Arid3a regulates nephric tubule regeneration via evolutionarily conserved regeneration signal-response enhancers
Abstract
Amphibians and fish have the ability to regenerate numerous tissues, whereas mammals have a limited regenerative capacity. Despite numerous developmental genes becoming reactivated during regeneration, an extensive analysis is yet to be performed on whether highly regenerative animals utilize unique cis-regulatory elements for the reactivation of genes during regeneration and how such cis-regulatory elements become activated. Here, we screened regeneration signal-response enhancers at the lhx1 locus using Xenopus and found that the noncoding elements conserved from fish to human function as enhancers in the regenerating nephric tubules. A DNA-binding motif of Arid3a, a component of H3K9me3 demethylases, was commonly found in RSREs. Arid3a binds to RSREs and reduces the H3K9me3 levels. It promotes cell cycle progression and causes the outgrowth of nephric tubules, whereas the conditional knockdown of arid3a using photo-morpholino inhibits regeneration. These results suggest that Arid3a contributes to the regeneration of nephric tubules by decreasing H3K9me3 on RSREs.
https://doi.org/10.7554/eLife.43186.001Introduction
Kidneys are necessary for the maintenance of homeostasis in vertebrates. The functional loss of this organ leads to severe defects in systems vital to all vertebrates, including human. During evolution, vertebrates evolved the following three complex kidney structures: pronephros, mesonephros, and metanephros. Metanephros is the adult kidney in higher vertebrates, such as humans and mice, whereas mesonephros is the adult kidney in fish and amphibians. Pronephros is the simplest and earliest kidney form (Jones, 2005; Desgrange and Cereghini, 2015). Despite the different levels of complexity of these three kidney types, their functional unit, the nephron, does not differ much (Brändli, 1999; Lienkamp, 2016). In human, there are approximately one million nephrons per kidney (Saxén and Sariola, 1987). Each nephron is composed of a glomerulus, a filtering component, and a nephric tubule, which is divided into the following four basic domains: proximal tubule, loop of Henle, distal tubule, and connecting tubule (Saxén and Sariola, 1987). According to molecular studies, the nephrons of the pronephros in Xenopus laevis can be subdivided into four distinct segments that are homologous to the segments of the metanephric nephrons of mammals (Raciti et al., 2008). Owing to these similarities, X. laevis is considered a suitable model for studying renal regeneration for application to human.
Gentamicin-induced nephropathy and partial nephrectomy showed that numerous vertebrates have the ability to repair damage to nephric structures, although this regenerative ability diverges between species (Nonclercq et al., 1992; Diep et al., 2011; Zhou et al., 2010; Caine and Mclaughlin, 2013). In mammals, nephrons contain mature tubular epithelial cells that have the capacity to regenerate following acute kidney injury (Maeshima et al., 2014). These epithelial cells rapidly lose their brush border, dedifferentiate into mesenchymal-like cells, and then migrate into regions where cells are damaged (Maeshima et al., 2014). Recent cell lineage studies have also suggested that renal stem cells and progenitor cells were found in the metanephric mesenchyme, limb of Henle’s loop, and distal convoluted tubule in mice (Kobayashi et al., 2008; Barker et al., 2012). Although mammalian nephrons possess cells that contribute to repair after injury, the regenerative capacity is restricted to the reconstruction of nephric epithelial cells in damaged regions (Maeshima et al., 2015). In contrast to mammals, which merely reconstitute tubular epithelial cells after injury, previous studies showed that X. laevis and zebrafish regenerate fully coiled and functional nephric tubule architecture after severe damage (Diep et al., 2011; Caine and Mclaughlin, 2013). In zebrafish, the transplantation of lhx1a-positive or six2 mesenchymal cells, which are considered stem cells, into adult fish in which the kidney has been injured by gentamicin reconstructs functional nephrons (Diep et al., 2011). X. laevis also regenerates the functional pronephros that can uptake albumin again after the mechanical loss of proximal tubules (Caine and Mclaughlin, 2013). Both amphibians and fish have a high regenerative capacity, but their regenerative mechanisms appear to differ. Zebrafish use kidney stem cells to repair the functional nephrons, whereas X. laevis appear to use remaining tubule cells to regenerate the functional pronephros (Diep et al., 2011; Caine and Mclaughlin, 2013). Thus, despite the different regenerative mechanisms, numerous vertebrates have the ability to repair injured nephrons.
Recently, studies have established protocols for generating the nephric structure derived from induced pluripotent cells (iPSCs) and embryonic stem (ES) cells by the combined application of the Wnt activator and other signaling factors such as BMP4 (Xia et al., 2013; Takasato et al., 2014; Takasato et al., 2015). In addition, direct reprogramming from fibroblasts into renal tubular epithelial cells, called induced renal tubular epithelial cells (iRECs), has been successfully achieved using a combination of transcription factors whose expression in the kidney is evolutionarily conserved between mice and Xenopus (Kaminski et al., 2016; Lienkamp, 2016). However, although the molecular factors for the construction of nephrons in vivo and in vitro have been identified, the molecular mechanisms that allow the reactivation of developmental genes during regeneration in highly regenerative animals remain unclear.
Genetic studies have shown that BMP and canonical Wnt and Fgf signals first specify intermediate mesoderm to form the pronephric primordium, followed by epithelialization, during kidney development (Krause et al., 2015). In addition to signaling factors, transcription factors such as Osr1, Osr2, Pax8, Hnf1b, Lhx1, and WT1 also coordinately regulate kidney formation (Bouchard, 2004; Lienkamp, 2016). These developmental genes for the kidney are evolutionarily conserved between pronephros formation in Xenopus and the more complex nephrons in mammalian meso- and metanephros (Lienkamp, 2016). During the regeneration of nephrons in zebrafish, transplanted lhx1a-positive mesenchymal cells begin to express developmental genes such as pax2a, wt1a, and fgf8a (Diep et al., 2011). In order to use developmental genes during regeneration, the genes have to be expressed again in regenerating tissues, which requires cis-regulatory elements called enhancers. Enhancers are known to determine the tissue specificity and timing of gene expression, and over 43,000 active enhancers have been identified in the human genome alone (Andersson et al., 2014). To date, numerous enhancers for tissue and organ development have been identified by the deletion mapping of upstream genomic regions and/or candidate screening using the epigenetic landscape (Kleftogiannis et al., 2016), whereas only a few enhancers for tissue regeneration have been reported, such as tissue regeneration enhancer elements for zebrafish heart reconstruction (Kang et al., 2016). Although it is generally assumed that the genome of vertebrates contains more enhancers that promote gene expression in regenerating tissues, the difficulty in identifying the in vivo function of enhancers prevents us from revealing the cis-regulatory architecture for regeneration. We have previously established an in vivo enhancer mapping system using X. laevis transgenesis (Ogino et al., 2008; Ochi et al., 2012; Suzuki et al., 2015). Here, we extended this strategy for identifying enhancers for the regeneration of nephric tubules and for examining the transcriptional mechanisms that regulate the enhancer activities during regeneration. We first found that lhx1 expression was induced within 24 hr after the surgical removal of nephric tubules. In order to explore the induction mechanisms of lhx1 in regenerating nephric tubules, we screened enhancers in genomic loci using a transgenic mapping system and found that genomic elements that are conserved from fish to human, called regeneration signal-response enhancers (RSREs), were activated in regenerating nephric tubules. A putative binding motif of Arid3a, a member of the AT-rich interaction domain family, is commonly found in RSREs for lhx1. We found that Arid3a directly binds to RSREs and Arid3a with H3K9me3 demethylases Kdm4/Jmjd2 reducing H3K9me3 levels on RSREs. We further found that the conditional knockdown of arid3a during regeneration suppressed the regeneration of nephric tubules. We now demonstrate that Arid3a is required for the regeneration of nephric tubules through the RSREs.
Results
Proximal tubules and intermediate tubules have different regenerative capacities
The pronephric kidneys of X. laevis become functional from Nieuwkoop and Faber stages 37–38. A previous study showed that proximal tubules that have been partially nephrectomized at stages 37–38 can regenerate functional nephrons (Raciti et al., 2008; Caine and Mclaughlin, 2013). Since we have previously established the Xla.Tg(Xtr.pax8:EGFP) transgenic line that can be used to trace nephric tubule formation, we first confirmed the regenerative process of nephric tubules using live imaging of stage 37 transgenic embryos (Ochi et al., 2012). Our careful nephric tubule surgery did not damage the glomus, which is situated medially to the tubules displaying podocin expression (Figure 1—figure supplement 1). When the proximal tubules were partially nephrectomized, a coiled structure appeared at around 24 hr (Figures 1A, B and E). This coiled tubule continued to extend from 24 to 48 hr, and then the regenerated tubules reconstructed the nephron between 72 and 120 hr (Figure 1B and E). In contrast, completely nephrectomized proximal tubules failed to form a coiled structure, whereas the remaining tubules, the intermediate tubules, continued to extend from the back to the front (Figure 1C and E). Conversely, when the intermediate tubules were completely removed, no tubule extension was observed (Figure 1D and E). Thus, although Xla.Tg(Xtr.pax8:EGFP) cannot capture all of the cells that contribute to the reconstruction of nephric tubules, our results indicate that the proximal tubules are essential for the regeneration of the coiled structure of the nephric duct. In addition, the intermediate tubules also have the capacity to regenerate nephric tubules, although this capacity is limited compared with that of the proximal tubules. It has been shown in a previous study that apoptotic cells and the expression of matrix metalloproteinase nine dramatically increase within 12 hr after nephrectomy, and these events continue until 24–48 hr (Caine and Mclaughlin, 2013). Our observation that regenerative tubules exhibit a coiled structure at around 24–48 hr together with previous findings suggests that the genetic mechanisms that occur within 48 hr after nephrectomy are crucial for nephron regeneration.
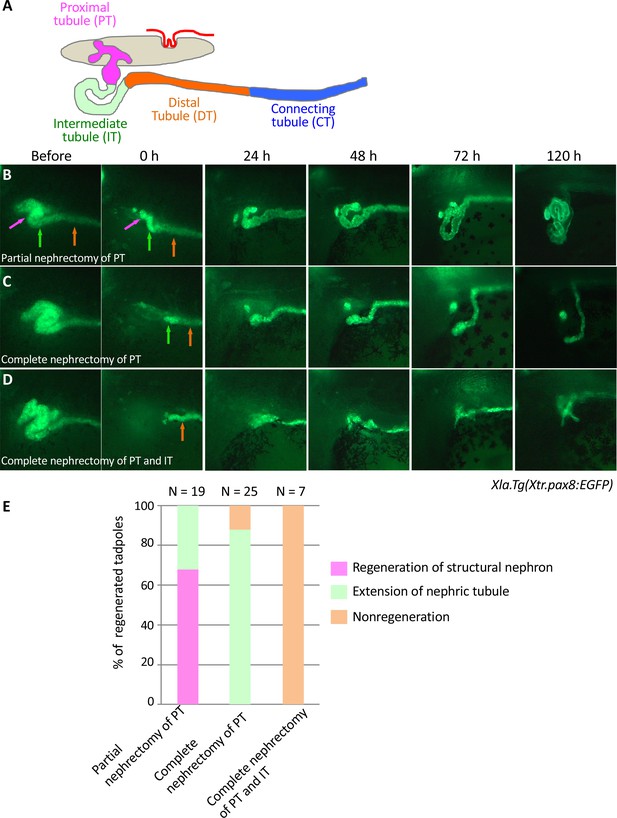
Live imaging of nephric tubules using transgenic X. laevis.
(A) A schematic image of X. laevis nephron. PT: proximal tubule; IT: intermediate tubule; DT: distal tubule; CT: connecting tubule. Magenta arrows: PT; green arrows: IT; orange arrows: DT. (B) Representative regeneration pattern of partially resected proximal tubules. Proximal tubules regenerate a coiled structure. (C) Regeneration pattern of completely resected proximal tubules. The remaining intermediate tubules extend, but no coiled structure is regenerated. (D) Regeneration pattern of completely resected intermediate tubules. No extension of tubules is observed. (E) Statistics of the regeneration pattern. The statistics of three independent experiments are summarized.
lhx1 expression appears in regenerating nephrons immediately after nephrectomy
In order to explore the molecular mechanisms behind the regeneration of nephric tubules, we examined the expression of the transcription factors osr1, osr2, lhx1, six2, hnf1b, hnf4a, pax2, pax8, and wt1 in regenerating nephric tubules. Among them, osr1, osr2, and lhx1, together with pax8, are known to induce ectopic nephrons when overexpressed in Xenopus embryos (Seufert et al., 1999; Carroll and Vize, 1999; Tételin and Jones, 2010), and lhx1- or six2-expressing mesenchymal cells can reconstruct functional nephrons in kidney-injured zebrafish (Diep et al., 2011). The in situ hybridization analysis showed that the expression of lhx1, pax8, hnf4a, hnf1b, and osr2 appeared within 24 hr after the nephrectomy (Figures 2A, D, G and J, Figure 2—figure supplement 1). Such expression became stronger around 48 hr (Figures 2B, E, H and K, Figure 2—figure supplement 1). Then, the expression of lhx1 disappeared at 96 hr, while the expression of pax8 was still detected in the nephric tubules (Figures 2C, F, I and L). In contrast, the expression of pax2 and osr1 appeared around 48 hr, and that of six2 was not detected in regenerating nephric tubules (Figure 2M–2R, Figure 2—figure supplement 1, data not shown). Although it is possible that primary induced lhx1-, pax8-, hnf4a-, hnf1b-, and osr2-expressing cells are not completely consistent with cells observed in Xla.Tg(Xtr.pax8:EGFP) regenerating nephric tubules, the immediate induction of lhx1, pax8, hnf4a, hnf1b, and osr2 after nephrectomy suggests that the loci of these genes contain enhancers of injury response.
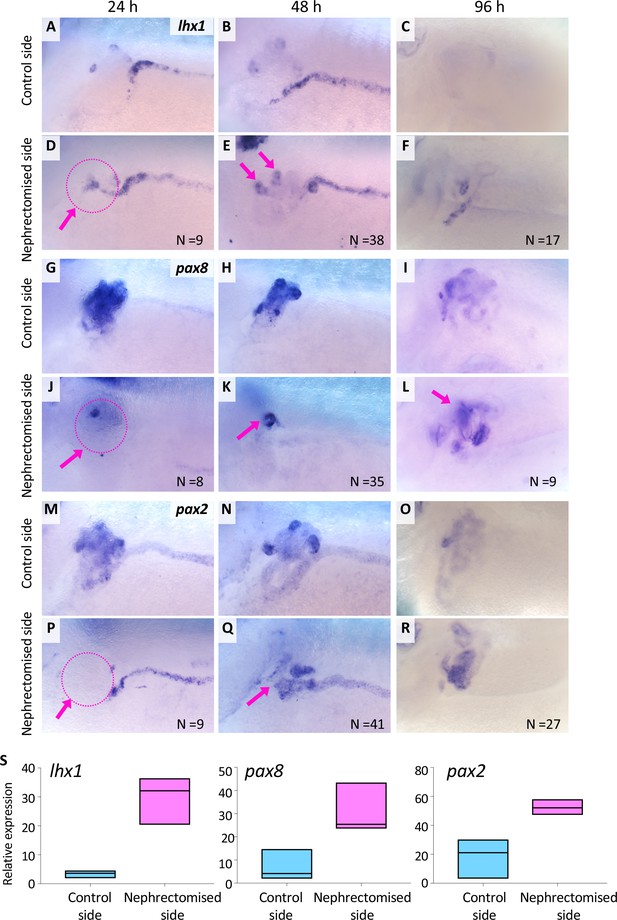
lhx1 expression appears immediately after nephrectomy.
(A–F) Expression of lhx1 on the control side and nephrectomized side at 24, 48, and 96 hr after nephrectomy. N indicates the number of examined embryos. (D) lhx1 expression appears in regenerating nephric tubules within 24 hr (arrows). (E, F) This expression becomes stronger around 48 hr but disappears at 96 hr. (G–L) Expression of pax8. pax8 expression appears in regenerating nephric tubules and is still detected at 96 hr after nephrectomy. (M–R) Expression of pax2 is not observed at 24 hr after nephrectomy (P, arrow). (S) Quantification of expression signals for lhx1, pax8, and pax2. The significance of differences between the control side and the nephrectomized side at 48 hr is calculated by two-tailed paired t-test (lhx1: p=0.0414; pax8: p=0.0102; pax2: p=0.0453). Lines in boxes indicate the median.
Mapping of RSREs
The expression of lhx1, pax8, and hnf1b was immediately induced after injury. It has been shown in a previous study involving simple mRNA injection using Xenopus embryos that Lhx1 with Pax8 can induce ectopic pronephron structures (Carroll and Vize, 1999). In addition, tissue-specific lhx1 knockout using CRISPR/Cas9 genome engineering clearly showed that Lhx1 is required for the development of nephric tubules in Xenopus (DeLay et al., 2018). These previous findings prompted us to focus on the mechanisms of lhx1 expression in regenerating nephric tubules. In order to explore the enhancers that activate lhx1 gene expression in regenerating nephric tubules, we first searched for evolutionarily conserved noncoding sequences (CNSs) as candidates for the enhancers (Woolfe et al., 2005; Ochi et al., 2012). In this study, we focused on two categories of CNSs. The first one is evolutionarily conserved between frog and zebrafish, which have a high regenerative capacity, and the other is conserved among human, mice, frog, and zebrafish (Figure 3). In order to identify CNSs, we compared the genomic sequence of a 365 kb segment encompassing the human LHX1 gene with the orthologous intervals in mice, opossums, frog (X. tropicalis), and zebrafish genomes using the MultiPipMaker alignment tool (Schwartz et al., 2000). This analysis identified 20 CNSs conserved between human and fish and 17 CNSs conserved between frog and zebrafish (Figure 3A). In order to find the enhancers that genuinely activate gene expression, we used an efficient transgenesis technique for a nonmosaic founder assay of X. laevis (Kroll and Amaya, 1996). Each X. tropicalis lhx1-CNS was cloned into a green fluorescent protein (GFP) reporter plasmid carrying a β-actin basal promoter (Ogino et al., 2008). Each construct was used to generate transgenic embryos, and the left side of the nephrons was then resected, after which the embryos were allowed to proceed to stage 37 (Figure 3B). Their GFP expression was examined 48 hr after nephrectomy (Figure 3B). Reporter constructs without a CNS showed no significant GFP expression (data not shown) (Ochi et al., 2012). When GFP expression was detected in the regenerating nephric duct, we counted this as a positive result (Figure 3C). CNSs for lhx1 conserved between frog and zebrafish showed enhancer activities, whereas CNSs conserved among vertebrates showed much stronger enhancer activities (Figure 3C). It is known that lhx1 is expressed in developing nephrons at the early tailbud stage and specifies the renal progenitor cell field (Taira et al., 1994; Carroll et al., 1999; Cirio et al., 2011). Therefore, we examined whether CNSs that have enhancer activities in regenerating nephric tubules are also activated in developing pronephros. The transgenic reporter analysis using early tailbud embryos showed that CNS17, CNS20, and CNS35 have enhancer activities in the eyes and somites at this stage, while lacking (CNS20 and CNS35) or showing (CNS17) very weak enhancer activities in developing pronephros, suggesting that these CNSs primarily function after nephrectomy (Figure 3—figure supplement 1). These results suggest that enhancers conserved between human and zebrafish regulate the reactivation of developmental genes in regenerating nephric tubules.
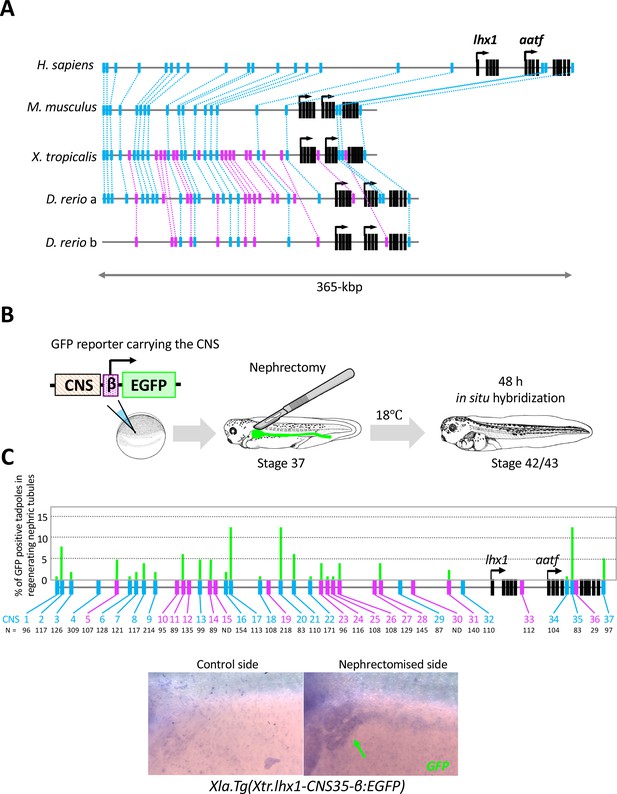
The RSRE for lhx1 is conserved between human and fish.
(A) A diagram of vertebrate lhx1 loci showing the position of CNSs. The magenta boxes indicate CNSs conserved between frog and fish, and the blue boxes indicate CNSs conserved between human and fish. The black boxes indicate the exons. (B) A diagram of the experimental design for mapping RSREs. GFP reporter constructs carrying lhx1-CNSs with the β-actin proximal promoter were subjected to transgenesis. All reporter-injected embryos underwent nephrectomy on the left side at stage 37. Nephrectomized embryos were incubated at 18°C for 48 hr and fixed at stages 42/43. Normally developed embryos were subjected to in situ hybridization in order to examine their GFP expression with maximum sensitivity. (C) A summary of RSRE screening. The green bar indicates % of GFP-positive tadpoles in regenerating nephric tubules. N indicates the number of scored tadpoles. The image shows a representative expression pattern of GFP in regenerating nephric tubules. The green arrow indicates the regenerating nephric tubule.
Arid3a functions as an input transcription factor for lhx1-RSREs
The transgenic reporter system of X. laevis combined with the surgical removal of nephric tubules revealed that the lhx1 loci contain the genomic elements that can activate gene expression in regenerating nephrons. We named these elements RSREs. Generally, enhancers activate gene expression by binding transcription factors (Bulger and Groudine, 2010). Therefore, the RSREs that we identified must also be regulated by transcription factors. In order to examine the transcription mechanisms for RSREs during nephron regeneration, we first searched for the binding motifs of transcription factors on RSREs. To dissect the transcriptional inputs for the RSREs, the open-access database JASPAR ver. 5 was used to define potential transcription factor binding sites (Mathelier et al., 2014). The candidate transcription factors were narrowed down according to their nephric expression using the Expression Atlas (the baseline atlas) (Petryszak et al., 2014), and then they were narrowed down further by phylogenetic footprinting (Figure 4—figure supplement 1). Although many lhx1-CNSs showed enhancer activities in regenerating nephric tubules, we focused on the top three lhx1 RSREs—CNS17, CNS20, and CNS35—which showed strong enhancer activities. The motif analysis showed that transcription motifs for Arid3a and Spib were commonly found in RSREs (Figure 4A). arid3a is known to be expressed in the ectoderm of the early neurula and in the epidermis at the late tailbud stage, whereas spib is expressed in the anterior ventral blood islands at the neurula stage (Callery et al., 2005; Costa et al., 2008). We performed an in situ hybridization analysis in order to determine whether these genes are also expressed in the nephric tubules and found that arid3a is expressed there, whereas spib is not (Figure 4B, Figure 4—figure supplement 2). In addition, we performed immunostaining of Arid3a using Xla.Tg(Xtr.pax8:EGFP), which showed that Arid3a protein was detected in proximal tubules and also in the glomus and/or nephrocoelom (Figure 4B, orange arrows and orange arrowheads, respectively). These results suggest that Arid3a is a good candidate for the input transcription factor for RSREs. Therefore, we next examined whether Arid3a regulates the expression of lhx1 in Xenopus and found that lhx1 was induced by conditionally induced arid3a in heat-shock-inducible transgenic X. laevis (Figure 4C) (Wheeler et al., 2000). We then examined whether Arid3a interacts with RSREs in X. laevis. The Myc-tagged X. tropicalis arid3a mRNA was injected into one-cell-stage X. laevis embryos, and then chromatin immunoprecipitation-qPCR (ChIP-qPCR) was performed using anti-Myc antibody. CNS35 was divided into two segments for qPCR, since it is 580 bp long, which is too long for qPCR. ChIP-qPCR showed that Arid3a directly binds to RSREs, but not to exon 5 and CNS32, which do not include putative Arid3a binding motifs (Figure 4D). Furthermore, a luciferase reporter analysis using 293 T cells derived from human embryonic kidneys was performed to examine whether Arid3a functions as a transcriptional activator for RSREs. We found that Arid3a activates CNS17 and CNS20 enhancer activities, whereas no activation was observed in the CNS35 reporter (Figure 4E). Since CNS35 is located in the intron of aatf, it is possible that the activation mechanism of this CNS is different from those of CNS17 and CNS20.
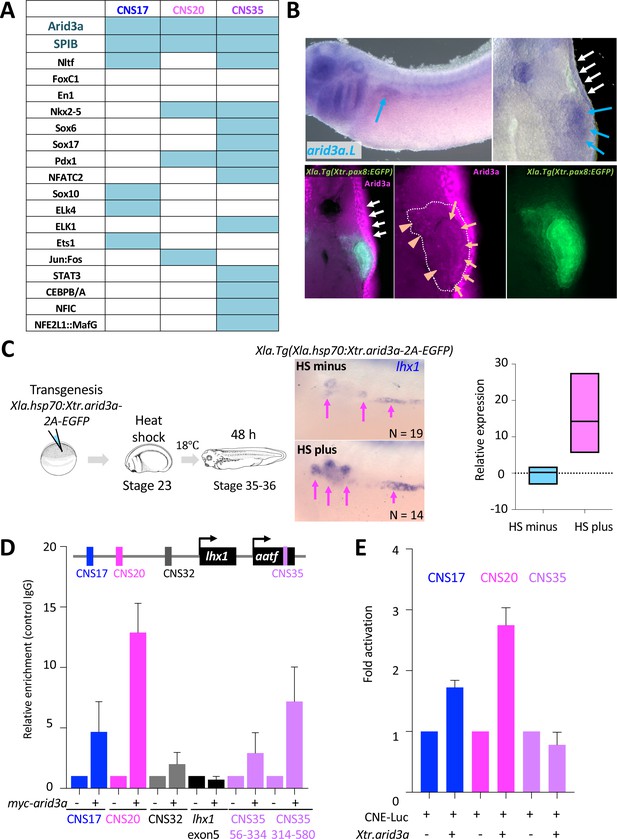
Arid3a is an input transcription factor for RSREs.
(A) A summary of transcription factor binding motifs on RSREs. (B) arid3a is expressed in nephric tubules. A lateral view of embryos at stage 31 and its transverse section. The upper panels show the in situ hybridization of arid3a.L. The blue arrows indicate the nephric tubules and the white arrows indicate the epidermis. The lower panels show immunostaining using anti-Arid3a using Xla.Tg(Xtr.pax8:EGFP) transgenic tadpole. White arrows: epidermis; orange arrows: proximal tubules; orange arrowheads: glomus and/or nephrocoelom. (C) Arid3a induces lhx1 expression. Xla.Tg(Xla.hsp70:Xtr.arid3a-2A-EGFP) transgenic X. laevis at stage 23 were treated at 34°C for 15 min, followed by 15 min at 14°C. These steps were repeated three times, and tadpoles were incubated at 18°C. lhx1 expression was observed 48 hr after the heat shock at stages 35–36. The signal intensity of in situ hybridization was measured and subjected to statistical analysis. The significance of differences between the control side and the nephrectomized side was calculated using two-tailed unpaired t-test (p=0.0131). The magenta arrows indicate lhx1 expression in proximal and intermediate tubules. N indicates the number of examined embryos. (D) Arid3a directly binds to CNS17, CNS20, and CNS35. Myc-tagged Xtr.arid3a mRNA-injected tadpoles were used for ChIP-qPCR. CNS32 and exon 5 were used as negative elements. The significance of differences between the control IgG and the anti-Myc for Arid3a was calculated using two-tailed unpaired Mann–Whitney t-test: CNS17, p=0.0286; CNS20, p=0.0022; CNS32, p=0.3143 (not significant); exon5, p=0.7000 (not significant); CNS35 (56-334), p=0.0079; CNS35 (314-580), p=0.0022. The error bars indicate SEM. (E) Arid3a activates CNS17 and CNS20. The luciferase reporter assay was performed using HEK293T cells. The significance of differences between the control vector and the CNS-containing reporter was calculated using two-tailed unpaired t-test (CNS17, p=0.0033; CNS20, p=0.0256). The error bars indicate SEM.
Arid3a promotes cell cycle progression in regenerating nephrons
During tail regeneration in X. laevis, the inhibition of apoptosis results in a failure to induce subsequent cell proliferation (Tseng et al., 2007). During proximal tubule regeneration, apoptosis occurs within 3 hr after nephrectomy, and the number of apoptotic cells is decreased at one day (Caine and Mclaughlin, 2013). Meanwhile, the nephric tubules begin to extend from the remaining tubules (Figure 1). During this regeneration step, cell proliferation must occur, as observed in other types of tissue regeneration (Poleo et al., 2001; Passamaneck and Martindale, 2012). Therefore, we first examined the time course of cell proliferation after nephrectomy (Figure 5—figure supplement 1). Immunofluorescence staining with anti-phosphorylated histone H3 antibody, a marker for mitotic cells, showed that there was no significant difference between the control and the nephrectomized sides until 6 hr after nephrectomy, whereas the number of proliferating cells increased on the nephrectomized side at 24 hr (Figure 5—figure supplement 1). It has been shown in a previous study that Arid3a stimulates cyclin E1/E2F-dependent cell cycle progression (Peeper et al., 2002). Therefore, we next investigated whether Arid3a contributes to the cell cycle progression. Xla.Tg(Xla.hsp70:Xtr.arid3a-2A-mcherry, Xtr.pax8:EGFP) and Xla.Tg(Xtr.pax8:EGFP) transgenic Xenopus embryos at tailbud stage 23 were treated with or without heat shock and then incubated for 48 hr at 18°C. Heat-shock-treated embryos were then sorted by mCherry positivity or negativity (Figure 5—figure supplement 2). These tadpoles were examined for the number of phosphorylated histones H3. No significant difference of phosphorylated histones H3 between the heat-shock-untreated embryos and the heat-shock-treated mCherry-negative embryos was observed (Figure 5—figure supplement 3). In contrast, the number of phosphorylated histones H3 in the mCherry-positive Xla.Tg(Xla.hsp70:Xtr.arid3a-2A-mcherry, Xtr.pax8:EGFP) was significantly increased compared with that in the heat-shock-treated Xla.Tg(Xtr.pax8:EGFP) and heat-shock-untreated Xla.Tg(Xla.hsp70:Xtr.arid3a-2A-mcherry, Xtr.pax8:EGFP) (Figure 5A). Since we showed that Arid3a can induce lhx1 expression, we then wondered whether Lhx1 can also promote the cell cycle. Transgenic X. laevis in which lhx1 was conditionally induced showed an increased number of phosphorylated histones H3, as observed in Arid3a-induced X. laevis (Figure 5B). These results suggest that Arid3a and also Lhx1 promote cell cycle progression after nephrectomy.
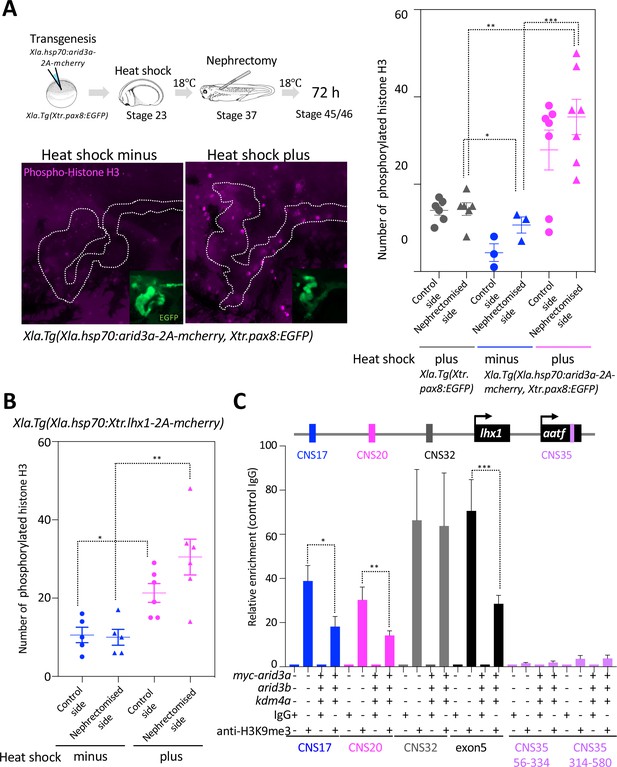
Arid3a promotes cell cycle progression.
(A) The number of phosphorylated histones H3 in the nephrectomized area was increased by the conditionally induced Arid3a. Heat-shocked Xla.Tg(Xla.hsp70:Xtr.arid3a-2A-mcherry, Xtr.pax8:EGFP) was nephrectomized at stage 37, incubated for 72 hr, and then fixed at stages 45/46. The white dotted lines indicate the pax8-expressing cells, and the magenta indicates the phosphorylated histone H3-positive cells. The left graph shows the statistical analysis; one-way analysis of variance (ANOVA) and Tukey’s post hoc test were used. ANOVA p=0.0001; *p = 0.5155 (not significant), **p = 0.001, ***p = 0.0019. The error bars indicate SEM. (B) Lhx1 promotes cell cycle progression in the regenerating area. Heat-shocked Xla.Tg(Xla.hsp70:Xtr.lhx1-2A-mcherry) was nephrectomized at stage 37 and then incubated for 72 hr. One-way ANOVA and Tukey’s post hoc test were used. ANOVA: p=0.0005, *p = 0.0015, and **p = 0.0011. (C) Arid3a with Kdm4a and Arid3b reduced the H3K9me3 levels on RSREs. ChIP analysis was performed using X. laevis. Significant differences were calculated by two-tailed unpaired t-test. The p-values from comparisons between the control and arid3a-, arid3b-, and kdm4a-injected embryos were as follows: *p = 0.0389, **p = 0.0313, and ***p = 0.0456. The error bars indicate SEM.
Arid3a with Arid3b and Kdm4a regulate the chromatin configuration of RSREs
The constitutive heterochromatin marker histone 3 lysine nine trimethylation (H3K9me3) is rapidly remodeled to create a chromatin environment, and the lack of H3K9me2/3 could allow global DNA demethylation (Burton and Torres-Padilla, 2010). Arid3a is known to stimulate the expression of immunoglobulin heavy chain (IgH) and is also known to change the accessibility of the IgH enhancer (Kim and Tucker, 2006; Lin et al., 2007). In addition, it has been shown in a previous study that the complex of Arid3a, Arid3b, and Kdm4c modulates the chromatin configuration of stemness genes for breast cancer by decreasing H3K9me3 (Liao et al., 2016). Therefore, we next investigated whether Arid3a modulates the H3K9me3 level on RSREs. ChIP-qPCR analysis using anti-H3K9me3 showed that H3K9me3 was enriched not only on the RSREs CNS17 and CNS20, but also on CNS32 and exon 5 of lhx1 (Figure 5C). However, no enrichment of H3K9me3 on CNS35 was observed (Figure 5C). Next, we found that the coinjection of arid3a, arid3b, and kdm4a decreased H3K9me3 on the RSREs CNS17 and CNS20, but not on CNS32 (Figure 5C). In addition, the enrichment of H3K9me3 on exon 5 was also decreased (Figure 5C). Thus, we found that Arid3a directly regulates lhx1 expression through changing the chromatin configuration on CNS17 and CNS20. In contrast, although CNS35 shows an enhancer activity in regenerating nephric tubules, both the chromatin modification and the activation mechanism differ from those of CNS17 and CNS20.
Arid3a is required for the regeneration of proximal tubules in Xenopus
Our results suggest that Arid3a is involved in the regeneration of nephric tubules in X. laevis. In order to investigate whether Arid3a is required for the regeneration of nephric tubules, we applied photo-morpholino oligonucleotide (Photo-MO) that was previously established in zebrafish in order to control the timing of gene knockdown (Tallafuss et al., 2012; Cabochette et al., 2015). The effectiveness of antisense-MO for gene knockdown is blocked by annealing with sense Photo-MO. Since Photo-MO is cleaved by ultraviolet (UV) treatment, we were able to control the timing of gene knockdown. We first designed the splicing-blocking morpholino for arid3a.L, since the expression arid3a.L in nephrons is stronger than that of arid3a.S (Figure 4B, Figure 4—figure supplement 1A). The arid3a.L-MO-injected embryos became abnormal during gastrulation, consistent with the phenotype that was previously reported using translational blocking morpholino (Figure 6—figure supplement 1B) (Callery et al., 2005). RT-PCR analysis using control and arid3a.L-MO-injected embryos showed that the level of transcripts that included exon three was dramatically reduced in arid3a.L-MO-injected embryos (Figure 6—figure supplement 1C). In contrast, embryos injected with arid3a.L-MO annealed with arid3a.L-Photo-MO developed normally (Figure 6—figure supplement 1D). Thus, arid3a.L-MO is rendered inactive by binding to arid3a.L-Photo-MO. Next, in order to investigate whether the photosensitive subunit is cleaved by 365 nm light, arid3a.L-photo-MO/arid3a.L-MO-injected embryos were treated with UV for 30 min at stages 29–30 (Figure 6A). RT-PCR analysis showed that the arid3a.L transcript, which included exon 3, was significantly decreased by treatment with UV light, same as upon injection of arid3a.L-MO alone (Figure 6A, Figure 6—figure supplement 1C). Since conditionally induced Arid3a promotes the expression of lhx1, we wondered whether the expression of lhx1 in regenerating nephric ducts is affected. In situ hybridization analyses showed that there was no significant increase of lhx1 in UV-treated embryos, suggesting that Arid3a is essential for the expression of lhx1 after nephrectomy (Figure 6B). Cell cycle progression occurred after nephrectomy (Figure 5—figure supplement 1). Therefore, we also examined whether arid3a knockdown induced by UV affects the cell cycle and found that the number of phosphorylated histone H3-positive cells after nephrectomy in UV-treated embryos was reduced (Figure 6—figure supplement 2). We then examined whether Arid3a contributes to the regeneration of proximal tubules. The proximal tubules of arid3a.L-photo-MO/arid3a.L-MO-injected embryos that were treated with UV were partially removed and subsequently incubated for 72 hr. The regenerated proximal tubules showed a coiled structure in UV-untreated controls (Figure 6C). In contrast, although regenerating proximal tubules extended to the front most of the resected X. laevis failed to reconstruct the coiled structure, indicating that Arid3a is required for the proper regeneration of nephric ducts in X. laevis (Figure 6C).
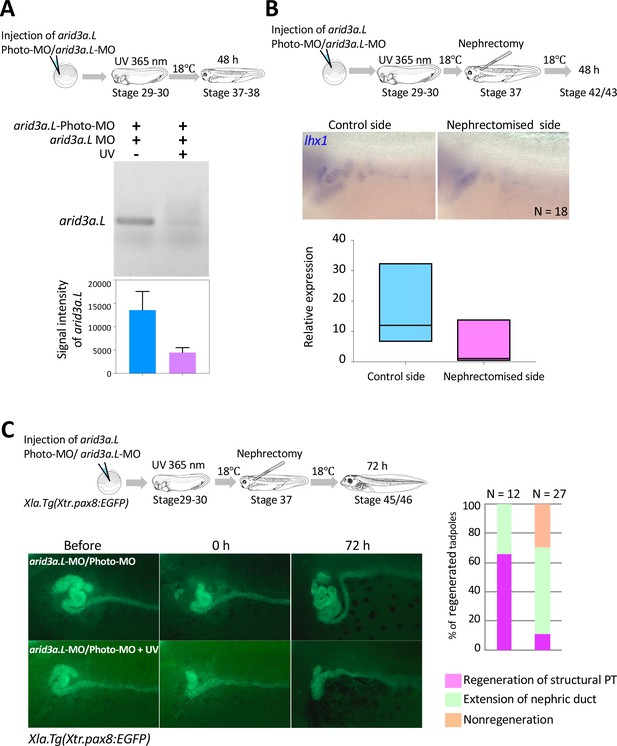
Arid3a is required for the regeneration of proximal tubules in X.laevis.
(A) Conditional knockdown of Arid3a using arid3a.L-photo-morpholinos (arid3a.L-Photo-MO). The upper panel shows the experimental design of conditional gene knockdown experiment using Photo-MO. arid3a.L-antisense-splicing-blocking MO (arid3a.L-MO) inactivated by Photo-MO is injected at the one-cell stage, subjected to UV exposure at stages 29–30, and then sacrificed for RT-PCR analyses. The lower panel shows the statistical analysis. The significance of differences between the UV-untreated and UV-treated embryos was calculated by two-tailed unpaired t-test (p=0.0217). The error bars indicate SEM. (B) Conditional knockdown of Arid3a during nephric regeneration causes the reduction of lhx1 expression. The upper panel shows the experimental design. arid3a.L-MO inactivated by Photo-MO is injected at the one-cell stage, followed by UV exposure at stages 29–30, nephrectomy at stages 36–37, and subsequently incubation for 48 hr. The lower panel shows the quantification of lhx1 expression signals. The analysis indicates that there was no significant difference between the control side and the nephrectomized side (two-tailed paired t-test, p=0.0748). N indicates the number of examined embryos. The lines in boxes indicate the median. (C) Arid3a is required for the regeneration of nephric tubules. The upper panel shows the experimental design. arid3a.L-MO inactivated by Photo-MO is injected at the one-cell stage, and UV exposure is performed at stages 29–30, followed by nephrectomy at stages 36–37 and subsequently incubation for 72 hr. The left panel shows a summary of the statistics of three independent experiments.
Discussion
In many cases of tissue regeneration, numerous developmental genes that are evolutionarily conserved among vertebrates become reactivated during regeneration (Poss, 2010; Witman et al., 2011; Halasi et al., 2012; Shibata et al., 2016). Therefore, it has been widely discussed that regeneration recapitulates developmental guidance (Witman et al., 2011; Halasi et al., 2012). Every gene in the genome requires cis-regulatory sequences such as a promoter and an enhancer to activate its expression, indicating that genes expressed in regenerating tissues also utilize enhancers and promoters for their expression (Bulger and Groudine, 2010). However, no extensive analysis has been performed on whether highly regenerative animals utilize unique cis-regulatory elements for regeneration and how such cis-regulatory elements become activated during regeneration. Previously, tissue regeneration enhancer elements were identified on the basis of epigenetic profiling (Kang et al., 2016). The screening of epigenetic profiles on the basis of open chromatin marks is useful to capture the regulatory blueprint in a genome-wide manner, while our one-by-one transgenic screening based on the actual activities in regenerating tissues provides a novel and powerful approach to directly identify tissue specificity and in vivo function. Once we identified the functional enhancers in the regenerating tissues, we were able to search for input transcription factors. Here, our transgenic analysis of functional cis-regulatory elements showed that RSREs for lhx1 are evolutionarily conserved between human and fish, and Arid3a with Arid3b and Kdm4a induces lhx1 by changing the chromatin configuration of RSREs (Figure 7A). In addition, we showed that knockdown of arid3a causes the failure of regeneration, whereas the conditionally induced Arid3a promotes cell cycle progression and causes the outgrowth of nephric tubules (Figure 7B and C, Figure 7—figure supplement 1).
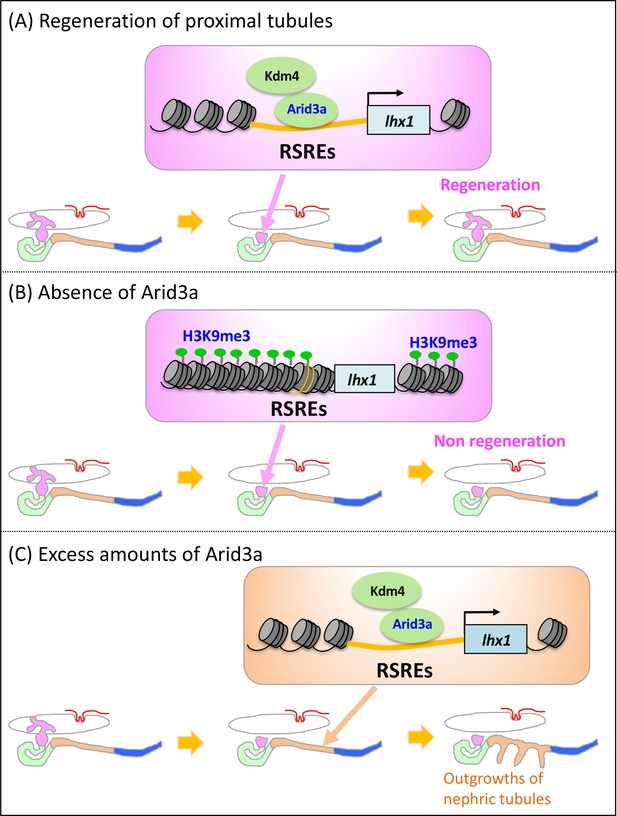
Model illustrating the Arid3a function in the regeneration of proximal nephric tubules.
(A) Arid3a binds to RSREs on lhx1 and changes the H3K9me3 levels. This chromatin modification allows the induction of lhx1 expression. (B) In the absence of Arid3a, proximal tubules fail to regenerate a complete nephron structure. (C) Excess amounts of Arid3a cause the outgrowth of nephric tubules from the distal nephric duct.
lhx1 is one of the earliest genes to be expressed in the pronephric anlagen, and its expression disappears during proximal tubule development (Carroll et al., 1999; Carroll and Vize, 1999) (Figure 2). As it recapitulates the developmental program, lhx1 expression appeared at the early stage in the construction of kidneys in vivo (Takasato et al., 2015). During in vivo nephric regeneration, lhx1 is one of the earliest genes to be expressed in regenerating nephrons (Figure 2). This lhx1 expression pattern prompted us to examine its noncoding DNA sequences. The Xenopus lhx1/xlim-1 enhancer responding to activin has been reported to encompass approximately 14.2 kb, including 5 kb upstream and the gene body (Rebbert and Dawid, 1997; Watanabe et al., 2002). Since recent genome-wide analyses have shown that enhancers are located not only near the gene body but also far away from it, we compared the genomic sequence of a 365 kb segment encompassing the human LHX1 gene with the orthologous intervals in mice, opossums, X. tropicalis, and zebrafish (Woolfe et al., 2005; Ochi et al., 2012). While the activin-responding lhx1 enhancer is located in intron 1, enhancers that showed high activities in regenerating nephrons are located 177 kbp (CNS17) and 144 kbp (CNS20) upstream of lhx1 and are also found in the intron of the next gene aatf (CNS35) (Figure 3). It is known that many genes have multiple enhancers (Bulger and Groudine, 2010). Such multiple enhancers often play different functional roles, while it is also known that they sometimes show overlapping functions (Osterwalder et al., 2018). CNS17, CNS20, and CNS35 are activated in regenerating nephric tubules, indicating that these enhancers have overlapping functions. On the other hand, we also found that CNS17 and CNS20 are modified by H3K9me3 but not CNS35 (Figures 3C and 5C). It has been shown in recent studies that functionally redundant enhancers provide phenotypic robustness (Osterwalder et al., 2018). Our observation that there are overlapping functions of CNS17, CNS20, and CNS35 in the regenerating nephric tubules, albeit with differential activation mechanisms, suggests that there is a redundancy of cis-regulatory elements for regeneration. In addition, we also found that many CNSs that are only conserved between frog and fish showed weak enhancer activities in regenerating nephric tubules (Figure 3C). Since these animals have a high regenerative capacity, it is possible that such weak multiple enhancers possessing overlapping activities may also be key to boosting the gene expression in regenerating tissues. Further studies of transcriptional mechanisms for frog/fish CNSs may provide novel insights into the unique trait of high regenerative capacity in fish and frog.
In zebrafish, lhx1a and six2 mesenchymal cells reconstruct the functional nephrons (Diep et al., 2011). In contrast, our live imaging using Xla.Tg(Xtr.pax8:EGFP) together with McLaughlin’s previous findings suggests that the wound healing and regrowth of existing tubules occur during the regeneration of the nephric tubules of X. laevis, since the remaining nephric tubule cells extend toward the glomus (Caine and Mclaughlin, 2013). In mammals, mature tubular epithelial cells rapidly lose their brush border and dedifferentiate into mesenchymal-like cells following acute kidney injury, and these cells proliferate to regenerating nephric tubules (Maeshima et al., 2014). Therefore, the regenerative mechanisms of the nephric tubules of X. laevis appear to be much closer to the regeneration of nephric tubules in mammals rather than the reconstruction of nephrons from stem cells. Further live imaging studies using transgenic animals with markers of dedifferentiating cells and regrowing cells are required in order to reveal the detailed regenerative mechanisms of nephric tubules in vertebrates.
Recently developed in vivo organ construction technologies enabled us to make nephric structures derived from iPSCs and ES cells by the combined application of the Wnt activator and other signaling factors such as BMP4 (Xia et al., 2013; Takasato et al., 2014; Takasato et al., 2015). Moreover, direct reprogramming from fibroblasts into renal tubular epithelial cells, called iRECs, has successfully converted mouse and human fibroblasts into renal tubular epithelial cells using a combination of transcription factors: Emx2, Hnf1b, Hnf4a, and Pax8 (Kaminski et al., 2016; Lienkamp, 2016). In Xenopus, it is well known that mRNA injection of Wnt11b, Osr1, Osr2, and Lhx1 with Pax8 can induce ectopic pronephron structures (Seufert et al., 1999; Carroll and Vize, 1999; Tételin and Jones, 2010). Although Arid3a is not strong enough to induce complete ectopic nephrons as with Osr1, Osr2, Lhx1, and Pax8 mRNA injection, our findings suggest that Arid3a may contribute to the improvement of the efficiency of in vitro reconstruction and in vivo direct reprogramming or regeneration of nephrons.
For the expression of stem cell factors, Arid3a changes its localization from cytoplasmic to nuclear, which is regulated by importin-9 (Liao et al., 2016). It has been shown in a recent study that A disintegrin and metalloproteinase 13 (ADAM13) increases the nuclear localization of a cleavage product of Arid3a and promotes the expression of the target gene tfap2 alpha (Khedgikar et al., 2017). ADAMs are known to control a variety of cell functions by modulating the ectodomain shedding of several membrane-anchored signaling molecules (Blobel, 2005). In addition, emerging evidence indicates that ADAMs are required for wound healing and the regeneration of oligodendrocytes (Schönefuß et al., 2012; Palazuelos et al., 2015). Therefore, it is possible that ADAMs change the localization of Arid3a after nephrectomy; nuclear-translocated Arid3a regulates H3K9me3 on RSREs, and this epigenetic alteration triggers lhx1 expression in regenerating nephric tubules. Further studies are necessary to reveal the molecular basis behind the signal transduction from the extracellular region to the Arid3a complex on lhx1 RSREs.
Materials and methods
Reagent type (species) or resource | Designation | Source or reference | Identifiers | Additional information |
---|---|---|---|---|
Gene (Xenopus tropicalis) | arid3a | This paper | RefSeq: NM_001011106.1 | |
Gene (Xenopus tropicalis) | arid3b | This paper | RefSeq: XM_002938881.4 | |
Gene (Xenopus tropicalis) | lhx1 | This paper | RefSeq: NM_001100228.1 | |
Genetic reagent (Xenopus laevis) | Xla.Tg(Xtr.pax8:EGFP) | Ochi, H., et al., 2012; doi: 10.1038/ncomms1851. | ||
Cell line (Homo sapiens) | 293T | RIKEN BRC CELL BANK | RCB2202, RRID:SCR_003163 | |
Transfected construct | pGL4.23 | Promega | E8411 | |
Transfected construct | pGL-lhx1-CNS17-Luc | This paper | New regent. The CNS17 fragments from IS-lhx1-CNS17-β-GFP vector introduced into the SacI and EcoRV sites of pGL4.23 vector. | |
Transfected construct | pGL-lhx1-CNS20-Luc | This paper | New regent. The CNS20 fragments from IS-lhx1-CNS17- β-GFP wereintroduced into the SacI and EcoRV sites of pGL4.23 vector. | |
Transfected construct | pGL-lhx1-CNS35-Luc | This paper | New regent. The CNS35 fragments from IS-lhx1-CNS17- β-GFP vector were introduced into the SacI and EcoRV sites of pGL4.23 vector. | |
Antibody | Anti-phospho histone H3 (Ser10) antibody | Milipore | 06–570 | (1:1000) |
Antibody | Anti-Arid3a antibody | DSHB | PCRP-ARID3A-1E9, RRID:AB_2618410 | (1:10) |
Antibody | Alexa 488 -conjugated goat anti-rabbit IgG | Invitrogen | A11001 | (1:1000) |
Antibody | Alexa 568-conjugated goat anti-mouse IgG | Invitrogen, | A11011 | (1:1000) |
Antibody | Anti-H3K9 (tri-methyl K9) antibody | Abcam, | ab8898 | (1:750) |
Antibody | Mouse monoclonal c-Myc (9E10) antibody | Santa Cruz Biotechnology Inc. | sc-40, RRID:AB_291323 | (1:750) |
Recombinant DNA reagent | Xenopus laevis hsp70 promoter | Wheeler, G. N., et al. 2000; doi.org/10.1016/S0960-9822 (00)00596–0 | ||
Recombinant DNA reagent | IS-β-GFP reporter | Ogino, H., et al., 2008; doi: 10.1242/dev.009548 | ||
Recombinant DNA reagent | pCS-myc-arid3a | New regent. The PCR product was introduced into the XhoI and XbaI sites of the pCS2 + MT plasmid. | ||
Recombinant DNA reagent | pCS-his-arid3b | New regent. The PCR product was introduced into the ClaI and XbaI site of the pCS vector. | ||
Recombinant DNA reagent | hsp70-myc-arid3a-2A-mcherry | This paper | New regent. The PCR amplified myc-arid3a and 2A-mcherry were introduced into the ClaI and XbaI sites of the IS-hsp70-cloning vector. | |
Recombinant DNA reagent | hsp70-myc-arid3a-2A-EGFP | This paper | New regent. The PCR amplified myc-arid3a and 2A-EGFP were introduced into the ClaI and XbaI sites of the IS-hsp70-cloning vector. | |
Recombinant DNA reagent | hsp70-lhx1-2A-EGFP | This paper | New regent. The PCR amplifiedlhx1 was introduced into the EcoRI sites of the pCS2 + MT plasmid. The PCR amplified myc-lhx1 and 2A-EGFP were introduced into the EcoRI and XbaI sites of the IS-hsp70-cloning vector. | |
Recombinant DNA reagent (Xenopus laevis) | arid3a.L | This paper | Xelaev18006256m | New regent. The PCR product was introduced into the EcoRI and XhoI sites of the pBluescript II SK plasmid. |
Recombinant DNA reagent (Xenopus laevis) | arid3a.S | This paper | Xelaev18009788m | New regent. The PCR product was introduced into the EcoRI and XhoI sites of the pBluescript II SK plasmid. |
Recombinant DNA reagent (Xenopus laevis) | spib. L | This paper | Xelaev18036193m.g | New regent. The PCR product was introduced into the EcoRI and XhoI sites of the pBluescript II SK plasmid. |
Recombinant DNA reagent (Xenopus laevis) | spib.S | This paper | Xelaev18037903m.g | New regent. The PCR product was introduced into the EcoRI and XhoI sites of the pBluescript II SK plasmid. |
Recombinant DNA reagent (Xenopus laevis) | hnf4a | This paper | Xelaev17043619m, Xelaev17043619m | New regent. The PCR product was introduced into the BamHI and HindIII sites of the pBluescript II SK plasmid. |
Recombinant DNA reagent (Xenopus laevis) | hnf1b | This paper | Xelaev18012186m.g, Xelaev18014991m.g | New regent. The PCR product was introduced into the BamHI and HindIII sites of the pBluescript II SK plasmid. |
Recombinant DNA reagent (Xenopus laevis) | osr1 | This paper | Xelaev14054577m.g, Xelaev14010174m.g | New regent. The PCR product was introduced into the XhoI and BamHI sites of the pBluescript II SK plasmid. |
Recombinant DNA reagent (Xenopus laevis) | osr2 | This paper | Xelaev14045820m.g, Xelaev14031017m.g | New regent. The PCR product was introduced into the XhoI and BamHI sites of the pBluescript II SK plasmid. |
Recombinant DNA reagent (Xenopus laevis) | six2 | This paper | Xelaev16000858m, Xelaev16036496m | New regent. The PCR product was introduced into the HindIII and XhoI sites of the pBluescript II SK plasmid. |
Recombinant DNA reagent (Xenopus laevis) | lhx1 | This paper | Xelaev16044871m.g | New regent. The PCR product was introduced into the SmaI and HindIII sites of the pBluescript II SK plasmid. |
Recombinant DNA reagent (Xenopus laevis) | pax2 | Heller and Brändli, 1997: doi.org/10.1016/S0925- 4773(97)00158-5 | ||
Recombinant DNA reagent (Xenopus laevis) | pax8 | Heller and Brändli, 1999: doi.org/10.1002/(SICI)1520- 6408(1999)24:3/4 < 208::AID- DVG4 > 3.0.CO;2 J | ||
Recombinant DNA reagent (Mus musculus) | kdm4a | Mammalian Gene Collection (MGC) Clones | 4207552 | BC028866 |
Sequence-based reagent (Xenopus tropicalis) | PCR primers for CNS | This paper | ||
Sequence-based reagent (Xenopus laevis) | ChIP-qPCR primers | This paper | ||
Sequence-based reagent (Xenopus laevis) | Photo-Morpholino oligonucleotide for arid3a,L | This paper | Gene Tools, LLC | AGAGGGAAGCCAGCAGGTACTCACC |
Sequence-based reagent (Xenopus laevis) | Morpholino oligonucleotide for arid3a,L | This paper | Gene Tools, LLC | AGTACCTGpTGGCTTCCCT |
Sequence-based reagent (Xenopus laevis) | PT-PCR primers for arid3a.L | This paper | ||
Sequence-based reagent (Homo sapiens) | hg19 chr17-34994909–35360679 | hg19 | UCSC Genome Browser, RRID:SCR_005780 | |
Sequence-based reagent (Mus musculus) | mm10 chr11-83838963–85151744 | mm10 | UCSC Genome Browser, RRID:SCR_005780 | |
Sequence-based reagent (Monodelphis domestica) | monDom5 chr2-185210169–185976291 | monDom5 | UCSC Genome Browser, RRID:SCR_005780 | |
Sequence-based reagent (Xenopus tropicalis) | xenTro3 GL173152-472286-845619 | xenTro3 | Xenbase, RRID:SCR_003280 | |
Sequence-based reagent (Danio rerio) | danRer10-chr15_27468859–28180541 | danRer10 | UCSC Genome Browser, RRID:SCR_005780 | |
Sequence-based reagent (Danio rerio) | danRer10- chr5_55422952–55633560 | danRer10 | UCSC Genome Browser, RRID:SCR_005780 | |
Software, algorithm | GraphPad Prism 7.0 | GraphPad Software | RRID:SCR_002798 | |
Software, algorithm | Adobe Photoshop | Adobe | RRID:SCR_014199 | |
Software, algorithm | MultiPipMaker | Schwartz, S., et al., 2000: doi: 10.1101/gr.10.4.577 | RRID:SCR_011806 | |
Software, algorithm | JASPAR ver. 5 | Mathelier, A., et al., 2014: doi: 10.1093/nar/gkt997. | RRID:SCR_003030 | |
Commercial assay or kit | ISOGEN | NIPPON GENE | Code No. 317–02503 | |
Commercial assay or kit | Dual-Luciferase Reporter Assay System | Promega | E1910 | |
Commercial assay or kit | Dynabeads Protein A | Dynabeads | 10001D | |
Chemical compound, drug | jetPEI (transfection) | Polyplus-transfection SA | 101–10N |
Construction of reporter plasmids
Request a detailed protocolThe GFP reporter plasmid carrying a chicken β-actin basal promoter (−55 to +53) was previously described as β-GFP (Ogino and Ochi, 2009). The CNSs were amplified from X. tropicalis genomic DNA by PCR and cloned into β-GFP reporter vectors. The primer sequences used in this study are summarized in Supplementary file 1.
Identification of CNSs
Request a detailed protocolThe 365 kb genomic sequence of the human LHX1 locus [hg19 chr17-34994909–35360679 (365,771 bp)] and its orthologous sequences in mice [mm10 chr11-83838963–85151744 (1,312,782 bp)], opossums [monDom5 chr2-185210169–185976291 (766,123 bp)], X. tropicalis [xenTro3 GL173152-472286-845619 (373,334 bp)], and zebrafish [danRer10-chr15_27468859–28180541 (711,683 bp), danRer10-chr5_55422952–55633560 (210,609 bp)] were downloaded from the UCSC Genome Browser and Xenbase Genome Browser. These sequences were aligned using MultiPipMaker (Schwartz et al., 2000).
Cloning of X. tropicalis Arid3a and Arid3b
Request a detailed protocolFull-length cDNA fragments of arid3a and arid3b were amplified from a cDNA pool of X. tropicalis tailbud embryos (stage 26). The product was introduced into the XhoI and XbaI sites of the pCS2 +MT plasmid and the ClaI and XbaI site of the pCS2 vector. The PCR-amplified X. laevis heat-shock promoter, Xtr.arid3a, and 2A-mcherry DNA fragments were constructed using the InFusion cloning method (Wheeler et al., 2000) (Clontech, Palo Alto, CA, USA). For the in situ hybridization probes of X. laevis, arid3a.L and arid3a.S were amplified from a cDNA pool of X. laevis tailbud embryos (stages 35/36). The primers used for the vector construction are listed in Table 1.
Primer sequences for the RT-PCR and quantitative RT-PCR.
https://doi.org/10.7554/eLife.43186.020Xtr.arid3a_full-length-F | ATGAAGCTGCAAGCGGTG |
Xtr.arid3a_full-length-R | TCAGGGAGAAGGATTGTTAG |
Xtr.arid3b_full-length-F | CGATGCCGCCACCATGCACCATCACCACCATCATCACCACCATCACT |
Xtr.arid3b_full-length-R | CTAGAGTGATGGTGGTGATGATGGTGGTGATGGTGCATGGTGGCGGCAT |
Xla.CNS17-qPCR-F | CTGAGTGAGTTTCAAATAAAAGGATTAAG |
Xla.CNS17-qPCR-R | GCTATGTAGAGTGGAATAGAGTTAGAATGA |
Xla.CNS20-qPCR-F | AATACTCACACAGGGAAGACAGC |
Xla.CNS20-qPCR-R | AAGGCCAAAATTACTTTTCATTTATCTTA |
Xla.CNS32-qPCR-F | GGGAATTAACCCCCATGGGAA |
Xla.CNS32-qPCR-F | TTTGCCTCCCTCCTGATCTATAGG |
Xla.exon5-qPCR-F | CCAGGTTCCATGCACTCTATG |
Xla.exon5-qPCR-R | TTTCTGGTGGGTGTGACAAA |
Xla.CNS35-qPCR-56–334 F | AGTTTATAATCTCTGCCGTGCT |
Xla.CNS35-qPCR-56–334 R | TGTGCTGCTTGGAATTCAAG |
Xla.CNS35-qPCR-314–580 F | CTTGAATTCCAAGCAGCACAT |
Xla.CNS35-qPCR-314–580 R | CCTCAAGAACAATTCTCATTTAAATCCAC |
Xla.arid3a-L-exon2-RT-PCR-F | CCCAAGCAATCTAGTCAACAGACATTTCC |
Xla.arid3a-L-exon4-RT-PCR-R | GCTGCACTGGTGATTGAAGTTGGTAG |
Xla.lhx1-F | TCTACTGTAAAAACGACTTCTTCAGG |
Xla.lhx1-R | CCATTGACTGATAGAGAAGAAAAGG |
Xla.six2.L-F | CGAAGCCAAAGAGAGGTACG |
Xla.six2.L-R | TTGGGATCCTTCAACTCTGG |
Xla.six2.S-F | ACCCGTTGTCCTCTTCAATG |
Xla.six2.S-R | TGACCTGCTGAATGCAAGT |
Xla.osr1-F | TCCTTCCTACAAGCCTTCAATGGAC |
Xla.osr1-R | CTGAACAGAACACAATCATGTACAAGGAATTC |
Xla.osr2-F | GGGAAGATGGGCAGCAAAGCT |
Xla.osr2-R | TAGAAGTCCTGTCTGGGGCTGTG |
Xla.hnf1b-F | TGGCTATGGATGCCTATAGTACTGGCC |
Xla.hnf1b-R | TGCTGATGCTGCTAGTATCTGTGACAAC |
Xla.hnf4a-F | CGGCTTTCTGTGAACTTCCACTGG |
Xla.hnf4a-R | CTACATAGCTTCCTGTTTGGTGATGGTC |
Transgenic reporter assay
Request a detailed protocolX. laevis embryos were generated by the sperm nuclear transplantation method with oocyte extracts (Kroll and Amaya, 1996). The manipulated embryos were cultured until stage 37, and all normally developed embryos were subjected to in situ hybridization in order to examine their GFP expression with maximum sensitivity. All CNS-carrying reporters were tested at least three times. The frequency of GFP expression varied depending on the constructs, but all constructs exhibited a reproducible expression pattern.
Nephrectomy
Request a detailed protocolWe first undertook training in the surgical removal of nephric tubules using stage 37 Xla.Tg(Xtr.pax8:EGFP) embryos in accordance with McLaughlin’s method (Caine and Mclaughlin, 2013). Then, we removed the nephric tubules of Xla.Tg(Xtr.lhx1-CNSs:EGFP). All nephrectomized X. laevis were cultured at 18°C until 24, 48, 72, and 120 hr after nephrectomy.
Motif analysis for transcription factor binding sites
Request a detailed protocolJASPAR ver. 5, an open-access database, was used to search for potential transcription factor binding sites in nephric enhancers (Mathelier et al., 2014). The candidate transcription factors were narrowed down according to their expression using the Expression Atlas (Petryszak et al., 2014). CNSs were aligned using ClustalW, and conserved sequences for the candidate transcription factor binding sites were further analyzed by phylogenetic footprinting (Blanchette and Tompa, 2002).
In situ Hybridization and Immunostaining
Request a detailed protocolThe cDNA clones for X. laevis lhx1, pax8, hnf4a, hnf1b, osr1, osr2, and six2 probes were synthesized from stages 35/36. The primers used for cDNA cloning are listed in Table 1. pax2 and pax8 cDNA clones were kind gifts from Dr. Brändli (Heller and Brändli, 1997; Heller and Brändli, 1999). The nephrectomized transgenic X. laevis were subjected to in situ hybridization in order to examine their GFP expression with maximum sensitivity. All CNS-carrying reporters were tested at least three times. The frequency of GFP expression varied depending on the constructs, but all constructs exhibited a reproducible expression pattern. The CNSs that drove nephric expression in more than 10% of the examined embryos were defined as RSREs. For whole-mount immunostaining, nephrectomized X. laevis were fixed in 3.7% formaldehyde/MEM at 4°C overnight. After fixation, embryos were washed with 100% ethanol and then incubated in a 2% BSA/PBS-t blocking solution. The embryos were then transferred to a primary antibody solution. A 1 : 1,000-diluted rabbit anti-phospho-histone H3 (Ser10) antibody (Millipore, 06–570) or 1 : 10-diluted anti-Arid3a antibody (DSHB, PCRP-ARID3A-1E9) was used as the primary antibody in conjunction with 1 : 1,000-diluted Alexa 488-conjugated goat anti-rabbit IgG (A11001; Invitrogen) and 1 : 1,000-diluted Alexa 568-conjugated goat anti-mouse IgG (A11011; Invitrogen) secondary antibodies. Images were acquired using ApoTome.2 (Axio Zoom.V16; Carl Zeiss).
Quantification of in situ Hybridization Signals
Request a detailed protocolIn situ hybridization signals were photographed using an Axio Zoom.V16 with AxioCam MRc cameras (Carl Zeiss). The images were then subjected to further analysis using Adobe Photoshop CS (Adobe, San Jose, CA, USA) as described in the Results. The in situ hybridization signals were first inverted, and then the relative luminosity, determined as the signal-positive nephric duct area minus an equal area of background, was measured. Then, the average luminosity was subjected to statistical analysis. The significance of differences between the control side and the nephrectomized side was calculated in Prism7 using two-tailed paired t-test (GraphPad Software).
Quantification of proliferation
Request a detailed protocolThe nephrectomized X. laevis processed for immunohistochemistry with phosphor-histone H3 (H3P) were photographed at the same magnification and exposure time. H3P-positive cells were counted in a square region (160 mm2) of regenerating tubules indicated by GFP expression of Xla.Tg(Xtr.pax8:EGFP).
Luciferase reporter assay
Request a detailed protocolFor the luciferase reporter assay, lhx1-CNSs were cloned into the pGL4.23 vector (Promega, Madison, WI, USA). HEK293T cells seeded in 24-well plates were transfected with 100 ng of lhx1-CNS luciferase plasmids and 10 ng of Renilla luciferase plasmids using jetPEI (Polyplus Transfection SA, Illkirch, France). The total amount of DNA per well was adjusted to 1 μg with pBKS plasmids. Transfected cells were incubated for 48 hr, and then the luminescence signals were measured following the manufacturer’s protocol (Promega).
ChIP analysis for lhx1-CNSs
Request a detailed protocolChIP was performed as described previously (Gazdag et al., 2016). Myc-Xtr.arid3a-, Xtr.Arid3b-, and mouse kdm4a mRNA-injected embryos were collected at stages 35/36 and subjected to ChIP using Dynabeads Protein A (Dynabeads, Great Neck, NY, USA). The following antibodies were used: rabbit polyclonal anti-H3K9 (tri-methyl K9) antibody (ab8898; Abcam, Cambridge, MA, USA). and mouse monoclonal c-Myc (9E10) antibody (sc-40; Santa Cruz Biotechnology Inc., Santa Cruz, CA, USA). Decrosslinking and elution of DNA were performed for real-time quantitative PCR using StepOne Plus (Thermo Fisher Scientific, Waltham, MA, USA). Injection and ChIP-qPCR were performed at least three times independently.
Conditional knockdown using Photo-Morpholino
Request a detailed protocolThe splicing-blocking antisense-MOs for Arid3a.L and photo-morpholinos (Gene Tools, Philomath, OR, USA) used in this study were as follows:
arid3a.L-exon2-intron2-MO: AGAGGGAAGCCAGCAGGTACTCACC.
arid3a.L-exon2-intron2-photo-MO: AGTACCTGpTGGCTTCCCT.
A ratio of 0.9 : 1 of photo-MO to MO was annealed at room temperature for 30 min, and then 2 nM photo-MO/MO was injected with a GFP tracer into one-cell-stage embryos. Cleavage of the antisense of photo-MO was performed by exposing embryos to UV light (365 nm) for 30 min.
Data availability
All data were generated and analysed during this study are included in the manuscript and supporting files.
References
-
ADAMs: key components in EGFR signalling and developmentNature Reviews Molecular Cell Biology 6:32–43.https://doi.org/10.1038/nrm1548
-
Transcriptional control of kidney developmentDifferentiation 72:295–306.https://doi.org/10.1111/j.1432-0436.2004.07207001.x
-
Towards a molecular anatomy of the Xenopus pronephric kidneyThe International Journal of Developmental Biology 43:381–395.
-
Enhancers: The abundance and function of regulatory sequences beyond promotersDevelopmental Biology 339:250–257.https://doi.org/10.1016/j.ydbio.2009.11.035
-
Epigenetic reprogramming and development: a unique heterochromatin organization in the preimplantation mouse embryoBriefings in Functional Genomics 9:444–454.https://doi.org/10.1093/bfgp/elq027
-
Regeneration of functional pronephric proximal tubules after partial nephrectomy in Xenopus laevisDevelopmental Dynamics 242:219–229.https://doi.org/10.1002/dvdy.23916
-
The ARID domain protein dril1 is necessary for TGF(beta) signaling in Xenopus embryosDevelopmental Biology 278:542–559.https://doi.org/10.1016/j.ydbio.2004.11.017
-
Synergism between Pax-8 and lim-1 in embryonic kidney developmentDevelopmental Biology 214:46–59.https://doi.org/10.1006/dbio.1999.9414
-
Xenopus: a prince among models for pronephric kidney developmentJournal of the American Society of Nephrology 16:313–321.https://doi.org/10.1681/ASN.2004070617
-
A regulated nucleocytoplasmic shuttle contributes to Bright's function as a transcriptional activator of immunoglobulin genesMolecular and Cellular Biology 26:2187–2201.https://doi.org/10.1128/MCB.26.6.2187-2201.2006
-
Progress and challenges in bioinformatics approaches for enhancer identificationBriefings in Bioinformatics 17:967–979.https://doi.org/10.1093/bib/bbv101
-
Transgenic Xenopus embryos from sperm nuclear transplantations reveal FGF signaling requirements during gastrulationDevelopment 122:3173–3183.
-
Using Xenopus to study genetic kidney diseasesSeminars in Cell & Developmental Biology 51:117–124.https://doi.org/10.1016/j.semcdb.2016.02.002
-
Regenerative medicine for the kidney: renotropic factors, renal stem/progenitor cells, and stem cell therapyBioMed Research International 2014:1–10.https://doi.org/10.1155/2014/595493
-
Resources and transgenesis techniques for functional genomics in XenopusDevelopment, Growth & Differentiation 51:387–401.https://doi.org/10.1111/j.1440-169X.2009.01098.x
-
Oligodendrocyte Regeneration and CNS Remyelination Require TACE/ADAM17Journal of Neuroscience 35:12241–12247.https://doi.org/10.1523/JNEUROSCI.3937-14.2015
-
Cell proliferation and movement during early fin regeneration in zebrafishDevelopmental Dynamics 221:380–390.https://doi.org/10.1002/dvdy.1152
-
Advances in understanding tissue regenerative capacity and mechanisms in animalsNature Reviews Genetics 11:710–722.https://doi.org/10.1038/nrg2879
-
Early organogenesis of the kidneyPediatric Nephrology 1:385–392.https://doi.org/10.1007/BF00849241
-
Role of ADAM-15 in wound healing and melanoma developmentExperimental Dermatology 21:437–442.https://doi.org/10.1111/j.1600-0625.2012.01490.x
-
PipMaker--a web server for aligning two genomic DNA sequencesGenome Research 10:577–586.https://doi.org/10.1101/gr.10.4.577
-
Developmental basis of pronephric defects in Xenopus body plan phenotypesDevelopmental Biology 215:233–242.https://doi.org/10.1006/dbio.1999.9476
-
Fgf signalling controls diverse aspects of fin regenerationDevelopment 143:2920–2929.https://doi.org/10.1242/dev.140699
-
Identification of distal enhancers for Six2 expression in pronephrosThe International Journal of Developmental Biology 59:241–246.https://doi.org/10.1387/ijdb.140263ho
-
Expression of the LIM class homeobox gene Xlim-1 in pronephros and CNS cell lineages of Xenopus embryos is affected by retinoic acid and exogastrulationDevelopment 120:1525–1536.
-
Xenopus Wnt11b is identified as a potential pronephric inducerDevelopmental Dynamics : An Official Publication of the American Association of Anatomists 239:148–159.https://doi.org/10.1002/dvdy.22012
-
Apoptosis is required during early stages of tail regeneration in Xenopus laevisDevelopmental Biology 301:62–69.https://doi.org/10.1016/j.ydbio.2006.10.048
-
Regulation of the Lim-1 gene is mediated through conserved FAST-1/FoxH1 sites in the first intronDevelopmental Dynamics 225:448–456.https://doi.org/10.1002/dvdy.10176
-
Inducible gene expression in transgenic Xenopus embryosCurrent Biology 10:849–852.https://doi.org/10.1016/S0960-9822(00)00596-0
-
Directed differentiation of human pluripotent cells to ureteric bud kidney progenitor-like cellsNature Cell Biology 15:1507–1515.https://doi.org/10.1038/ncb2872
-
Characterization of mesonephric development and regeneration using transgenic zebrafishAmerican Journal of Physiology-Renal Physiology 299:F1040–F1047.https://doi.org/10.1152/ajprenal.00394.2010
Article and author information
Author details
Funding
Japan Society for the Promotion of Science (16K07362)
- Haruki Ochi
Takeda Science Foundation
- Haruki Ochi
- Hajime Ogino
Suzuken Memorial Foundation
- Haruki Ochi
Japan Society for the Promotion of Science (15K07082)
- Hajime Ogino
Japan Society for the Promotion of Science (16H04794)
- Hajime Ogino
Japan Society for the Promotion of Science (25124704)
- Haruki Ochi
Japan Society for the Promotion of Science (25125716)
- Haruki Ochi
Japan Society for the Promotion of Science (16H04828)
- Haruki Ochi
Japan Society for the Promotion of Science (17KT0049)
- Haruki Ochi
The funders had no role in study design, data collection and interpretation, or the decision to submit the work for publication.
Acknowledgements
This work was supported by Grants-in-Aid for Scientific Research from the Japan Society for the Promotion of Science (JSPS) (Grants nos. 16K07362, 25124704, 25125716, 16H04828, and 17KT0049 to H Ochi and 15K07082 and 16H04794 to H Ogino), as well as grants from CREST (JST to H Ogino), the Takeda Science Foundation (to H Ochi and H Ogino), Suzuken Memorial Foundation, and YU-COE (C) (H Ochi). The genomic DNA of X. tropicalis for the screening enhancer was provided by the Amphibian Research Center, Hiroshima University, with partial support from the National Bio-Resource Project of AMED, Japan.
Ethics
Animal experimentation: This study was performed in strict accordance with the recommendations in the Act on Welfare and Management of Animals in Japan and the Guide for the Care and Use of Laboratory Animals of the National Institutes of Health. All of the animals were handled in accordance with the Animal Research Guidelines at Yamagata University. The protocol was approved by the Committee on the Ethics of Animal Experiments of Yamagata University.
Copyright
© 2019, Suzuki et al.
This article is distributed under the terms of the Creative Commons Attribution License, which permits unrestricted use and redistribution provided that the original author and source are credited.
Metrics
-
- 2,391
- views
-
- 299
- downloads
-
- 26
- citations
Views, downloads and citations are aggregated across all versions of this paper published by eLife.
Citations by DOI
-
- 26
- citations for umbrella DOI https://doi.org/10.7554/eLife.43186