tec-1 kinase negatively regulates regenerative neurogenesis in planarians
Abstract
Negative regulators of adult neurogenesis are of particular interest as targets to enhance neuronal repair, but few have yet been identified. Planarians can regenerate their entire CNS using pluripotent adult stem cells, and this process is robustly regulated to ensure that new neurons are produced in proper abundance. Using a high-throughput pipeline to quantify brain chemosensory neurons, we identify the conserved tyrosine kinase tec-1 as a negative regulator of planarian neuronal regeneration. tec-1RNAi increased the abundance of several CNS and PNS neuron subtypes regenerated or maintained through homeostasis, without affecting body patterning or non-neural cells. Experiments using TUNEL, BrdU, progenitor labeling, and stem cell elimination during regeneration indicate tec-1 limits the survival of newly differentiated neurons. In vertebrates, the Tec kinase family has been studied extensively for roles in immune function, and our results identify a novel role for tec-1 as negative regulator of planarian adult neurogenesis.
Introduction
The capacity for adult neural repair varies across animals and bears a relationship to the extent of adult neurogenesis in the absence of injury. Adult neural proliferation is absent in C. elegans and rare in Drosophila, leaving axon repair as a primary mechanism for healing damage to the CNS and PNS. Mammals undergo adult neurogenesis throughout life, but it is limited to particular brain regions and declines with age (Tanaka and Ferretti, 2009). By contrast, select organisms can undergo more extreme neural repair and also typically undergo more extensive ongoing neural differentiation through adulthood. Axolotls and zebrafish are able to repair extensive damage (Tanaka and Ferretti, 2009), while organisms such as planarians and hydra are able to regenerate an entirely new brain through whole-body regeneration (Tanaka and Reddien, 2011). Based on identification of similar mechanisms of regeneration from the distantly related planarians and acoels, whole body regeneration has been proposed to be an ancestral feature (Gehrke and Srivastava, 2016; Reddien, 2018). Understanding the regulatory mechanisms of adult neurogenesis could therefore reveal methods to enhance neural repair across species. In particular, specific negative regulators of neurogenesis would be important targets for repair enhancement, but few have yet been identified (He and Jin, 2016).
The planarian Schmidtea mediterranea undergoes complete brain regeneration within 1–2 weeks after injury and also perpetual homeostatic replacement of brain tissue, making this species an ideal model to identify such factors. The planarian brain is composed of an anterior bi-lobed cephalic ganglia with axon-rich neuropils containing interneurons and glia, as well as lateral branches with chemosensory, mechanosensory, and other neurons (Agata et al., 1998; Mineta et al., 2003; Nakazawa et al., 2003; Roberts-Galbraith et al., 2016; Wang et al., 2016). Two ventral nerve cords relay signals to the body through a peripheral nervous system, with many neuron subtypes, including serotonergic, GABAergic, dopaminergic, octopaminergic, cholinergic, and glutaminergic neurons (Nishimura et al., 2007a; Nishimura et al., 2007b; Cebrià, 2008; Nishimura et al., 2008a; Nishimura et al., 2008b; Collins et al., 2010; Nishimura et al., 2010). In addition, planarian cell atlas projects recently revealed the existence of ~50 distinct types of neurons, pointing to considerable complexity of regulation for producing and maintaining neurons through ongoing differentiation (Fincher et al., 2018; Plass et al., 2018). Planarian tissues are maintained and regenerated by piwi-1+ neoblast stem cells, a mesenchymal cell population found in a parenchymal region and constituting the animal’s only proliferative cells. Transcriptional profiling and candidate approaches have identified subpopulations of neoblasts specified for participation in distinct tissue lineages including a subpopulation of TSPN+ neoblasts that can give rise to all cell types (Lapan and Reddien, 2011; Cowles et al., 2013; Cowles et al., 2014; Scimone et al., 2014; Brown et al., 2018; Zeng et al., 2018). Neural progenitors have been identified as neoblast subpopulations expressing either pro-neurogenic transcription factors or transcription factors expressed in unique differentiated neurons, and some have been assigned to particular lineages through RNAi (van Wolfswinkel et al., 2014; Molinaro and Pearson, 2016; Fincher et al., 2018; Plass et al., 2018; Ross et al., 2018). Thus, neoblasts and their differentiating progeny sustain ongoing neurogenesis in the absence of injury as well as in regeneration of a new head.
Brain regeneration in planarians is a robust process that always ensures a proper restoration of relative neuron abundance in the animal. Decapitation triggers waves of wound-induced signals, bursts of proliferation, a patterning process to sense missing tissues, and differentiation of new tissues within an outgrowing blastema. Planarians do not grow appreciably as they regenerate, so the end of regeneration is characterized by a return to uninjured cell and tissue proportionality with respect to body size (Oviedo et al., 2003; Hill and Petersen, 2015). In addition, the regeneration process involves re-scaling and integrating pre-existing tissues with new tissues. For example, regenerating head fragments reduce the size of their brain while they form a small tail blastema until they reach appropriate proportionality. The robustness of the regeneration process suggests first that the system is well suited for identifying subtle phenotypes affecting adult neurogenesis. Secondly, the planarian’s ability to attain and maintain a predictable relative abundance of neurons suggests the process is under both positive and negative control.
We report here the identification of a Tec non-receptor tyrosine kinase gene through RNAi screening in planarians that limits neuron abundance in regeneration and homeostatic tissue maintenance. Analysis of tec-1’s mechanism of action reveals it is unlikely to control the process of differentiation, but instead negatively regulates neuron survival. These results suggest that like neural development, both regeneration and stem-cell dependent adult neurogenesis both involve an initial overproduction of new neurons and identify a new factor involved in limiting the specific amplification of cells produced by adult neurogenesis.
Results
tec-1 limits the regeneration of brain neurons
To identify negative regulators of neuronal regeneration in planarians, we conducted an RNAi screen of ~50 kinases and receptors with expression enriched in neoblasts (Supplementary file 1). In order to quantitatively measure effects on neuronal regeneration, we used a histological assay for staining and enumerating chemosensory neurons of the brain expressing the cintillo gene homologous to the Degenerin superfamily of sodium channels (cto, Figure 1A). cto-expressing neurons are arranged in a stereotyped pattern in close proximity to the lateral brain branches, and their numbers scale with overall animal and brain size. Because these cells adopt a planar and well-spread configuration, quantification of whole animal cto cell number through automated image segmentation is straightforward and robust (Oviedo et al., 2003; Hill and Petersen, 2015). Decapitated animals which initially lack cto+ cells regenerate them as they form a new head until an appropriate ratio of cto+ cells to body size is attained. We treated animals with dsRNA and amputated to produce head, tail, and trunk fragments that were fixed and stained in 96-well mesh plates after regeneration. In order to maximize the effects of RNAi, regenerating trunk fragments were amputated again and scored for their ability to regenerate cto+ cells after the second round of regeneration, whereas tail and head fragments were only fixed and scored after a single round of regeneration (Reddien et al., 2005). After imaging, numbers of cto+ cells and total animal area were measured using a CellProfiler pipeline, and differences in relative cto+ cell regeneration due to RNAi treatment were determined by computing a normalized log2-fold change ratio as compared to groups treated with a non-targeting dsRNA (C. elegans unc-22). Treatment with a positive control dsRNA targeting ndk, a factor that restricts head regionalization in planarians (Cebrià et al., 2002), produced increased numbers of cto+ cells in the screen. The screen identified four genes whose inhibition reduced numbers of cto+ cells attained through regeneration (log2FC < −1 and padj <0.05), though several other treatments caused smaller cto+ cell decreases that did not meet the false-discovery corrected statistical cut-off (Supplementary file 2). Because these factors could either be required for expression of cto in mature neurons, be required for differentiation from neural progenitors, or be required for maintenance of neoblasts in general, we did not pursue any further analysis of them here (Figure 1B).
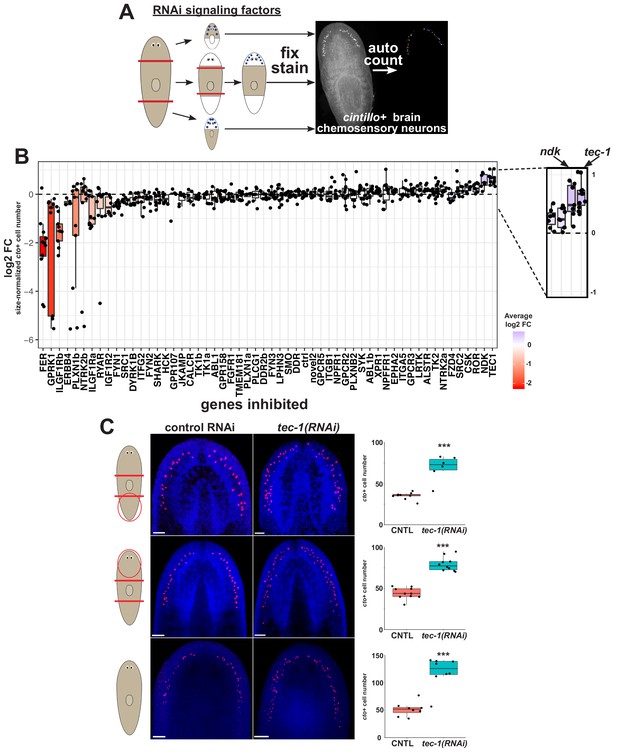
An RNAi screen identifies regulators of neuronal regeneration.
(A) Design of RNAi screen. Animals were fed over several weeks, challenged to regenerate after head and tail amputation, and then fixed and stained for cto expression using Hoechst as a counterstain to detect total animal tissue (heads and tail fragments fixed at d23, while and regenerating trunks were fed dsRNA at d10 then amputated again the following day and then fixed 12 days later). Numbers of cto+ cells were enumerated using CellProfiler and normalized to animal size as measured by Hoechst area in CellProfiler. (B) Log2-transformed fold changes of area-normalized cto-cell number were determined by comparison to similar fragments treated with control dsRNA, combined across head, trunk and tail fragment types for each dsRNA treatment and plotted in ascending order of average log2-fold change. Dots represent log2-fold change for each regenerating fragment, with boxplot shading representing average log2-fold change compared to controls. Knockdown of tec-1 increased cto+ cell number more than nou-darake positive control on average. Dotted line indicates log2FC for control RNAi conditions. (C) To measure tec-1 RNAi’s effect on head regeneration and injury-induced remodeling, animals were fed dsRNA for 2 weeks before decapitation and allowed to regenerate for 16 days. To measure the effects of tec-1 inhibition in homeostasis, animals were fed dsRNA for 60 days and fixed without injury. An increase in cto+ cell number was detected in all contexts. ***p<0.001 by two-tailed t-test. Scale bars: 100 μm.
We sought to uncover factors that negatively regulated cto+ cell production and found one factor, a Tec-family kinase (tec-1), whose inhibition caused a statistically significant increase in abundance of cto+ cells (Figure 1B, Figure 1—figure supplement 1, Figure 1—figure supplement 2, Supplementary file 2). To verify these effects, we inhibited tec-1 in a similar design as the screen but with an increased sample size. These experiments confirmed that inhibition of the Tec homolog resulted in a robust ~50–100% increase to the number of cto+ cell numbers, which had approximately normal spatial distribution in the animal (Figure 1C). tec-1 knockdown led to an increase in cto+ cell number both in decapitated animals regenerating an entirely new head and also in head fragments undergoing tissue remodeling (Figure 1C). This increase in relative cto+ cell abundance was not due to a change in overall animal size, but rather to an increase in absolute numbers of cto+ cells (Figure 1—figure supplement 3). Regeneration of head and tail fragments involves extensive stem cell-dependent production of new brain neurons and control of the rates of cell death, as well as injury-induced signals that initiate the process. Uninjured planarians undergo perpetual homeostatic regeneration of all cell types, including neurons of the brain, but lack expression of injury induced factors. In order to test whether tec-1’s function depended on wound signaling, we fed animals tec-1 dsRNA for 60 days in the absence of injury and found that cto+ cell number increased to a similar extent as in amputated fragments (Figure 1C). Therefore, tec-1 functions to limit numbers of neurons independent of injury signaling.
To examine a possible redundancy of function, we scanned the Schmidtea mediterranea genome and transcriptome and identified a second Tec family kinase, which we named tec-2 (Figure 1—figure supplement 2). Inhibition of tec-2 by RNAi did not increase cto+ cell numbers or enhance the effects of tec-1 RNAi (Figure 1—figure supplement 4). Therefore, tec-2 likely does not act redundantly with tec-1 for control of cto+ cell abundance.
RNAi phenotypes that modify body patterning (such as nou darake RNAi) can result in production of excess cto+ cells as a consequence of axis transformation (Gurley et al., 2008; Iglesias et al., 2008; Petersen and Reddien, 2008; Lander and Petersen, 2016; Scimone et al., 2016), so we tested whether tec-1 could operate similarly. Whereas ndk inhibition increased cto+ cell number concomitant with increasing the size of the brain (Cebrià et al., 2002), tec-1 inhibition did not alter brain size (Figure 2A). Instead, tec-1 RNAi, but not ndk RNAi, significantly increased the number of cto+ cells normalized to brain size. The observation that tec-1 inhibition increased numbers of cto+ neurons without altering the relative size of the head or brain raised the question of how such animals physically pack increased neurons. To clarify how planarian brains could increase the number of cells without altering their overall size, we mapped the 3D positions and sizes of cto+ cells in control versus tec-1(RNAi) animals, and determined their nearest-neighbor distances and cell body volumes using Fiji/ImageJ (Figure 2—figure supplement 1). tec-1 inhibition decreased the average nearest-neighbor distance of cto+ cells and also the average volume of each cell as measured by cto mRNA distribution. Therefore, tec-1 influences cto+ cell abundance and size.
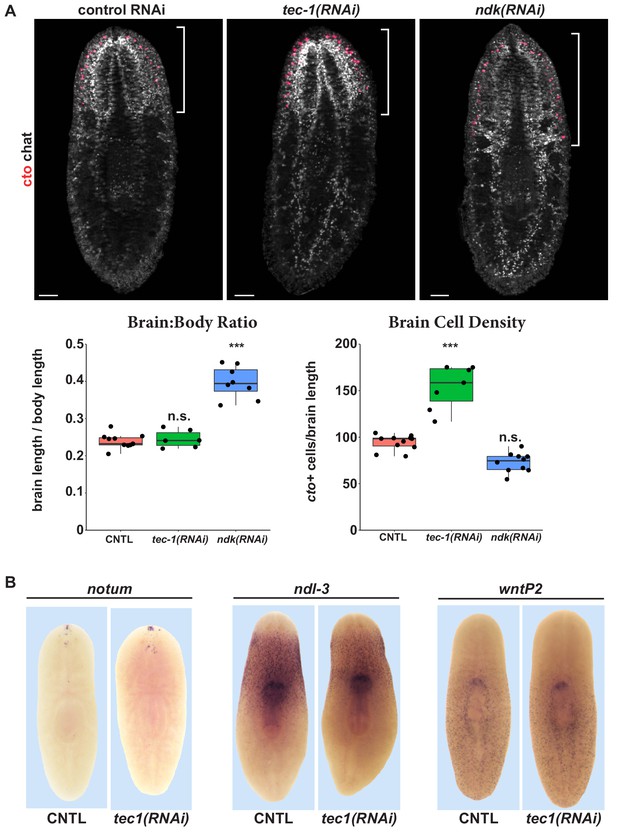
tec-1 inhibition increases chemosensory neuron abundance but not head regionalization.
(A) Animals were fed dsRNA for 2 weeks, amputated to remove heads and tails, and allowed to regenerate for 16 days. FISH was performed on trunk fragments to simultaneously measure cto+ chemosensory neurons and chat+ cholinergic neurons that allow visualization of the brain. Effects on the brain:body size and density of cto+ cells normalized to brain size were quantified. ndk RNAi resulted in an increase in brain:body size but not in numbers of cto+ cells normalized to brain size. By contrast, tec-1 RNAi increased density of cto+ cells within the brain without increasing the proportion of the body axis occupied by the brain. (B) tec-1 RNAi did not strongly affect the expression of anterior-posterior positional control genes notum, ndl-3, and wntP-2 as measured by WISH (images representative for 27/27 animals probed). Scale bars: 100 μm.
To confirm a lack of overall changes to body regionalization in tec-1(RNAi) animals, we examined positional control genes whose expression demarcates distinct body territories. ndl-3 and wntP-2 expression was not appreciably altered in tec-1 RNAi treatments that result in excess cto+ cells (Figure 2B). By WISH, tec-1 inhibition was observed to modestly alter the expression of the anteriorly expressed gene notum, but through FISH analysis this effect could be attributed to the expansion of a population of previously identified notum+chat+ neurons present in the anterior of the brain (Figure 2B, Figure 2—figure supplement 2). (Hill and Petersen, 2015). We conclude that tec-1 limits numbers of cto+ cells by altering neuron density rather than participating strongly in body patterning, and that tec-1 regulates the abundance of more than one type of neuron.
tec-1 negatively regulates the abundance of many neural cell types
In order to test the specificity tec-1’s function to limit differentiated cell abundance, we investigated the impact of tec-1 knockdown on other neural cell types whose abundances could be quantified with high precision. We began by examining neurons expressed more medially within the brain compared to chemosensory neurons. glutamic acid decarboxylase (gad) is expressed in GABAergic neurons in the ventral-medial and dorsal-lateral CNS (Nishimura et al., 2008b). Neurons in these domains have distinct progenitor populations expressing nkx2.1 and tcf1 respectively (Currie et al., 2016; Brown et al., 2018). Inhibition of tec-1 increased the total number of GABAergic cells and also numbers of cells within both domains (Figure 3A and Figure 3—figure supplement 1), suggesting that tec-1’s activity is not restricted to a single brain region or a specific neuronal lineage. To confirm this, we quantified cells found throughout the brain which expresses the neuropeptide precursor-encoding gene pyrokinin prohormone-like 1 (ppl-1) (Collins et al., 2010). Cephalic ppl-1+ cells increased in abundance after tec-1 knockdown (Figure 3A). ppl-1 is also expressed prominently in neurons of the pharynx, but these cells were unaffected by tec-1 RNAi (Figure 3B), indicating tec-1 does not regulate abundance of all neurons throughout the animal. We additionally examined serotonergic neurons in the CNS marked by serotonin transporter (sert), which have a defined progenitor cell type (Currie and Pearson, 2013; März et al., 2013), and dd17258+ neurons expressed in a domain similar to cto+ cells (Fincher et al., 2018). We observed that the numbers of both of these populations increased upon tec-1 inhibition. To test whether tec-1 might act exclusively within the CNS, we quantified the density of nociceptory trpA+ neurons (Wenemoser et al., 2012; Arenas et al., 2017) and peripheral cholinergic chat+ neurons (Nishimura et al., 2010) of the in the PNS and found that tec-1 RNAi increased the abundance of both cell types (Figure 3A). To obtain more information on the effects of tec-1 knockdown throughout the body axis, we also sought neuron cell types distributed throughout the body and with densities and abundances amenable to whole-animal enumeration. We selected four such cell types that had been identified in a prior scRNAseq cell atlas study (Figure 3—figure supplement 2) (Collins et al., 2010; Fincher et al., 2018). tec-1 knockdown increased the density of three of these cell types: dd2223+, dd3733+, and spp-4+ neurons. However, tec-1 knockdown did not affect the abundance of dd2723+ neurons, which are expressed in the CNS and pharynx (Figure 3—figure supplement 2A). In addition, we also examined photoreceptor neuron abundance, because tec-1(RNAi) animals regenerating a new head sometimes produced disorganized eyes (Figure 3B). Despite affecting eye morphology, tec-1 inhibition did not modify the number of photoreceptor neurons. Together, we found that tec-1 inhibition increased the abundance of 10 of 13 neuron markers investigated, indicating that tec-1 negatively regulates the abundance of many but not all types of neurons within the central and peripheral nervous systems.
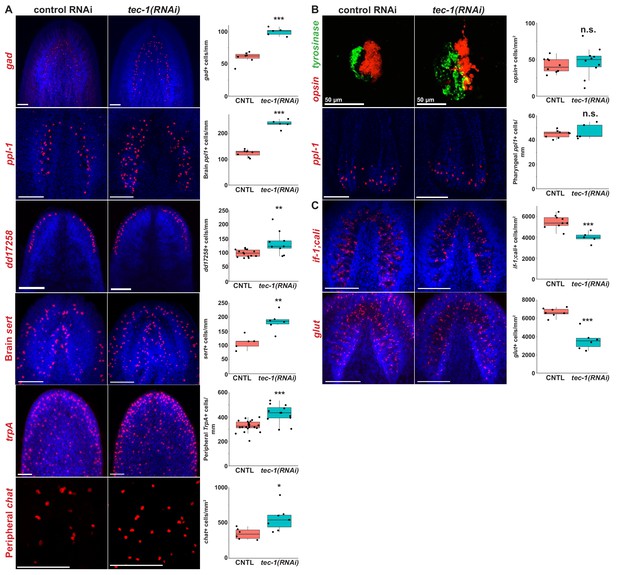
tec-1 inhibition increases abundance of several CNS and PNS neurons.
(A-C) Animals were fed dsRNA for two weeks, amputated to remove heads and tails, and allowed to regenerate for 15 days. Regenerating head fragments were fixed and stained for gad expression, regenerating tail fragments were stained for ppl-1, trpA, opsin/tyrosinase, glut, or if-1/cali expression, and regenerating trunks were stained for chat or dd17258 expression. Cell types amenable to total animal enumeration were quantified by normalizing cell number to body size by dividing by the square root of whole animal area (gad+, brain and pharynx ppl1+, brain sert+, dd17258+, opsin+ cells). Abundances of cell types too numerous for whole-body counting were quantified by manually scoring cell numbers in a region of interest and normalizing to the area of that region (trpA+ cells were scored in an anterolateral region, peripheral chat+ neurons scored in a postpharyngeal ventromedial region, if-1+;cali, and glut+ cells scored within the brain defined by Hoechst staining). (A) tec-1 RNAi animals had increased numbers of gad+ neurons, ppl-1+ neurons within the brain, dd17258+ neurons, sert+ brain neurons, trpA+ peripheral neurons, and chat+ peripheral neurons. (B) tec-1(RNAi) animals regenerated disorganized photoreceptors but had no alteration in opsin+ cell numbers. Likewise, tec-1 inhibition did not alter numbers of pharyngeal ppl-1+ neurons. (C) tec-1 knockdown decreased the density of glut+ and pooled if-1/cali+ glial cells in the brain. Significance determined by two-tailed t-tests (*, p<0.05; ***, p<0.001; n.s. p>0.05). Images show Hoechst-stained maximum projections except for maximum-projected pharyngeal ppl-1+ cells shown for clarity overlayed with a single slice of Hoechst-labeled pharynx tissue. Scale bars: 100 μm unless otherwise noted.
Because many of these effects were identified in brain-localized, anterior cell types, we wanted to understand whether the tec-1 RNAi phenotype only affected anterior cells. Planarian proliferative cells have differential sensitivities to DNA damage repair and cell death across the A-P axis (Peiris et al., 2016). To test a potential influence of axis position on the tec-1 RNAi phenotype, we determined the A-P position of all dd2223+ cells in control and tec-1 RNAi animals and binned these into anterior and posterior regions (Figure 3—figure supplement 2B). tec-1 inhibition increased the abundance of dd2223+ neurons in both the anterior and posterior of the animal. Furthermore, tec-1 RNAi did not alter the relative proportion of anterior versus posterior dd2223+ neurons. Together, we conclude that tec-1 can act equivalently on neurons independent of body position.
We also wanted to determine whether tec-1 exerts its influence equally on distinct cell types. To test this, we stained tec-1(RNAi) and control animals simultaneously for cto and either gad or ppl-1, which are expressed in different areas of the brain. Counting two neuron populations at once allowed direct comparisons of the tec-1 RNAi phenotype’s expressivity for each animal. tec-1 inhibition increased numbers of these neuron cell types to the same extent without affecting gad:cto or ppl1:cto cell ratios (Figure 3—figure supplement 3). Therefore, tec-1 appears to affect multiple neuron type abundances concordantly.
We next sought to determine whether excess neurons produced in tec-1(RNAi) animals had features of normal neurons. We noticed that riboprobes detecting cto mRNA primarily stained the cell body, but high magnification imaging revealed some detection of signal in processes extending laterally from these bodies, putatively related to axonal or dendritic regions. A similar proportion of cto+ cells had such processes in control versus tec-1(RNAi) animals (Figure 3—figure supplement 4A). We also used alpha-tubulin staining to examine overall innervation patterns. In this assay, tec-1(RNAi) animals displayed a qualitative increase in size of nerve bundles, particularly evident in the transverse commissural fibers joining the nerve cords along the ventral side of the animal (Figure 3—figure supplement 4B, 6/7 animals examined). Treatments disrupting synaptic transmission can cause failure of neuron regeneration in planarians (Inoue et al., 2007), suggesting that the excess neurons in such animals may be functional.
We reasoned that tec-1 might control cell abundance specifically within a subset of CNS and PNS neurons or more generally throughout the animal. Neither collagen+ body wall muscle cells nor epidermal nuclei were found to have statistically significant changes in density after tec-1 inhibition (Figure 3—figure supplement 5A), suggesting the specificity of tec-1’s cell number control activity to the nervous system. We also examined animals stained for broad markers of excretory and intestinal tissue (Figure 3—figure supplement 5B). There were not obvious increases in expression of either marker of these tissue types.
In addition, we also examined non-neuronal cells in the CNS characterized as astrocyte-like glial cells (Roberts-Galbraith et al., 2016; Wang et al., 2016). tec-1 inhibition decreased the density of cells expressing pooled glial markers intermediate filament 1 (if-1) and calamari (cali) as well as those expressing glucose transporter (glut) (Figure 3C). The functions for planarian glial cells are not yet characterized, but these cells could have a role in neuron surveillance.
tec-1 suppresses neuronal cell number by regulating cell survival
We next sought to determine how tec-1 exerts its negative regulatory function on neuron abundance. Differentiated cells are produced continually from neoblasts in adult planarians, so that increases to numbers of differentiated cells could either arise from an increase in stem cell-dependent tissue production or through decreases in the death of differentiated cells. Because tec-1 knockdown broadly increased neuronal numbers while decreasing glial numbers, and it is known that neurons and glial cells can arise from common progenitor cells in some contexts in other organisms, we initially hypothesized that tec-1 might control a differentiation switch at some early point in neural specification (Mori et al., 2005; Homem and Knoblich, 2012).
To better understand the dynamics of tec-1’s effects on regeneration, we measured cto+ cell abundance over time during head regeneration after decapitation and during remodeling of head fragments (Figure 4A). In decapitated tec-1(RNAi) animals regenerating a new head, the rate of production of new cto+ cells was not altered at early times (4 days), but abundance of cto+ cells was higher after a week of regeneration (8 days) and reached a maximum point at 16 days. We also confirmed through WISH that tec-1 is expressed broadly in the animal and throughout regeneration from day 2 to day 12 post-amputation without undergoing discernable regeneration-induced expression changes (Figure 4—figure supplement 1). Given this expression and that tec-1 affects neuron density in both regeneration and homeostasis, it is likely this gene functions constitutively to regulate neuron numbers. To test for the perdurance of the phenotype in regeneration, we measured cto+ cell number up to 4 weeks after decapitation and found that cto cells had reached a steady-state maximum by two weeks post-amputation (Figure 4—figure supplement 2). In tec-1(RNAi) head fragments undergoing tissue remodeling, the maximal cto+ cell abundance phenotype was observed somewhat earlier, by 4 days, and persisted (Figure 4A). The progressive nature of the phenotype in decapitated regenerating animals suggests that tec-1 is unlikely to regulate rates of differentiation commonly across all timescales and conditions. However, it remained possible that rates of neuron differentiation are maximal during early head regeneration, while tec-1 could restrict rates of differentiation in a process common to the contexts of late head regeneration, head remodeling, and homeostatic maintenance.
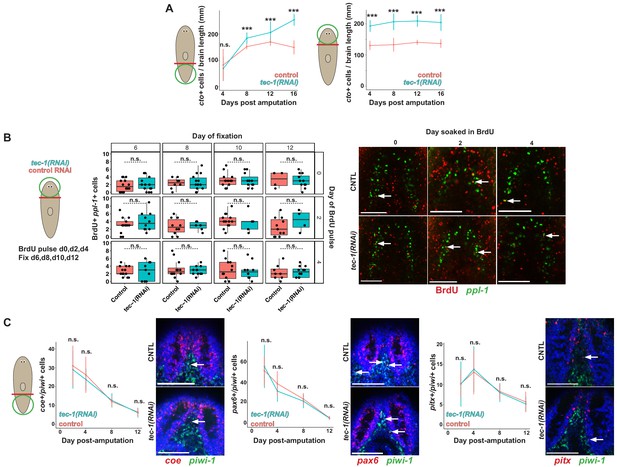
tec-1 inhibition does not increase rates of neuron differentiation.
All animals were fed dsRNA for 2 weeks before surgeries as indicated by cartoons. (A) Time courses of cto+ cell number in regeneration of a new head (left) or remodeling of a pre-existing head (right). Each data point represents sample size of between 4 and 12 animals. (B) Animals were transtioned into high-salt water one week before surgery, and either whole animals (day 0) or head fragments (days 2 and 4) were soaked in BrdU for 4 hr. Heads fragments were fixed at days 6, 8, 10, and 12 post-amputation. Co-staining of control versus tec-1(RNAi) animals for ppl-1 mRNA and BrdU showed no detectable increase in numbers of BrdU+ppl-1+ cells at any time point (left). Single confocal slices show staining of BrdU and ppl-1 in day 12 animals (right). White arrows show colocalization. (C) Regenerating tail fragments were fixed at 2, 4, 8, or 12 days post injury and stained with FISH to detect piwi-1 and coe or pax6 transcription factors, which label broad neuronal progenitors, or pitx transcription factor, labeling progenitors of serotonergic neurons. Single confocal slices show staining of transcription factors, piwi-1, and Hoechst counterstain in day 12 animals. No significant differences in numbers of neural progenitor cells were detected (***, p<0.001, n.s. p>0.05 by two-tailed t-test, error bars represent standard deviation). Scale bars: 100 μm.
To test this possibility, we designed a BrdU labeling strategy to test for possible effects of tec-1 inhibition on the rate of neuron differentiation. We chose to investigate regenerating head fragments undergoing brain remodeling because tec-1 inhibition caused a rapid attainment of excess neurons compared to control animals, so we reasoned these fragments would provide a context in which tec-1-dependent regulation is prominent. Neoblasts are the only proliferative cells in adult planarians, so a pulse of BrdU initially marks these cells, followed by labeling their newly-born post-mitotic descendants (Newmark and Sánchez Alvarado, 2000). In order to measure rates of neuron production, we soaked animals with BrdU earlier on same the day of amputation (d0) or at 2 or 4 days after amputations to generate head fragments, then fixed the animals at 6, 8, 10 and 12 days post-amputation in order to detect BrdU incorporation into new mature neurons (Figure 4B). We chose to analyze BrdU incorporation into ppl-1+ brain neurons because they are under robust control by tec-1 (Figure 3A), and they are more numerous than cell types such as cto+ or gad+ neurons, thus maximizing the ability to detect any influence of tec-1 on neuron differentiation given possible inefficiencies in BrdU label uptake. However, numbers of ppl-1+BrdU+ cells were not significantly different in control versus tec-1(RNAi) animals over a range of timepoints of BrdU pulsing and fixation in regeneration. These data indicate that tec-1 inhibition can increase numbers of neurons without modifying rates of differentiation, pointing instead to a function in controlling neuron survival.
A model in which tec-1 controls neuronal survival would predict that tec-1 does not influence the abundance of neural progenitor cells that are the intermediates between pluripotent neoblast stem cells and differentiated neurons (Figure 4C). coe encodes a transcription factor expressed in a subpopulation of piwi-1+ neoblasts as well as in a variety of differentiated neurons, and its inhibition reduces the abundance of several types of neurons, including gad+ and cto+ neurons (Cowles et al., 2013; Cowles et al., 2014). tec-1 inhibition did not alter numbers of coe+piwi-1+ cells in a timeseries between 2 and 12 days of head regeneration, despite detection of an overall decreasing abundance of such progenitors in both control and tec-1(RNAi) animals over time as regeneration proceeds. To confirm these observations, we examined a subpopulation of piwi-1+ neoblasts expressing the proneural transcription factor pax6 (Scimone et al., 2014) and found that its abundance was also unaltered by tec-1 inhibition in the same time period. coe and pax6 mark broad classes of neural progenitors, so we also sought to test the abundance of neural progenitors that produce individual neural cell types. pitx transcription factor is required for production of sert+ serotonergic neurons and pitx is expressed within a piwi-1+ neoblast subpopulation proposed to be the progenitors for these cells. Although tec-1 inhibition increased the abundance of sert+ neurons in the brain (Figure 3A), tec-1 RNAi did not alter numbers of pitx+piwi-1+ serotonergic neuron progenitors near the brain (Figure 4C).
We next examined tec-1 expression by double-FISH to determine whether this gene could be expressed preferentially within neurons, neural progenitors, or other cell types. However, we detected tec-1 FISH signal not only within each type of neuron tested (cto, gad, ppl-1) but also within pitx, coe, or pax6A-expressing cells, neoblasts or their differentiating progeny marked with anti-PIWI-1 antibody, and in if-1;cali+ glial cells (Figure 4—figure supplement 3). Therefore, tec-1 is a broadly expressed gene that exerts a specific effect on neuronal cell density. Consistent with these observations and the specificity of the tec-1(RNAi) phenotype, tec-1 inhibition did not modify numbers of H3P+ mitotically active cells in response to injury (Figure 4—figure supplement 4). Taken together, these results support the model that tec-1 does not regulate the process of neuronal differentiation or control a switch between neuronal and glial specification.
In light of these results, we reasoned that tec-1 might function to activate cell death specifically of CNS and PNS neurons. Uninjured planarians undergo a basal rate of homeostatic cell death thought to occur across tissue types as older cells die off and require stem cell-dependent replacement, and amputation triggers a systemic elevation of cell death of differentiated cells as part of the tissue remodeling process (Pellettieri et al., 2010). We used TUNEL staining on remodeling head fragments to test whether tec-1 promotes cell death and found that tec-1 RNAi reduced but did not eliminate numbers of TUNEL+ cells (Figure 5A). This observation is consistent with a model in which tec-1 promotes cell death of some but not all cells.
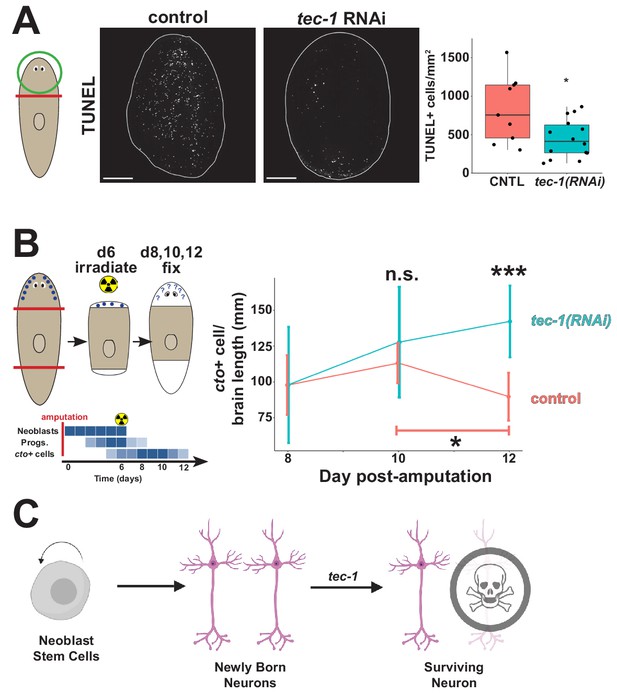
tec-1 promotes cell death and limits survival of new neurons.
Animals were fed dsRNA for 2 weeks before amputation to measure numbers of dying cells and persistence of regenerated chemosensory neurons after lethal irradiation. (A) Head fragments were TUNEL stained 72 hr after injury and numbers of TUNEL+ cells normalized to fragment area quantified to find that tec-1 inhibition resulted in diminished numbers of dying cells. (B) Amputated trunk fragments were allowed to regenerate for 6 days, treated with 6000 rads of X-rays to eliminate neoblasts and subsequent neuron differentiation, then fixed at the indicated times post-injury, and numbers of cto+ cells detected and counted by FISH and normalized to brain length determined by Hoechst staining. Schematic shows predicted effects of lethal irradiation on population abundances (blue shading) for cells involved in producing new cto+ neurons in head regeneration: neoblasts, neural progenitors, and differentiated CNS neurons after irradiation during head regeneration over the course of days. In control animals, the density of cto+ neurons (red) decreased between four and six days after irradiation (bracket). By contrast, tec-1(RNAi) animals had normal numbers of newly formed cintillo+ cells, cto+ number is not significantly greater than controls at d10 post amputation, but is significantly greater than controls two days later. Each data point represents sample size of between 4 and 9 animals. (C) Model of normal neuronal production, where tec-1 acts to cull excess neurons in homeostasis and regeneration (*p<0.05, **p<0.01, ***p<0.001, n.s. p>0.05 by two-tailed t-test, error bars represent standard deviation). Scale bars: 100 μm.
We hypothesized based on the above results that tec-1 might promote cell death in a fraction of newly born neurons. The requirement of tec-1 under conditions of both homeostasis and regeneration would then suggest that, as in embryonic development in other organisms, regenerated brain neurons in planarians undergo an initial overproduction followed by rapid cell death. The regeneration of a new head in decapitated animals represented a condition in which we reasoned this phenomenon would be most easily detected, because in that context the new production of brain neurons is synchronized by amputation. However, detailed time course analysis of new neuron production in such animals has not yet identified such overproduction, suggesting that ongoing neuron production during the ~1–2 weeks of head regeneration might mask these effects. Therefore, we sought a means to isolate the fates of a limited cohort of neurons produced only within a narrow time window after amputation. To do this, we used lethal irradiation early during head regeneration to eliminate neoblasts acutely, followed by a timeseries of fixation and staining, to measure the survival of a cohort of neurons produced only within in a specific time frame. After tec-1 inhibition and head amputation, planarian fragments were irradiated 6 days later then fixed in a time series in the absence of proliferating cells. Staining these animals with a piwi-1 riboprobe 2 days after irradiation showed complete elimination of neoblasts by this time (Figure 5—figure supplement 1). In control animals, cto+ cell number decreased between days 10 and 12 post-amputation, consistent with the prediction of initial overproduction (Figure 5B and Figure 5—figure supplement 2). tec-1(RNAi) animals did not produce significantly more cto+ cells at day 10 compared to controls but did not undergo the day 12 decrease in cto+ cells. These data confirm that tec-1 does not influence rates of cell production but instead strongly suggest that tec-1 promotes the death of newly born neurons in order to limit the abundance of cells produced through adult neurogenesis (Figure 5C).
Discussion
Here we identify the conserved gene tec-1 as a potent and novel negative regulator of stem cell-dependent adult neurogenesis in Schmidtea mediterranea. Completion of regeneration and homeostatic maintenance requires control of both the rates of new cell differentiation and also control of cell survival (LoCascio et al., 2017). Several positively acting factors contribute to differentiation of new neurons in planarians. Injuries trigger expression of the runt-1 transcription factor within neoblasts, required for producing normal numbers of trpA+ brain cells, eyes, and other cells. Other fate determinants appear to act constitutively to control differentiation or expression within distinct neuronal populations (Wenemoser et al., 2012; Cowles et al., 2013; Currie and Pearson, 2013; März et al., 2013; Scimone et al., 2014; van Wolfswinkel et al., 2014; Currie et al., 2016; Molinaro and Pearson, 2016; Brown et al., 2018; Fincher et al., 2018).
By contrast, fewer regulators of cell death have been identified in planarians and these appear to regulate multiple lineages. Inhibition of bcl2-1, a conserved antiapoptotic factor, causes a rapid increase in systemic cell death and subsequent animal lysis (Pellettieri et al., 2010). jnk and yorkie inhibition both decrease cell death and increase numbers of cto+ cells relative to overall body size in decapitated head fragments (Almuedo-Castillo et al., 2014; Lin and Pearson, 2014). However, in both of these phenotypes, an increase in neuronal number is linked to an increase in overall brain size and is in a context in which the brain normally undergoes reduction through injury-induced remodeling. Based on tec-1’s specificity for neural cells and utilization in both regeneration and homeostasis, we suggest it operates in a distinct pathway (Figure 5C).
We envision at least two possible mechanisms by which tec-1 facilitates neuronal cell death based on differing sites of action. tec-1 could act cell autonomously within neurons to promote cell death or alternatively could be necessary within a distinct cell population involved in neuron killing and/or engulfment. The broad expression of tec-1 throughout multiple tissues would be consistent with either possibility (Figure 4—figure supplement 3). Cell atlas projects also found that tec-1 is expressed broadly but is moderately enriched in neural progenitors, consistent with the first possibility, but also within intestine and cathepsin+ cells that could have uncharacterized roles in neuron homeostasis (Figure 5—figure supplement 3) (Fincher et al., 2018; Plass et al., 2018). Future work delineating tec-1 signaling targets could clarify this aspect of tec-1’s anti-survival function.
Tec family kinases (TFKs) are non-receptor tyrosine kinases similar to Src family kinases. TFKs are perhaps best known for signaling downstream of antigen receptors in hematopoietic differentiation and lymphomas (Berg et al., 2005; Rickert, 2013). However, they are also involved in a wide variety of signaling pathways downstream of GPCRs, integrins, and both receptor and non-receptor tyrosine kinases, and are known to regulate apoptosis, cell adhesion, and the actin cytoskeleton in addition to differentiation (Takesono et al., 2002). In mammals, a majority of studies have found that Tec kinases such as BTK are essential for the survival or proliferation of immune cells, including phagocytic cells (Takesono et al., 2004; Jongstra-Bilen et al., 2008; Melcher et al., 2008; Palmer et al., 2008), suggesting that planarian tec-1 might not act cell autonomously within neurons to promote death. Alternatively, in some contexts, Tec kinases have been reported to function as cell autonomous tumor suppressor genes that promote p53-mediated cell death pathways (Rada et al., 2018). Within the mammalian brain, chemical inhibition of the Tec-family kinase BTK increased brain cell survival after ischemic brain injury, an effect attributed to the modification of macrophage activity (Ito et al., 2015). It is possible that Tec kinases could regulate conserved functions in adult neurogenesis to control of neuron abundance in other organisms.
The requirement for tec-1 for neuron suppression in regeneration and homeostasis in planarians additionally suggests that developmental cell death of neurons might be a common feature of adult neurogenesis across species. Cell death is extensively used throughout the animal kingdom to sculpt the developing nervous system, from nematodes to mammals (Yamaguchi and Miura, 2015). Among well-studied invertebrates, adult neurogenesis has been detected in Drosophila within the medulla cortex, where it can also mediate injury repair (Fernández-Hernández et al., 2013). However, the bulk of neurogenesis in C. elegans and Drosophila occurs at embryonic or larval stages prior to adulthood, so the precise roles of cell death for adult neurogenesis and ways they may be conserved across distant phyla have yet to be fully elucidated. Based on TUNEL staining and the observation that mouse mutants defective for cell death in the nervous system overproduce neurons in multiple regions of the brain, developmental cell death of neurons is integral to adult neurogenesis in mammals (Biebl et al., 2000; Winner et al., 2002; Sun et al., 2004), and cell death after differentiation has been confirmed through direct observation (Pilz et al., 2018). Regulation of cell death at the level of neural progenitors may control brain morphology by preventing hyperplasia or may define where crucial morphogenic signals are expressed (Haydar et al., 1999; Nonomura et al., 2013; Yamaguchi and Miura, 2015), while regulation of the survival of newly formed neurons has been proposed to provide mechanisms to ensure proper connectivity or limit interneuron cell number (Southwell et al., 2012; Dekkers et al., 2013). During brain development, preventing neurotransmitter secretion results in assembly of a mostly normal brain followed by massive neuronal apoptosis, suggesting that regulated cell death plays a role in the maturation of newly-differentiated neurons during embryogenesis (Verhage et al., 2000). Reliance on neurotransmitter signaling to promote survival of immature neurons is also used extensively in murine adult neurogenesis (Petreanu and Alvarez-Buylla, 2002), among other cell-type specific mechanisms (Pfisterer and Khodosevich, 2017). Many of these pathways converge on promoting survival through BCL2 family members, while death is promoted by Bax and Bak (Motoyama et al., 1995; Shindler et al., 1997; Sun et al., 2004; Savitt et al., 2005; Nakamura et al., 2016). Therefore, inhibitors of core cell death pathways have been proposed as therapeutic agents to assist in damage repair after brain injury (Degterev et al., 2005; Loane and Faden, 2010), though these would be expected to have substantial effects on other organ systems. If the ability of Tec kinases to limit neuron production ultimately proves to be conserved, they would be potential targets for enhancing neural repair in other species.
The identification of negative regulators of neurogenesis may be an important step in understanding neural regeneration in planarians and other systems. Suppressors of mTOR and JAK/STAT signaling in vertebrates can be inhibited to increase axon growth after spinal cord injury in laboratory conditions (Park et al., 2008; Liu et al., 2010; Sun et al., 2011). The JAK/STAT inhibitors Socs3 and Sfpq attenuate axonal regrowth in the optic nerve of mice and zebrafish (Smith et al., 2009; Sun et al., 2011; Elsaeidi et al., 2014). Additionally, while canonical Wnt signaling appears to promote neural regeneration in vertebrates (Yin et al., 2008; Strand et al., 2016; Patel et al., 2017), non-canonical Wnt signaling inhibits axon guidance and growth (Onishi et al., 2014). A more complete understanding of negative inputs into adult tissue homeostasis could provide new and more specific targets for the enhancement of neural tissue repair or treatment of degenerative disease (Trounson and McDonald, 2015). Our results suggest that planarians, which undergo extensive homeostasis of the CNS in adulthood, can be a model for efficiently identifying such negative regulators of adult neurogenesis.
Materials and methods
Reagent type (species) or resource | Designation | Source or reference | Identifiers | Additional information |
---|---|---|---|---|
Gene (Schmidtea mediterranea) | Tec-1 | Planmine | dd_Smed_v6_4818_0_1 | |
Antibody | Polyclonal rabbit anti-digoxigenin-POD, Fab fragments | Sigma/Roche | # 11207733910 RRID: AB_514500 | 1:2000 dilution |
Antibody | Polyclonal rabbit anti-fluorescein-POD, Fab fragments | Sigma/Roche | #11426346910 RRID: AB_840257 | 1:2000 dilution |
Antibody | Polyclonal rabbit digoxigenin-AP | Sigma/Roche | #11093274910 | 1:4000 dilution |
Antibody | Rat polyclonal Anti-BrdU | Abcam | 6326 | 1:1000 dilution |
Antibody | Rabbit monoclonal anti-phospho-ser10 Histone H3 | Cell Signaling | D2C8 | 1:3000 dilution |
Antibody | Mouse monoclonal anti-TUBULIN-ALPHA AB-2 | Thermo/Fisher | MS581P1 | 1:1000 dilution |
Commercial assay or kit | TUNEL labeling kit | Thermo/Fisher | EP0162 | N/A |
Antibody | Goat polyclonal anti-rat HRP | Jackson ImmunoResearch | 112-036-072 | 1:1000 dilution |
Planarian culture
Request a detailed protocolAsexual strain CIW4 of the planarian Schmidtea mediterranea were maintained in 1 × Montjuic salts at 19°C as described (Petersen and Reddien, 2011). Animals were fed a liver paste and starved for at least 7 days before experiments.
Screen
Request a detailed protocol60 putative regulatory molecules were selected by analysis of the planarian transcriptome to identify a set with expression detected in neoblasts after FACS sorting (Labbé et al., 2012), (into populations of X1 neoblasts in G2/S/M phases, X2 cells comprised of a mixture of G1 neoblasts and G0 newly postmitotic progenitor immediate descendants of neoblasts or other irradiation-sensitive G1/G0 cells, and Xins differentiated cells) with either X1/Xins or X2/Xins expression greater than two or either X1 or X2 FPKM >3. Genes classified through blastx or panther as receptor tyrosine kinases, protein tyrosine kinases, integrins, GPCRs, or other signaling factors were prioritized. dsRNA was generated through reverse transcription and two rounds of nested PCR with primers indicated (Table S1) and further amplified to add T7 sites for dsRNA production. dsRNA was produced by T7 in vitro transcription, annealing and purification by ethanol precipitation and added to liver as described previously. Animals were fed three times over a week and amputated to remove heads and tails. Head and tail fragments were fixed at 23 days following amputation (‘A-score’ heads and tails), while trunk fragments were fed again at d10 and amputated to remove heads and tails then regenerating trunk fragments were fixed 12 days later (‘B score’ trunks). Fixations, fluorescence in situ hybridizations, and Hoechst stainings to detect cto expression were carried out as described previously but in 96-well mesh-bottomed plates (Milipore Multiscreen Plates, MANMN4010) incubating in either Multiscreen receiver plates or rectangular 1-well dishes (VWR 73521–420). Stained animals were mounted and imaged at 40x using a fluorescence dissecting microscope to obtain a view of cto expression within the head and also at 25x to obtain a Hoechst-stained view of the entire fragment. A CellProfiler pipeline for automated image analysis was developed to quantify numbers of cto+ cells and also to measure area of the fragment as detected by Hoechst staining. Automated cell counting was optimized by adjusting the fixed detection threshold and object size range. Processed images were manually inspected for proper segmentation and scored manually in the event of visible errors in automated counting. Numbers of cto+ cells scale with animal length, so we normalized cto cell number to the square root of animal area as an approximate of animal length. Log2-fold change of normalized cto cell number was computed for each specimen by comparison to the average of control RNAi treatments, and these values for all fragment types (A-score heads and tails, B-score trunks), were binned and plotted in R using ggplot2. T-tests comparing area-normalized cto+ cell numbers between each RNAi condition and RNAi controls were adjusted for false discovery using the Benjamini-Hochberg method as implemented in R.
Phylogenetic analysis
Request a detailed protocolProtein sequences were aligned using MUSCLE with default settings (Chojnacki et al., 2017). Maximum likelihood analysis was run using PhyML with 100 bootstrap replicates, the WAG model of amino acid substitution, four substitution rate categories, and the proportion of invariable sites estimated from the dataset (Guindon and Gascuel, 2003; Guindon et al., 2010). We used proteins from Homo sapiens (hs), Mus musculus (mm), danio rerio (dr), Drosophila melanogaster (dm), and Caenorhabditis elegans (ce).
RNAi
Request a detailed protocolRNAi was performed by dsRNA feeding. For RNAi, dsRNA was synthesized from in vitro transcription reactions (NxGen, Lucigen). dsRNA corresponding to Caenorhabditis elegans unc-22, not present in the planarian genome, served as a negative control. Unless noted otherwise, animals were fed a mixture of liver paste and dsRNA six times in two weeks prior to amputation of heads and tails.
In Situ hybridization, Immunostaining and qPCR
Request a detailed protocolAnimal fixation, bleaching, and in situ hybridization were performed as previously described (Pearson et al., 2009; King and Newmark, 2013). Briefly, fixation, bleaching, and probe synthesis and hybridization were performed according to Pearson et al. Antibody blocking and tyramide development were performed according to King and Newmark. Digoxigenin- or fluorescein-labeled riboprobes were detected with anti-digoxigenin-HRP (1:2000, Roche/Sigma-Aldrich 11207733910, RRID: AB_514500), anti-fluorescein-HRP (1:2000, Roche/Sigma-Aldrich 11426346910, RRID: AB_840357). Hoechst 33342 (Invitrogen) was used at 1:1000 as a counterstain. Colorimetric (NBT/BCIP) assays were performed as described and detected with anti-digoxigenin-AP (1:4000, Roche/Sigma-Aldrich 11093274910).
For immunostainings, animals were fixed in 4% formaldehyde. Antibodies against mouse anti-tubulin alpha (1:1000 anti-Tubulin Alpha Neomarkers) or rat anti-BrdU (1:1000 Abcam 6326) and detected with goat anti-mouse HRP (1:150 Life Technologies, T20914) or goat anti-rat HRP (1:1000, Jackson ImmunoResearch 112-036-072) respectively. For histone staining, animals were fixed in Carnoy's solution as described (Umesono et al., 1997), using tyramide amplification to detect labeling with rabbit anti-phospho-ser10 Histone H3 (1:3000, Cell Signaling D2C8). Rabbit anti-PIWI1 polyclonal antibody (a kind gift of P. Reddien) was used at 1:1000 and detected with goad anti-rabbit HRP (1:150 Life Technologies, T20924).
For qPCR, total animal RNA was collected using Trizol with a tissue homogenizer, reverse transcribed with oligo-dT primers using Superscript II. qPCR was conducted to detect tec-1 mRNA using tec-1 primers (5’-GTTTTGATGCTAGAATGTTG-3’ and 5-TTTGACACACATACTCAAAG-3’), with normalization to a ubiquitously expressed gene gapdh detected with gapdh primers (5'-TGGTATTCAATTGACCGATACG-3' and 5'-GATCGATTACACGGCAACTG-3') using the delta-Ct method.
BrdU, TUNEL staining, and Irradiation
Request a detailed protocolFor BrdU experiments, the concentration of Montjuic salts were gradually increased to 5x one week before surgery. Animals were treated with 0.0625% N‐acetyl cysteine dissolved in 1x Montjuic salts for one minute, washed with 1xMontjuic salts, and incubated in 25 mg/ml BrdU (Sigma) dissolved in 1x salts containing 3% dimethyl sulfoxide for 4 hr either 1 hr before amputation (day 0 soak), or 2 or 4 days post-amputation (day two soak or day four soak respectively). Animals were then maintained in 5x Montjuic salts until fixation on the day indicated in the experiment (Cowles et al., 2012; Zhu and Pearson, 2018). The BrdU and in situ hybridizations were carried out as previously described above, with all HRP inactivations carried out using formaldehyde (4% in 1xPBSTx for at least 45 min) (Hill and Petersen, 2018). Briefly, animals were rehydrated and bleached in 6% hydrogen peroxide in PBSTx for 3–4 hr on a light box. Following FISH protocol described as above, acid hydrolysis was performed in 2N HCl for 45 min, samples were washed with 1xPBS (twice) then 1xPBSTx (four times), and blocked in PBSTB for 6 hr at room temperature. Primary antibody incubation was performed using rat anti-BrdU antibody (1:1000 in PBSTB, Abcam 6326) overnight at room temperature, followed by 6x washes in PBSTB, and overnight incubation in anti-rat HRP secondary antibody (1:1000, Jackson ImmunoResearch 112-036-072). Tyramide development was performed at room temperature for 1 hr (Invitrogen Alexa568-TSA Kit, tyramide at final concentration of 1:150).
Terminal uridine nick-end labeling (TUNEL) was performed as described by Pellettieri et al. (2010), with modifications. Animals were sacrificed in 5% N-acetyl-cysteine in 1 × PBS, fixed in 4% formaldehyde in 1 × PBSTx, and bleached overnight in 6% hydrogen peroxide in 1 × PBSTx. Samples were labeled with DIG-11-dUTP (Roche) by terminal deoxyuridine transferase (TdT) reaction (Thermo) at 37°C for 2 hr, then blocked and incubated overnight in anti-DIG-POD (Roche; 1:2000 in 10% horse serum in 1 × PBSTx) prior to tyramide development (Invitrogen).
To measure neuronal persistence after neoblast ablation, animals were fed and cut as described above. Regenerating trunk fragments were irradiated at 6 days post amputation. Irradiations were carried out in a RS2000 Biological Research Irradiator (Rad Source) at 160 kV over 13’30’ for a total, lethal dose of 60 Gy (Bardeen and Baetjer, 1904; Reddien et al., 2005). Irradiated fragments were fixed at 8, 10, and 12 days post-amputation.
Image analysis and cell counting
Request a detailed protocolNBT/BCIP-stained animals were imaged with a Leica M210F dissecting microscope and a Leica DFC295, with adjustments to brightness and contrast using Adobe Photoshop. Fluorescent-stained animals were imaged with a Leica TCS SPE confocal microscope or a Leica DM5500B compound microscope.
Unless otherwise noted, cell numbers were counted manually or using Image J’s Cell Counter analysis tool (Schindelin et al., 2012; Schneider et al., 2012). Cell counts were normalized to animal length or brain length using ImageJ or to the square root of the animal’s area calculated by Hoechst staining using CellProfiler (Lamprecht et al., 2007) as noted. opsin+ photoreceptor neurons were counted using Imaris x64 7.0.0 (Bitplane AG, Badenerstrasse 682, 8048 Zürich, Switzerland). Surface module was used to define either opsin+ or tyrosinase+ volume on each eye and then Spots module was used to count nuclei inside these volumes, as previously described (Vásquez-Doorman and Petersen, 2016). Data of cell counts from individual samples used for plotting in each figure panel is presented in Supplementary file 3.
For analysis of cto+ cell size and density, z-stacks of animals stained with a cto riboprobe were imaged at 20x on a Leica SPE with one micron slices, and cto+ cells were segmented in Fiji/ImageJ using the ‘3D object counter 2.0’ plugin after manual thresholding, and the X,Y,Z centroid positions of each cell and cell volume were obtained. Nearest-neighbor analysis was performed by computing the matrix of all pairwise distances between all cell centroids, then for each cell determining the distance between the nearest distinct cell.
Data availability
All data analyzed during this study are presented in the manuscript and supporting files.
References
-
The inhibitive action of the roentgen rays on regeneration in planariansJournal of Experimental Zoology 1:191–195.https://doi.org/10.1002/jez.1400010107
-
Tec family kinases in T lymphocyte development and functionAnnual Review of Immunology 23:549–600.https://doi.org/10.1146/annurev.immunol.22.012703.104743
-
Programmatic access to bioinformatics tools from EMBL-EBI update: 2017Nucleic Acids Research 45:W550–W553.https://doi.org/10.1093/nar/gkx273
-
Cell biology in neuroscience: death of developing neurons: new insights and implications for connectivityThe Journal of Cell Biology 203:385–393.https://doi.org/10.1083/jcb.201306136
-
Adult neurogenesis in DrosophilaCell Reports 3:1857–1865.https://doi.org/10.1016/j.celrep.2013.05.034
-
Neoblasts and the evolution of whole-body regenerationCurrent Opinion in Genetics & Development 40:131–137.https://doi.org/10.1016/j.gde.2016.07.009
-
Drosophila neuroblasts: a model for stem cell biologyDevelopment 139:4297–4310.https://doi.org/10.1242/dev.080515
-
PTEN deletion enhances the regenerative ability of adult corticospinal neuronsNature Neuroscience 13:1075–1081.https://doi.org/10.1038/nn.2603
-
Neuroprotection for traumatic brain injury: translational challenges and emerging therapeutic strategiesTrends in Pharmacological Sciences 31:596–604.https://doi.org/10.1016/j.tips.2010.09.005
-
Eye absence does not regulate planarian stem cells during eye regenerationDevelopmental Cell 40:381–391.https://doi.org/10.1016/j.devcel.2017.02.002
-
The novel roles of glial cells revisited: the contribution of radial Glia and astrocytes to neurogenesisCurrent Topics in Developmental Biology 69:67–99.https://doi.org/10.1016/S0070-2153(05)69004-7
-
Search for the evolutionary origin of a brain: planarian brain characterized by microarrayMolecular Biology and Evolution 20:784–791.https://doi.org/10.1093/molbev/msg086
-
Bromodeoxyuridine specifically labels the regenerative stem cells of planariansDevelopmental Biology 220:142–153.https://doi.org/10.1006/dbio.2000.9645
-
Reconstruction of dopaminergic neural network and locomotion function in planarian regeneratesDevelopmental Neurobiology 67:1059–1078.https://doi.org/10.1002/dneu.20377
-
Characterization of tyramine beta-hydroxylase in planarian dugesia Japonica: cloning and expressionNeurochemistry International 53:184–192.https://doi.org/10.1016/j.neuint.2008.09.006
-
Axon guidance and injury-lessons from wnts and wnt signalingCurrent Opinion in Neurobiology 27:232–240.https://doi.org/10.1016/j.conb.2014.05.005
-
Allometric scaling and proportion regulation in the freshwater planarian Schmidtea mediterraneaDevelopmental Dynamics 226:326–333.https://doi.org/10.1002/dvdy.10228
-
Formaldehyde-based whole-mount in situ hybridization method for planariansDevelopmental Dynamics 238:443–450.https://doi.org/10.1002/dvdy.21849
-
Cell death and tissue remodeling in planarian regenerationDevelopmental Biology 338:76–85.https://doi.org/10.1016/j.ydbio.2009.09.015
-
Maturation and death of adult-born olfactory bulb granule neurons: role of olfactionThe Journal of Neuroscience 22:6106–6113.https://doi.org/10.1523/JNEUROSCI.22-14-06106.2002
-
Neuronal survival in the brain: neuron type-specific mechanismsCell Death & Disease 8:e2643.https://doi.org/10.1038/cddis.2017.64
-
BTK: a two-faced effector in Cancer and tumour suppressionCell Death & Disease 9:1064.https://doi.org/10.1038/s41419-018-1122-8
-
New insights into pre-BCR and BCR signalling with relevance to B cell malignanciesNature Reviews Immunology 13:578–591.https://doi.org/10.1038/nri3487
-
Bcl-x is required for proper development of the mouse substantia nigraJournal of Neuroscience 25:6721–6728.https://doi.org/10.1523/JNEUROSCI.0760-05.2005
-
Fiji: an open-source platform for biological-image analysisNature Methods 9:676–682.https://doi.org/10.1038/nmeth.2019
-
NIH image to ImageJ: 25 years of image analysisNature Methods 9:671–675.https://doi.org/10.1038/nmeth.2089
-
Bax deficiency prevents the increased cell death of immature neurons in bcl-x-deficient miceThe Journal of Neuroscience 17:3112–3119.
-
Wnt/β-catenin signaling promotes regeneration after adult zebrafish spinal cord injuryBiochemical and Biophysical Research Communications 477:952–956.https://doi.org/10.1016/j.bbrc.2016.07.006
-
Programmed cell death of adult-generated hippocampal neurons is mediated by the proapoptotic gene baxJournal of Neuroscience 24:11205–11213.https://doi.org/10.1523/JNEUROSCI.1436-04.2004
-
Beyond calcium: new signaling pathways for tec family kinasesJournal of Cell Science 115:3039–3048.
-
Considering the evolution of regeneration in the central nervous systemNature Reviews Neuroscience 10:713–723.https://doi.org/10.1038/nrn2707
-
The cellular basis for animal regenerationDevelopmental Cell 21:172–185.https://doi.org/10.1016/j.devcel.2011.06.016
-
A planarian orthopedia homolog is specifically expressed in the branch region of both the mature and regenerating brainDevelopment, Growth and Differentiation 39:723–727.https://doi.org/10.1046/j.1440-169X.1997.t01-5-00008.x
-
Long-term survival and cell death of newly generated neurons in the adult rat olfactory bulbEuropean Journal of Neuroscience 16:1681–1689.https://doi.org/10.1046/j.1460-9568.2002.02238.x
-
Programmed cell death in neurodevelopmentDevelopmental Cell 32:478–490.https://doi.org/10.1016/j.devcel.2015.01.019
-
Repair effect of Wnt3a protein on the contused adult rat spinal cordNeurological Research 30:480–486.https://doi.org/10.1179/174313208X284133
Article and author information
Author details
Funding
National Institutes of Health (1R01GM129339)
- Christian P Petersen
Natural Sciences and Engineering Research Council of Canada (PGSD3-471547-2015)
- Nicolle A Bonar
National Institutes of Health (R01GM130835)
- Christian P Petersen
The funders had no role in study design, data collection and interpretation, or the decision to submit the work for publication.
Acknowledgements
We thank members of the Petersen lab for critical comments and P Reddien for the kind gift of the PIWI-1 antibody.
Copyright
© 2020, Karge et al.
This article is distributed under the terms of the Creative Commons Attribution License, which permits unrestricted use and redistribution provided that the original author and source are credited.
Metrics
-
- 1,430
- views
-
- 170
- downloads
-
- 11
- citations
Views, downloads and citations are aggregated across all versions of this paper published by eLife.
Citations by DOI
-
- 11
- citations for umbrella DOI https://doi.org/10.7554/eLife.47293