with a rise in dopamine signaling independent of ciliary TRP-4-associated mechanosensation. In other words, loss of EFHC-1 function at the synapse may inappropriately stimulate dopamine release in the absence of TRP-4-mediated mechanosensation.
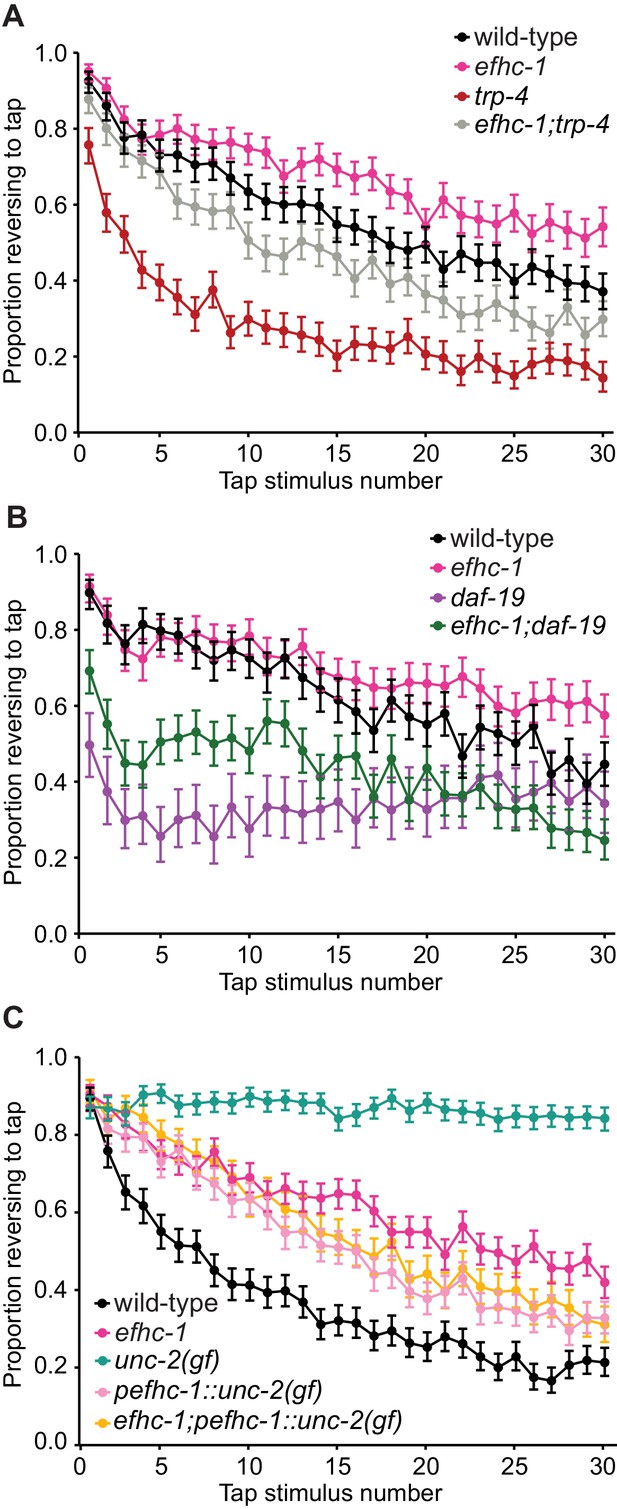
EFHC-1-mediated regulation of dopamine signaling occurs, in part, downstream of cilia.
(A) Double mutants of efhc-1 and trp-4 show intermediate habituation compared to the trp-4 and efhc-1 single mutants. At each tap, the points represent the number of worms reversing over the total number of worms tracked per strain, and the error bars represent the binomial confidence intervals. Binomial logistic regression followed by Tukey’s HSD (honest significant difference) test was used to determine significance of the habituated level (proportion reversing at tap 30) for each pair of strains. All strains are statistically different from each other (p < 0.001). (B) efhc-1 mutants with absent cilia due to a daf-19 mutation show intermediate habituation compared to daf-19 and efhc-1 single mutants. All strains are statistically different from each other (p < 0.001). (C) An unc-2(gain-of-function) mutation driven in EFHC-1-expressing cells shows slow habituation compared to wild-type animals, but faster habituation compared to animals with the unc-2(gain-of-function) mutation expressed throughout. Furthermore, efhc-1 mutants with the addition of an unc-2(gain-of-function) mutation driven in EFHC-1-expressing cells does not exacerbate the habituation phenotype. All strains are statistically different from each other (p < 0.001). For sample sizes (n) for tap habituation experiments and complete statistical comparisons (p values) see Supplementary files 1 and 2, respectively.
To confirm that EFHC-1 functions downstream of cilia, likely at the synapse, to modulate dopamine signaling, efhc-1 was combined with a daf-19 mutant lacking cilia in many sensory neurons, including dopaminergic neurons (daf-19 encodes a transcription factor required for the expression of many ciliary genes, but not efhc-1 - see Figure 1—figure supplement 4E). Interestingly, daf-19 mutants respond weakly to tap stimuli and habituate rapidly, likely due to loss or misregulation of many cilium-dependent sensory inputs required to fine-tune mechanosensation and habituation; notably, this phenotype is similar to the loss of TRP-4, which requires cilia to function (Figure 4B). In contrast, the efhc-1;daf-19 double mutants show responsiveness and habituation phenotypes that are intermediate between the daf-19 and efhc-1 single mutants (Figure 4B), again suggesting that the loss of EFHC-1 results in increased dopamine signaling downstream of ciliary function. These data, together with the localization of EFHC-1 at synaptic regions, are consistent with EFHC-1 preventing spontaneous release of dopamine at synapses in the absence of mechanical stimulation by food.
The release of dopamine at the synapse requires the activation of voltage-gated calcium channels at presynaptic termini, which allows for calcium influx and subsequent dopamine-bearing vesicle fusion (Figure 2A). In humans, EFHC1 physically interacts with and modulates the alpha subunit of the R-type voltage-gated calcium channel (Suzuki et al., 2004), which is most closely related to UNC-2 in C. elegans. We therefore hypothesized that EFHC-1 prevents spontaneous activity of UNC-2 in C. elegans dopaminergic neurons, such that loss of efhc-1 would stimulate UNC-2 activity even in the absence of mechanosensation at the cilium and result in increased dopamine release. Since unc-2 mutants are uncoordinated and cannot be tested for habituation defects, we took advantage of a gain-of-function (gf) allele that shows slow habituation compared to both wild-type animals and efhc-1 mutants (Figure 4C). Also, because unc-2 expression is found in many neurons apart from the dopaminergic neurons that may modulate tap habituation, a construct was made with UNC-2(gf) under the control of the efhc-1 promoter. Remarkably, expressing overactive UNC-2 specifically in efhc-1-expressing cells results in a slow habituation phenotype similar (but not identical) to that seen in efhc-1 mutants; crossing the UNC-2(gf) construct into efhc-1 mutants did not result in slower habituation, but instead leads to habituation similar to that seen in animals with UNC-2(gf) specifically in efhc-1-expressing cells (Figure 4C). Similarly, these trends were replicated in SWIP assays (Figure 2—figure supplement 2E). Our findings therefore provide evidence that independent of its sensory/activation role at the cilium, EFHC-1 may negatively regulate UNC-2, such that loss of EFHC-1 results in maximum activation of the voltage-gated channel at the synapse.
Discussion
Although the cilia of multicellular organisms are typically classified as either motile or non-motile, unicellular organisms possess one or more cilia responsible for both producing movement and responding to mechanosensory cues to modulate movement (Figure 5). Despite an established role for EFHC1 in ciliary motility (Conte et al., 2009; Suzuki et al., 2008; Suzuki et al., 2009), its disruption has no effect on ciliary structure (Suzuki et al., 2009), raising the possibility that it plays a role in regulating motility—for example in the mechanosensory feedback required to support normal ciliary motility. Our work in a representative eumetazoan (C. elegans) that lacks motile cilia supports this idea, where EFHC-1 has been adapted to function in a specific class of non-motile ciliated mechanosensory cells, the dopaminergic neurons (see model in Figure 5), where it is required for mechanosensation and proper dopamine signaling, yet dispensable for non-motile ciliary structure.
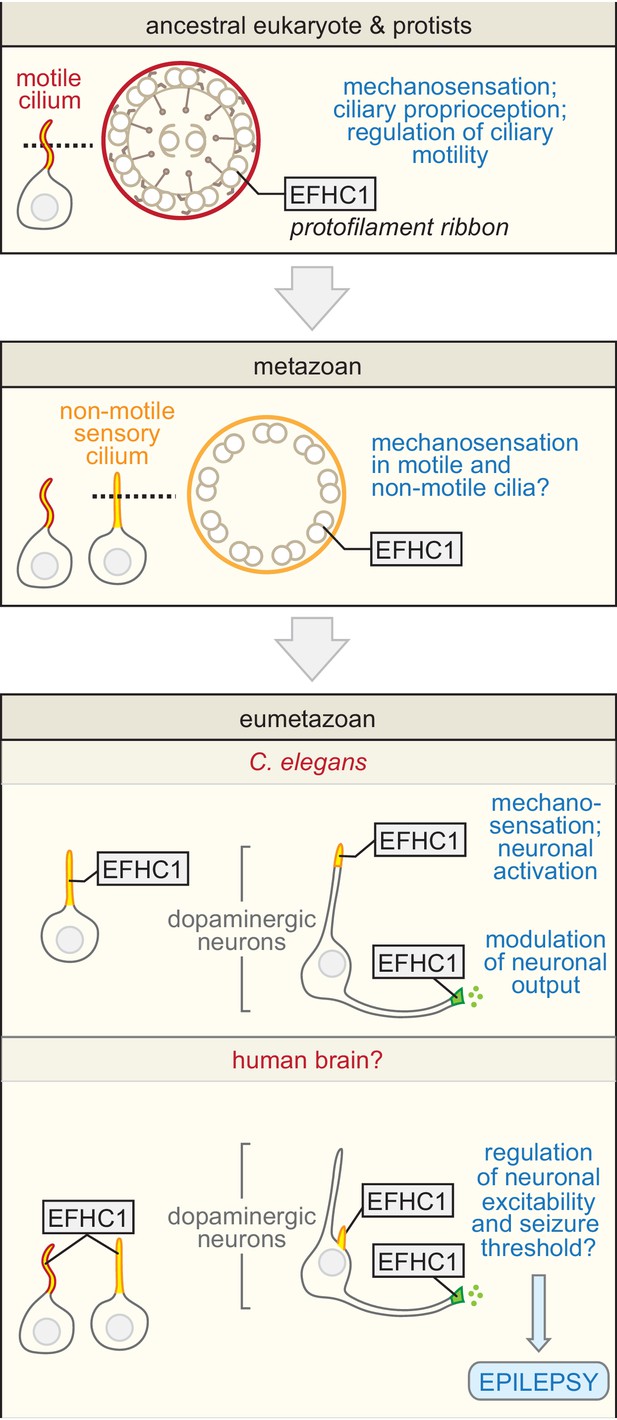
Proposed evolutionary model for the exaptation of EFHC1 motility-associated ciliary functions in non-motile cilia and synapses.
The role of EFHC1 in extant protists (and probably the ancestral eukaryote) is likely to regulate ciliary motility (Ikeda et al., 2003), potentially by acting as a mechanosensor together with a TRP family (or other) calcium channel. In metazoans, this function was retained for motile cilia (Suzuki et al., 2009), and adapted for mechanosensation and potentially other signaling roles in non-motile cilia. EFHC1 evolved in C. elegans, and potentially other neuron-bearing eumetazoans, to also regulate synaptic transmission. In C. elegans, the combined functions for EFHC-1 at cilia (mechanosensory input) and at the synapse (output) help to regulate neuronal excitability and dopamine signaling, both of which may be relevant to epilepsy in human patients with mutations in EFHC1. Motile and non-motile cilia are shown in red and orange, and synapses in green.
Our findings suggest that EFHC1, by being an integral part of the ciliary axoneme and by potentially modulating TRP (or other types of) mechanosensitive calcium channels (Katano et al., 2012) may have adapted its mechanosensation role from motile cilia (e.g., for environmental sensing and/or internal sensation and regulation of motile cilium movement) to a strictly sensory role in non-motile cilia. We also find that in C. elegans, EFHC-1 functions at the synapses of dopaminergic neurons (Figure 5). Importantly, this role is also likely related to the modulation of a calcium channel, namely UNC-2, that localizes to presynaptic active zones of sensory neurons (Saheki and Bargmann, 2009), where it may play an important role in evoked neurotransmitter release (Mathews et al., 2003; Richmond et al., 2001; Schafer and Kenyon, 1995). Together, our results make strides to reconcile the association of a non-ion channel protein with epilepsy by suggesting a possible role for EFHC-1 in modulating ion channels to fine-tune neuronal excitation/transmission both at the cilium, the site for sensory input (mechanosensation), and at the synapse, the site for sensory output that regulates the amount of neurotransmitter released.
Previous work has identified EFHC1 at both ciliary (Conte et al., 2009; Ikeda et al., 2003; Ikeda et al., 2005; Suzuki et al., 2008; Suzuki et al., 2009) and synaptic (Rossetto et al., 2011) regions, yet proposed functions at either location have only been considered separately. Our work in C. elegans provides a unique perspective given that EFHC-1-containing cells harbor both ciliary and synaptic regions, thus raising the intriguing possibility of differential trafficking to these two distinct locations within single cells. We show that EFHC-1’s ciliary localization is independent of UNC-101 and synaptic localization is independent of UNC-104, indicating that EFHC-1’s unique localization pattern does not rely on these known active transport mechanisms. While it is possible that EFHC-1’s localization pattern is dictated by other transport mechanisms, it could also result from diffusion of EFHC-1 throughout cells, followed by ciliary/synaptic retention through interactions with cytoskeletal arrangements, or specific proteins, unique to each location that enable scaffolding (e.g., microtubule-associated proteins for ciliary localization [Ikeda et al., 2005] and actin-associated proteins for synaptic localization [Torres and Inestrosa, 2018]). These possibilities warrant further attention to help better understand EFHC-1’s mechanism of action at each site.
By identifying an epilepsy-related protein that directly modulates dopamine signaling in C. elegans, our work helps to strengthen the potential link between dopamine signaling and epilepsy, where evidence is accumulating to suggest that inappropriate regulation of dopamine signaling may contribute to various forms of epilepsy (Bozzi and Borrelli, 2013). A role for dopamine is particularly relevant in JME because patients also display several deficits in cognitive functions influenced by dopamine, such as behavioral disturbances, social integration problems, impaired executive functions, reduced cognitive speed and impaired working memory (Bailet and Turk, 2000; Devinsky et al., 1997; Janz, 1985; Pascalicchio et al., 2007; Swartz et al., 1996). Interrogation of the dopaminergic system in JME patients has revealed that dopamine signaling is indeed dysregulated in JME patients (Ciumas et al., 2008; Ciumas et al., 2010; Odano et al., 2012). In combination with our work, this suggests that dopamine may play a role in the onset of seizures and/or cognitive impairments associated with JME.
In conclusion, we propose that our discovery of a dual ciliary (sensory input) - synapse (neuronal output) mechanism that regulates neuronal activity may not only apply to C. elegans dopaminergic neurons, but could be relevant to mammalian brain neurons as well, which have non-motile cilia. Indeed, dopamine receptors (DRD1, 2, 5) themselves are found on cilia, as are numerous other receptors, including for serotonin, Melanin-concentrating hormone, somatostatin, galanin, and neuropeptide (FF/YY) (Hilgendorf et al., 2016). In such neurons (and others), cilia may modulate neuronal excitation thresholds and thus play important roles in collectively influencing behavior, energy homeostasis and other aspects of mammalian physiology. More generally, our work supports the notion that the relationship between sensory structures and synapses (Kang et al., 2010) represents an important biological phenomenon that deserves further attention.
Materials and methods
Strains
All strains were grown and cultured according to standard procedures on nematode growth medium (NGM) plates with OP50 bacteria as a food source. The efhc-1(gk424336) mutant strain with a nonsense mutation approximately halfway through the gene was obtained from the Million Mutation Project (MMP) collection, and the efhc-1(tm6235) mutant strain with an in-frame deletion of exons 3–7 was obtained from the Mitani lab. Both mutant strains were backcrossed six times before being used in experiments or crosses (MX1661 efhc-1(gk424336) (6X), MX1977 efhc-1(tm6235) (6X)). The wild-type strain used in all experiments was the N2 Bristol strain obtained from the Caenorhabditis Genetics Center (CGC). Other strains used were as follows: MX081 N2;nxEx[Y49A10A.1::gfp +xbx-1::tdTomato +rol-6(su1006)]; CB1112 cat-2(e1112); RM2702 dat-1(ok157); AQ2044 ljIs103[Pcat-1::YCD3]; AQ2815 trp-4(gk341);ljIs103[Pcat-1::YCD3]; AQ2829 ljEx421[Pocr-4::YC3.60 codon optimized]; VC818 trp-4(gk341); TQ420 trp-4(sy695);xuEx542[trp-4::yfp +Podr-1::rfp]; DR86 daf-19(m86); OW47 unc-2(zf35); CX3877 lin-15(n765);kyIs156[str-1p::odr-10::gfp +lin-15(+)].
The following strains were created in this work: MX79 nxEx279[pefhc-1::efhc-1::gfp +Posm-5::xbx-1::tdTomato +rol-6(su1006)]; MX1971 efhc-1(gk424336); nxEx[pefhc-1::efhc-1 (217–447)::gfp +ccgfp]; MX2192 efhc-1(gk424336); nxEx[pefhc-1:: efhc-1 (1–216)::GFP +Posm-5::xbx-1::tdTomato +ccgfp]; MX2195 nxEx[pefhc-1::efhc-1::gfp +pefhc-1::xbx-1::tdtomato +cc::gfp]; MX2194 N2;nxEx[snb-1::tdtomato +Y49A10A.1::gfp +cc::gfp]; MX2132 efhc-1(gk424336);cat-2(e1112); MX2403 efhc-1(gk424336);ljIs103[Pcat-1::YCD3]; MX2317 efhc-1(gk424336);trp-4(sy695);xuEx542[trp-4::yfp +Podr-1::rfp]; MX2129 efhc-1(gk424336);trp-4(gk341); MX2316 efhc-1(gk424336);daf-19(m86); MX1973 daf-19(m86) II;daf-12(sa204);nxEx[efhc-1::gfp +cc::gfp]; MX2185 dpy-5(e907);nxEx[pefhc-1::unc-2(zf35) +cc::gfp +dpy-5(+)]; MX2341 efhc-1(gk424336);dpy-5(e907);nxEx[pefhc-1::unc-2(zf35) +cc::gfp +dpy-5(+)]; MX2317 efhc-1(gk424336);trp-4(sy695);xuEx542[trp-4::yfp +Podr-1::rfp]; MX2137 efhc-1(gk424336);ljEx421[Pocr-4::YC3.60 codon optimized]. MX2724 efhc-1(nx163[efhc-1::gfp::3xflag])X; MX2727 N2;egIs1[pdat-1::gfp];nxEx2714[Pdat-1::elks-1::tdtomato +rol-6(su1006)]; MX2726 efhc-1(gk424336);egIs1[pdat-1::gfp];nxEx2714[Pdat-1::elks-1::tdtomato +rol-6(su1006)]; MX2742 efhc-1(nx163[efhc-1::gfp::3xflag])X; nxEx2714[Pdat-1::elks-1::tdtomato +rol-6(su1006)]; MX2754 efhc-1(nx163[efhc-1::gfp::3xflag])X; nxEx2754[Pdat-1::elks-1::tdTomato +rol-6(su1006)]; MX2769 efhc-1(nx163[efhc-1::gfp::3xflag])X; nxEx2769 [Pdat-1::mcherry::rab-3+rol-6(su1006)]; MX2904 unc-104(e1265); efhc-1(nx163[efhc-1::gfp::3xFlag])X;nxEx2769[Pdat-1::mCherry::rab-3+rol-6(su1006)]; MX2905 unc-104(e1265); efhc-1(nx163[efhc-1::gfp::3xFlag])X; nxEx2754[Pdat-1::ELKS-1::tdTomato +rol-6(su1006)]; MX2908 unc-101(m1); efhc-1(nx163[efhc-1::gfp::3xFlag])X; nxEx2769[Pdat-1::mCherry::rab-3+rol-6(su1006)]; MX2909 unc-101(m1); lin-15(n765); kyIs156[str-1p::odr-10::gfp +lin-15(+)]
Generation and visualisation of transgenic animals
Generation of fusion constucts
Request a detailed protocolBoth the pefhc-1::XBX-1::tdTomato and pefhc-1::SNB-1::tdTomato constructs were created using 2036 bp of efhc-1’s endogenous upstream promoter fused to all the exons/introns of xbx-1 or snb-1 and then fused in-frame to tdTomato. The pefhc-1::unc-2(gf) construct was again created using 2036 bp of efhc-1’s endogenous upstream promoter fused to the cDNA of unc-2 with a gain-of-function mutation and a synthetic intron. To construct the presynaptic markers, mcherry::rab-3::unc-10 3’ UTR was cloned from a plasmid (kindly provided by the Mizumoto lab), and elks-1 was amplified from genomic DNA and stitched to the tdTomato::unc-54 3’ UTR. Both amplified PCR fragments were stitched to the dat-1 promoter (~700 bp) to drive expression in the dopaminergic neurons (CEP, ADE and PDE).
Generation of an efhc-1::gfp knock-in strain
Request a detailed protocolThe unc-119 guide RNA sequence in pU6::unc-119 sgRNA vector (Addgene #46169) was replaced with the predicted efhc-1 CRISPR guide RNA sequence (5’- GTACAGTCTAGTGGAGAGA-3’), which is present in exon 10 of efhc-1 using site-directed mutagenesis technique (http://crispr.mit.edu/) (Friedland et al., 2013). The donor template for the C-terminal GFP knock-in was prepared from pDD282 (Addgene #66823) (Dickinson et al., 2015). The pDD282 was digested with SpeI and AvrII to remove ccdB markers and mixed with upstream and downstream homology (500 ~ 650 bp) arms for efhc-1. The donor plasmid was cloned using Gibson Assembly. The GFP donor template plasmid contains T434T silent mutation in PAM site. The mixture (50 ng/μl coel::RFP (Addgene #8938), 50 ng/μl pCAS9 (Addgene #46168), 50 ng/μl efhc-1 sgRNA, and 50 ng/μl donor template) was injected into 30 adult worms. F1 worms were screened using 250 μg/ml hygromycin solution followed by picking 100% roller F2. A self-excising drug selection cassette (SEC), which contains sqt-1, hs::Cre, and hygR, was removed by heat shock at 34°C for 4 hr. This worm strain was confirmed by Sanger DNA sequencing.
Visualization of transgenic animals
Request a detailed protocolFluorescently-tagged proteins were visualized by first immobilizing animals using 0.5 μl of 10 mM levamisole and 0.5 μL of 0.1 μm diameter polystyrene microspheres (Polysciences 00876–15, 2.5% w/v suspension) on 5% agarose pads. Z-stack images were collected using a spinning disc confocal microscope (WaveFX; Quorum Technologies), a Hammamatsu 9100 EMCCD camera and Volocity software (PerkinElmer). Fluorescence recovery after photobleaching (FRAP) assay was performed using LSM880 laser scanning microscope with Airyscan and ZEN 2.3 software. Thresholded Pearson’s correlation coefficients for the colocalization of EFHC-1::GFP and ELKS-1::tdTomato in CEP axons were calculated using Volocity 6.3 software.
Mating efficiency test
Request a detailed protocolAn OP50 culture was grown at 37°C for 16 hr. To make mating plates, 20 μl of the culture was pipetted onto an NGM agar plate and the seeded plates were incubated at 25°C for 20 hr. One heat-shock generated homozygous L4 stage male (wild-type or mutant) and one L4 stage wild-type hermaphrodite were placed onto the bacterial lawn of the mating plate. All mating plates were incubated at 20°C for 4 days. The plates containing male progeny were counted as successful matings.
Behavioral analyses
All behavioral analyses were conducted on at least two separate days to account for day-to-day differences.
Tap habituation
Request a detailed protocolWorms were age synchronized by allowing five gravid adults to lay eggs for 3–4 hr on individual NGM plates seeded with E. coli OP50 bacteria and testing the worms after 4 days. At least one hour before testing, 20–30 worms were transferred to fresh NGM plates, with or without food. Plates with food were seeded with 50 μL of liquid culture OP50 bacteria in lysogeny broth (LB) 24 hr beforehand, while plates without food were seeded with 50 μL LB. Plates with worms were then placed into the Multi-Worm Tracker (MWT), and after a 300 or 600 s acclimatization period, 30 taps were administered to the side of the plate using a solenoid tapper at 10 s interstimulus intervals (ISI) [31]. The script used for tap habituation analysis is available at Loucks (2018) (copy archived at https://github.com/elifesciences-publications/dopamine_habituation). All experiments were done at 20°C, and in most experiments strains were cultured at 20°C. In the habituation experiment involving daf-19(m86), all strains were cultured at 15°C for four days then moved to 20°C for two days to enrich for non-dauer worms in daf-19 and daf-19;efhc-1 worms. The script for analysis is available at: https://github.com/cloucks/dopamine_habituation). Experiments from individual days were only included if at least 80% of wild-type animals responded to the initial tap, indicating that laboratory conditions (temperature, age and humidity) were appropriate for the subsequent analyses. Given that the MWT does not track every animal on a plate at a given time, minimum sample sizes for a single tap in a given experiment are presented in Supplementary file 1.
Swimming-induced paralysis (SWIP)
Request a detailed protocolWorms were age synchronized by allowing 10 gravid adults to lay eggs for 3 hr on individual NGM plates seeded with OP50 bacteria. L4 worms were tested after 2 days at 20°C. Two different SWIP assays were performed: one manual and one automated. For both assays, ten L4 animals were placed in 30 μL of tap water in a single well of a Pyrex Spot Plate (Fisher). For the manual assay, animals were assessed for paralysis at 10 min, defined as no C-shaped bends for at least 5 s. For the automated assay, the plate was analysed using the Multi-worm tracker (MWT) to assess thrashing frequency of animals over 11 min (script available at: https://github.com/cloucks/dopamine_swimming).
Basal slowing response
Request a detailed protocolWorms were age synchronized by allowing five gravid adults to lay eggs for 3–4 hr on individual NGM plates seeded with OP50 bacteria and testing the worms after 3 days. On the day of testing, worms were washed off their individual plates with M9 buffer and transferred to individual NGM with or without food. Plates with food were seeded with 100 µL HB101 24 hr beforehand, while plates without food were seeded with 100 μL LB. Plates with worms were then placed into the Multi-Worm Tracker (MWT) (Swierczek et al., 2011) for 600 s. Body bends were counted for animals persisting from 60 to 120 s.
Suppression of foraging
Request a detailed protocolSuppression of foraging was performed as described previously (Kindt et al., 2007b; Loucks et al., 2016).
Single worm tracking behavioral assessment
Request a detailed protocolSingle-worm tracking was performed as described previously (Loucks et al., 2016; Yemini et al., 2013).
Calcium imaging of CEP and OLQ neurons
Request a detailed protocolCalcium imaging of nose touch stimulation of glued animals was performed using identical protocols to our previous work (Loucks et al., 2016), which was essentially as described previously, using a 1 s ‘press’ or 3.7 s ‘buzz’ stimulus (Kindt et al., 2007a; Kindt et al., 2007b). Images were recorded at 10 Hz using an iXon EM camera (Andor Technology), captured using IQ1.9 software (Andor Technology) and analysed using a Matlab (MathWorks) program custom written by Ithai Rabinowitch. A rectangular region of interest (ROI) was drawn around the cell body and for every frame the ROI was shifted according to the new position of the centre of mass. Fluorescence intensity, F, was computed as the difference between the sum of pixel intensities and the faintest 10% pixels (background) within the ROI. Fluorescence ratio R = Fyellow/Fcyan (after correcting for bleed through) was used for computing ratio change, expressed as a percentage of R0 (the average R within the first 3 s of recording) (Rabinowitch et al., 2013).
Transmission electron microscopy (TEM)
Request a detailed protocolDay one adult worms were fixed (glutaraldehyde), embedded, serial sectioned and imaged as previously described (Sanders et al., 2015). The one exception is the wild type image in Figure 3C, which was obtained from sections of adult day one worms fixed and embedded using high pressure freezing (Leica EMPACT2) and freeze substititution (FS), as previously described (Cohen et al., 2008). The FS step was performed using a solid metal block placed at −75°C for 48 hr, followed by −20°C for 24 hr, and then room temperature for 3 hr.
Statistical tests
Request a detailed protocolLogistic regression was used for the statistical analyses performed because the dependent variables (proportion reversing to tap, proportion swimming and proportion responding) are not continuous but instead represent binary outcomes (e.g. reversing or not reversing). Futhermore, our data meet the assumptions required for logistic regression, including the independence of sample outcomes, an absence of strongly influential outliers and a sufficient number of recorded events (Stoltzfus, 2011). Sample sizes for each behavioral assay were chosen to be either equal to or greater than sample sizes reported in the literature that were sufficient to detect biologically relevant differences.
Data availability
All data (including data generated during the revision process) have been deposited in Dryad, 10.5061/dryad.bb46h5s.
-
Dryad Digital RepositoryData from: EFHC1, implicated in juvenile myoclonic epilepsy, functions at the cilium and synapse to modulate dopamine signaling.https://doi.org/10.5061/dryad.bb46h5s
References
-
Type III adenylyl cyclase localizes to primary cilia throughout the adult mouse brainThe Journal of Comparative Neurology 505:562–571.https://doi.org/10.1002/cne.21510
-
The role of dopamine signaling in epileptogenesisFrontiers in Cellular Neuroscience 7:e157.https://doi.org/10.3389/fncel.2013.00157
-
Mechanism of extrasynaptic dopamine signaling in caenorhabditis elegansNature Neuroscience 7:1096–1103.https://doi.org/10.1038/nn1316
-
Electron microscopy of lamin and the nuclear lamina in caenorhabditis elegansMethods in Cell Biology 88:411–429.https://doi.org/10.1016/S0091-679X(08)00421-4
-
Expression profile and distribution of Efhc1 gene transcript during rodent brain developmentJournal of Molecular Neuroscience 39:69–77.https://doi.org/10.1007/s12031-009-9179-6
-
EFHC1, a protein mutated in juvenile myoclonic epilepsy, associates with the mitotic spindle through its N-terminusExperimental Cell Research 312:2872–2879.https://doi.org/10.1016/j.yexcr.2006.05.011
-
EFHC1 interacts with microtubules to regulate cell division and cortical developmentNature Neuroscience 12:1266–1274.https://doi.org/10.1038/nn.2390
-
Redundant localization mechanisms of RIM and ELKS in caenorhabditis elegansJournal of Neuroscience 25:5975–5983.https://doi.org/10.1523/JNEUROSCI.0804-05.2005
-
Summation of the workshops and discussion: the new wave of research in the epilepsiesAnnals of Neurology 16:S145–S158.https://doi.org/10.1002/ana.410160721
-
Frontal functions in juvenile myoclonic epilepsyNeuropsychiatry, Neuropsychology, and Behavioral Neurology 10:243–246.
-
Heritable genome editing in C. elegans via a CRISPR-Cas9 systemNature Methods 10:741–743.https://doi.org/10.1038/nmeth.2532
-
The primary cilium as a cellular receiver: organizing ciliary GPCR signalingCurrent Opinion in Cell Biology 39:84–92.https://doi.org/10.1016/j.ceb.2016.02.008
-
The sensory cilia of caenorhabditis elegansWormBook Online Rev. C Elegans Biol pp. 1–22.
-
Epilepsy with impulsive petit mal (juvenile myoclonic epilepsy)Acta Neurologica Scandinavica 72:449–459.https://doi.org/10.1111/j.1600-0404.1985.tb00900.x
-
Caenorhabditis Elegans TRPA-1 functions in mechanosensationNature Neuroscience 10:568–577.https://doi.org/10.1038/nn1886
-
Insights into the structure and function of ciliary and flagellar doublet microtubules: tektins, Ca2+-binding proteins, and stable protofilamentsThe Journal of Biological Chemistry 289:17427–17444.https://doi.org/10.1074/jbc.M114.568949
-
PACRG, a protein linked to ciliary motility, mediates cellular signalingMolecular Biology of the Cell 27:2133–2144.https://doi.org/10.1091/mbc.E15-07-0490
-
Caenorhabditis Elegans rab-3 mutant synapses exhibit impaired function and are partially depleted of vesiclesThe Journal of Neuroscience 17:8061–8073.https://doi.org/10.1523/JNEUROSCI.17-21-08061.1997
-
Caenorhabditis Elegans: a new model system for the study of learning and memoryBehavioural Brain Research 37:89–92.https://doi.org/10.1016/0166-4328(90)90074-O
-
Presynaptic CaV2 calcium channel traffic requires CALF-1 and the alpha(2)delta subunit UNC-36Nature Neuroscience 12:1257–1265.https://doi.org/10.1038/nn.2383
-
Logistic regression: a brief primerAcademic Emergency Medicine 18:1099–1104.https://doi.org/10.1111/j.1553-2712.2011.01185.x
-
Dopaminergic neurons in the nematode caenorhabditis elegansThe Journal of Comparative Neurology 163:215–226.https://doi.org/10.1002/cne.901630207
-
Mutations in EFHC1 cause juvenile myoclonic epilepsyNature Genetics 36:842–849.https://doi.org/10.1038/ng1393
-
Sequential expression of Efhc1/myoclonin1 in choroid plexus and ependymal cell ciliaBiochemical and Biophysical Research Communications 367:226–233.https://doi.org/10.1016/j.bbrc.2007.12.126
-
Efhc1 deficiency causes spontaneous myoclonus and increased seizure susceptibilityHuman Molecular Genetics 18:1099–1109.https://doi.org/10.1093/hmg/ddp006
-
High-throughput behavioral analysis in C. elegansNature Methods 8:592–598.https://doi.org/10.1038/nmeth.1625
-
The structure of the nervous system of the nematode caenorhabditis elegansPhilosophical Transactions of the Royal Society B: Biological Sciences 314:1–340.https://doi.org/10.1098/rstb.1986.0056
-
A database of caenorhabditis elegans behavioral phenotypesNature Methods 10:877–879.https://doi.org/10.1038/nmeth.2560
Article and author information
Author details
Funding
Canadian Institutes of Health Research (MOP82870)
- Michel R Leroux
Michael Smith Foundation for Health Research (Senior Scholar Award)
- Michel R Leroux
Natural Sciences and Engineering Research Council of Canada (Discover Grant 122216-2013)
- Catharine H Rankin
Science Foundation Ireland (11/PI/1037)
- Oliver E Blacque
Medical Research Council (MC_A023_5PB91)
- William Schafer
Wellcome (WT103784MA)
- William Schafer
Canadian Institutes of Health Research (MOP130287)
- Catharine H Rankin
Canadian Institutes of Health Research (Frederick Banting and Charles Best Canada Graduate Scholarship)
- Catrina M Loucks
Natural Sciences and Engineering Research Council of Canada (Vanier Canada Graduate Scholarship)
- Kwangjin Park
The funders had no role in study design, data collection and interpretation, or the decision to submit the work for publication.
Acknowledgements
Some of the nematode strains used in this work were provided by the Caenorhabditis Genetics Center (funded by the National Institutes of Health National Center for Research Resources), the Mitani lab and the Million Mutation Project (MMP) collection. We would also like to thank Dr. Mark Alkema for the unc-2(gf) strain and associated plasmid, Dr. Shawn Xu for the strain expressing TRP-4::YFP and Dr. Kota Mizumoto for the plasmid carrying mcherry::rab-3. Finally, we would like to thank Robyn Branicky for the AQ2044 strain, Ithai Rabinowitch for the AQ2815 and AQ2829 strains and Marios Chatzigeorgiou for the AQ2815 strain.
Copyright
© 2019, Loucks et al.
This article is distributed under the terms of the Creative Commons Attribution License, which permits unrestricted use and redistribution provided that the original author and source are credited.
Metrics
-
- 2,376
- views
-
- 274
- downloads
-
- 12
- citations
Views, downloads and citations are aggregated across all versions of this paper published by eLife.
Citations by DOI
-
- 12
- citations for umbrella DOI https://doi.org/10.7554/eLife.37271