β3-Adrenoceptor redistribution impairs NO/cGMP/PDE2 signalling in failing cardiomyocytes
Abstract
Cardiomyocyte β3-adrenoceptors (β3-ARs) coupled to soluble guanylyl cyclase (sGC)-dependent production of the second messenger 3’,5’-cyclic guanosine monophosphate (cGMP) have been shown to protect from heart failure. However, the exact localization of these receptors to fine membrane structures and subcellular compartmentation of β3-AR/cGMP signals underpinning this protection in health and disease remain elusive. Here, we used a Förster Resonance Energy Transfer (FRET)-based cGMP biosensor combined with scanning ion conductance microscopy (SICM) to show that functional β3-ARs are mostly confined to the T-tubules of healthy rat cardiomyocytes. Heart failure, induced via myocardial infarction, causes a decrease of the cGMP levels generated by these receptors and a change of subcellular cGMP compartmentation. Furthermore, attenuated cGMP signals led to impaired phosphodiesterase two dependent negative cGMP-to-cAMP cross-talk. In conclusion, topographic and functional reorganization of the β3-AR/cGMP signalosome happens in heart failure and should be considered when designing new therapies acting via this receptor.
Introduction
Over the last two decades, functional β3-adrenergic receptors (β3-ARs) have been found and studied in cardiomyocytes isolated from various species including humans and rodents (Gauthier et al., 1998; Mongillo et al., 2006; Hammond and Balligand, 2012). Depending on the cell type (cardiomyocytes vs adipocytes or atrial vs ventricular myocytes), β3-ARs have been reported to couple to both stimulatory (Gs) and inhibitory (Gi) proteins and to regulate cardiac contractility. In human and rodent ventricular myocardium, catecholamine binding to β3-ARs elicits negative inotropic and positive lusitropic effects by signalling via Gi and the second messenger 3’,5’-cyclic guanosine monophosphate (cGMP) (Gauthier et al., 1998; Mongillo et al., 2006). Unlike β1- and β2-AR, the β3-AR is resistant to agonist-induced desensitization, (Liggett et al., 1993; Nantel et al., 1993) and its expression is increased in heart failure as well as in sepsis and diabetic cardiomyopathy (Amour et al., 2007; Moniotte et al., 2007; Moniotte et al., 2001). It was hypothesised that β3-AR/cGMP pools can attenuate excessive cardiotoxic β1-AR/cAMP signalling, as well as pathological cardiac hypertrophy and remodelling which takes place in cardiomyocytes during the progression towards heart failure (Mongillo et al., 2006; Hammond and Balligand, 2012; Takimoto et al., 2005). Endothelial nitric oxide synthase (eNOS), has been detected in close proximity to β3-ARs in cardiomyocyte caveolae structures. The caveolae are believed to provide discrete signalling domains, necessary for the autonomic regulation of the heart (Feron et al., 1998). It has been shown indirectly that β3-AR/cGMP is most likely degraded by the phosphodiesterases 2 and 5 (Mongillo et al., 2006; Takimoto et al., 2005). Recently, overexpression of β3-AR in transgenic mice has been shown to protect the heart from catecholamine-induced hypertrophy and remodelling via an eNOS/soluble guanylyl cyclase (sGC)/cGMP-dependent signalling pathway. The same study showed localization of β3-ARs together with eNOS in caveolae-enriched membrane fractions, which had been separated via ultracentrifugation (Belge et al., 2014). Another mouse study identified the sGC subunit α1 as the facilitator of the NO dependent but Ca2+ independent effects of β3-AR using sGC α1 KO mice (Cawley et al., 2011). Despite its name the ‘soluble’ sGC has been shown to act in close association with β3-ARs and membrane located signalosomes (Mongillo et al., 2006; Feron et al., 1998). However, the exact localization of functional β3-ARs in adult cardiomyocytes and the spatio-temporal regulation of their cGMP signals as well as their potential interaction with cAMP signalling pathways have not been studied before.
In this study, we employ a highly sensitive Förster Resonance Energy Transfer (FRET)-based biosensor, red cGES-DE5, in combination with scanning ion conductance microscopy (SICM). We demonstrate that in healthy rat cardiomyocytes, functional β3-ARs are localized exclusively within the transverse (T)-tubules and stimulate a cGMP pool which is predominantly regulated by phosphodiesterases (PDEs) 2 and 5. Furthermore, by using the cAMP specific FRET-based biosensor Epac1-camps we show that β3-AR stimulation can decrease overall adenylate cyclase dependent cAMP levels in healthy cardiomyocytes by a PDE2-mediated cGMP-to-cAMP cross-talk. This cross-talk appears to be disrupted in heart failure, where β3-AR stimulation no longer has a significant effect on overall cAMP levels. In failing cells, β3-AR/cGMP signals decrease in the T-tubules. Heart failure leads to altered co-localization of sGC and caveolin-3, as shown via immunocytochemical staining. Together, these alterations result in the impairment of the β3-AR-dependent cGMP signalling pathway and of a PDE2-mediated β3-AR induced decrease of local cAMP.
Results
Echocardiography and biometric data show heart failure phenotype
To study β3-AR-dependent cGMP dynamics, we used ventricular cardiomyocytes isolated from healthy and failing rat hearts transduced with an adenovirus to express a highly sensitive cytosolic FRET biosensor red cGES-DE5. As a model of heart failure, we used rats which underwent left coronary artery ligation for 16 weeks (Lyon et al., 2009). Echocardiographic and biometric data from these animals are summarized in Figure 1. Data analysis showed typical clinical signs of heart failure, including a loss of pump function, left ventricular dilation and left ventricular wall thickening.
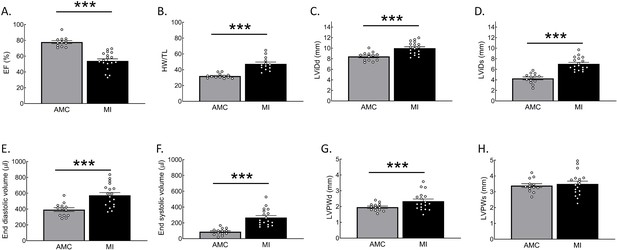
Histograms of echocardiography and biometric data in rat age matched control (AMC) hearts and hearts with myocardial infarction (MI).
(A) Ejection Fraction, (B) Heart weight (HW) corrected to tibia length (TL), (C) left ventricular diastolic internal dimension (LViDd), (D) left ventricular systolic internal dimension (LViDs), (E) end-diastolic volume, (F) end-systolic volume, (G) end-diastolic left ventricular posterior wall thickness (LVPWd), (H) end-systolic left ventricular posterior wall thickness (LVPWs). Statistical significance was analysed via two-sided T-test. ***p<0.001.
-
Figure 1—source data 1
Echocardiography and biometric data for respective treatment groups.
- https://cdn.elifesciences.org/articles/52221/elife-52221-fig1-data1-v2.xlsx
Isoproterenol (ISO) induces a β3-AR-dependent cGMP increase in adult rat cardiomyocytes
β-adrenergic stimulation (ISO, 100 nmol/L) of healthy control rat ventricular cardiomyocytes expressing the cGMP biosensor red cGES-DE5, led to the production of substantial amounts of cGMP (Figure 2A) in about 2/3 of all tested cells. In failing cardiomyocytes isolated from rats 16 weeks post-myocardial infarction, administration of the same saturating concentrations of ISO resulted in a significant two-fold reduction in the amount of detectable cGMP (Figure 2B, p=0.0465). Blocking β1- and β2-ARs (with 100 nmol/L CGP20712A and 50 nmol/L ICI118551, respectively) in control cells did not abolish this cGMP production (Figure 2C). The signal was however strongly and significantly inhibited in control cells by the application of the β3-AR antagonist SR59230A (Figure 2D, p=0.0316) or by the nitric oxide synthase (NOS) blocker, nitro-L-arginine methyl ester (L-NAME, Figure 2E, p=0.0217).
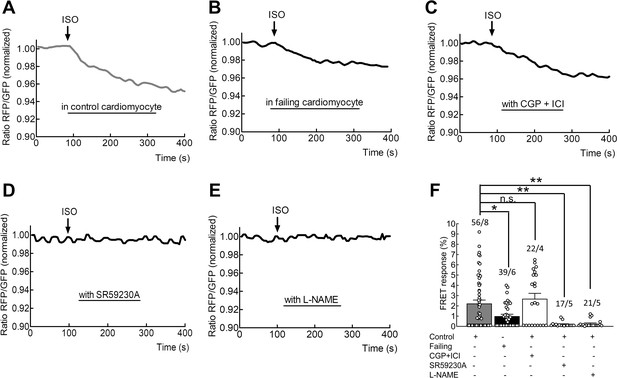
Measurements of β3-AR-dependent cGMP responses in adult cardiomyocytes.
Representative FRET tracings of a control (A) or a failing cardiomyocyte (B) treated with isoproterenol (100 nmol/L). FRET responses of control cardiomyocytes pre-treated for 5 min with either with the β1-AR and β2-AR inhibitors CGP20712A (100 nmol/L) and ICI118,551 (50 nmol/L) (C), β3-AR inhibitor SR59230A (100 nmol/L) (D) or for 10 min with the nitric oxide synthase blocker L-NAME (300 µmol/L) (E), before the application of isoproterenol (100 nmol/L). (F) Quantification of whole cell cGMP-FRET responses from protocols described in A-E). Error bars represent standard error of the mean. Numbers of cells/hearts are shown above the bars. Statistical significance was calculated via Mann Whitney U-test for independent treatments versus control followed by Bonferroni correction: *p<0.05; **p<0.01.
-
Figure 2—source data 1
FRET microscopy data - 'whole-cell' analysis.
- https://cdn.elifesciences.org/articles/52221/elife-52221-fig2-data1-v2.xlsx
β3-AR/cGMP is preferentially controlled by PDE2 and PDE5
Next, we stimulated cells with ISO and then applied selective inhibitors of the various cGMP-degrading PDEs to investigate the regulation of β3-AR/cGMP dynamics. Following the application of selective PDE blockers we applied the non-selective PDE inhibitor IBMX. We found that β3-AR/cGMP levels are under the control of multiple PDEs. PDE1 inhibition has a minimal effect on β3-AR/cGMP production, whereas PDE2 and PDE5 represent the most prominent β3-AR/cGMP degrading families (Figure 3B,D). Both PDE2 and PDE5 contribute to more than a half of the overall PDE inhibitory response as determined by IBMX treatment (Figure 3B,D and E). Furthermore, we observed that in failing cells, the PDE2, PDE3 and PDE5 inhibitor effects, while not statistically significant, showed a tendency towards increasing (Figure 3B,D and E).
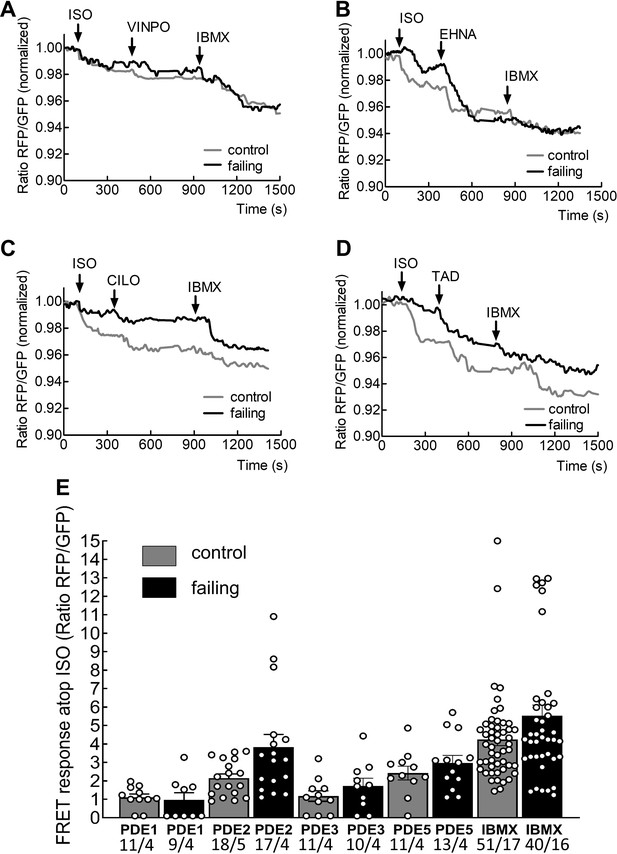
Investigation of phosphodiesterase regulation of β3-AR/cGMP in adult cardiomyocytes.
Representative FRET response curves of control (grey line) and failing (black line) cardiomyocytes following whole cell treatment with isoproterenol (100 nmol/L) followed by the PDE1 blocker vinpocetine (VINPO, 10 µmol/L) (A), the PDE2 inhibitor EHNA (10 µmol/L) (B), the PDE3 inhibitor cilostamide (CILO, 10 µmol/L) (C) and the PDE5 inhibitor tadalafil (TAD, 100 nmol /L) (D) followed by the non-specific PDE blocker IBMX (100 µmol/L). The scatter plot/histograms present whole cell cGMP-FRET responses evoked by PDE inhibition further to the isoproterenol responses in % from (A–D) (E) Error bars represent standard error of the mean. Numbers of cells/hearts are shown below the bars. Statistical significance was calculated via mixed ANOVA followed by χ2-test: No statistically significant differences between control and failing conditions for any PDE could be detected, only tendencies to increased responses for PDE2, PDE3 and PDE5 inhibitors.
-
Figure 3—source data 1
FRET microscopy data - 'whole cell' analysis PDEs.
- https://cdn.elifesciences.org/articles/52221/elife-52221-fig3-data1-v2.xlsx
Functional β3-ARs are localized in the T-tubules of healthy cells and migrate to the non-tubular sarcolemma in heart failure
Using SICM/FRET we were able to localize functional β3-ARs by measuring cGMP-FRET signals following local ligand application from the SICM nanopipette. This approach stimulates cardiomyocytes specifically within T-tubules or on the non-tubular sarcolemma. In healthy cardiomyocytes, we observed that functional β3-ARs reside mainly in the T-tubules with very few responses being detectable outside of T-tubules (Figure 4A–E), whereas in failing cells, the β3-ARs responses after localized stimulation can be detected in both tubulated and non-tubulated areas across the sarcolemma (Figure 4F–J).
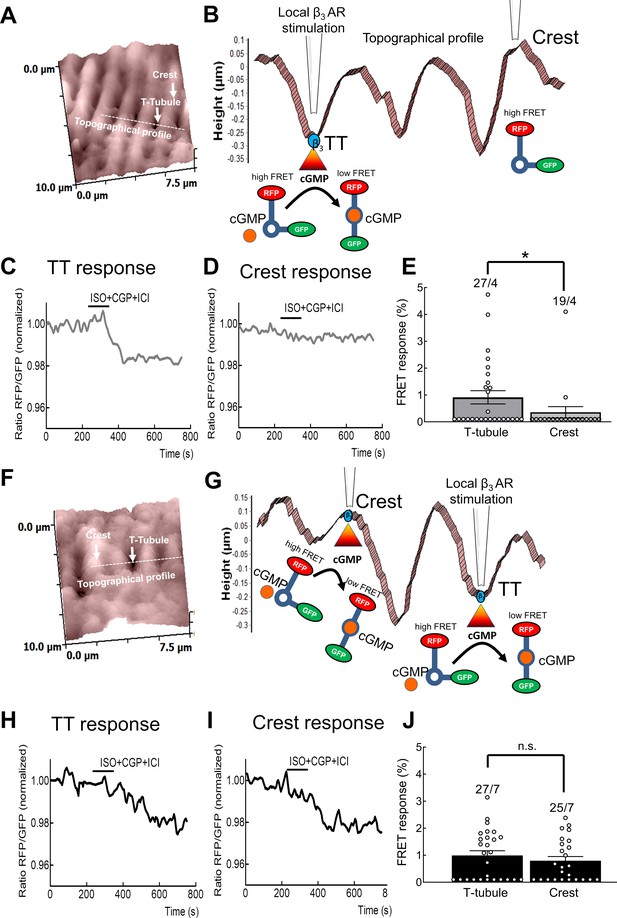
Identification of β3-AR/cGMP signal localization using scanning ion conductance microscopy (SICM) combined with Förster Resonance Energy Transfer (FRET).
Representative SICM surface scan of a 10 × 10 µm area of a healthy (A) and a failing cardiomyocyte (F). White arrows indicate T-tubule or crest structures and a dotted white line indicates the areas selected for the topographical profiles presented in (B) and (G). Representative topographical profiles of healthy (B) and failing cardiomyocytes (G). Images present schematics of local β3-AR stimulation with Isoproterenol (50 µmol/L) either inside a T-tubule opening or on the cell crest via the SICM nanopipette. Representative FRET response curves during perfusion with the β1-AR blocker CGP20712A (100 nmol/L) and the β2-AR blocker ICI118551 (50 nmol/L) and local stimulation inside the T-tubule (C) and the crest of a control cardiomyocyte (D) or the T-tubule (H) and crest (I) of a failing cardiomyocyte. Scatter plots presenting the localised cGMP-FRET responses of control (E) and failing cardiomyocytes (J). Error bars represent standard error of the mean. Numbers of cells/hearts are shown above the bars. *p<0.05, n.s. – not significant by Mann-Whitney U test.
-
Figure 4—source data 1
FRET microscopy data - SICM/FRET.
- https://cdn.elifesciences.org/articles/52221/elife-52221-fig4-data1-v2.xlsx
The increased activity of β3-ARs in non-tubulated surface areas in failing cells might be linked to a disrupted association of β3-AR with caveolar signalosomes. We investigated this hypothesis by using the cell-permeable peptide disruptor of caveolar signalling TAT-C3SD. The addition of this peptide leads to the dissociation of caveolar signalosomes by inhibiting signalling which is dependent upon the binding to the caveolin-3 specific scaffolding domain (C3SD) (Macdougall et al., 2012). In control cells, β3-AR-cGMP responses in the T-tubules are higher than in the non-tubulated cell surface areas, as is seen in Figure 4E. However, the T-tubular localization of the receptor can be abolished by treating cells with the TAT-C3SD peptide (Figure 4—figure supplement 1), so that the response level in the crest areas equals the response level of the T-tubules.
Heart failure disrupts β3-AR associated sGC localization in caveolin-rich membrane domains
To precisely investigate the localization of the components of the β3-AR signalosome in control cells and failing cardiomyocytes, we performed immunocytochemical staining of sGC subunits together with caveolin-3 or α-actinin. We detected partial co-localization of sGCα1 with caveolin-3 (Figure 5A,C,E) which was significantly decreased by about 20% in failing cardiomyocytes (Figure 5E, p=0.0014). Concomitantly, sGCα1 co-localization with the microfilament protein α-actinin (Figure 5B,D,F) had a tendency to increase in failing cardiomyocytes (Figure 5F, p=0.0244), whereas sGCβ1 subunit co-localization with caveolin-3 also decreased by about 16% (Figure 5—figure supplement 1, p=0.0044), indicating a redistribution of sGC away from caveolin-rich microdomains.
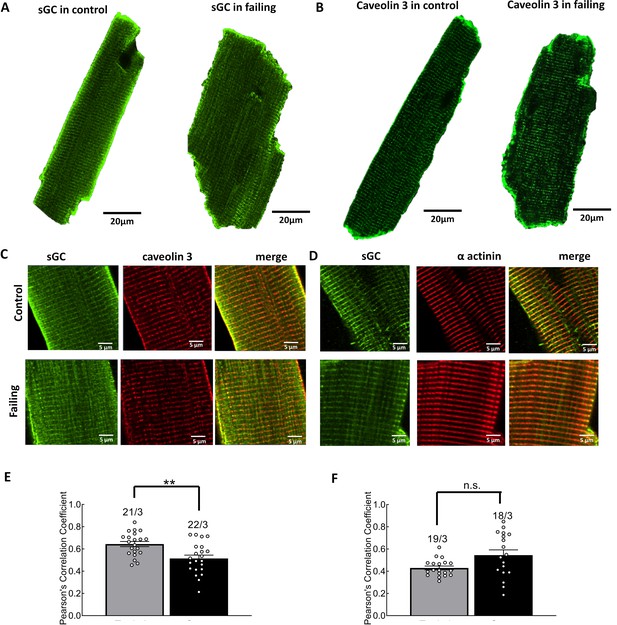
Investigation of sGC and caveolin-3 localization in control and failing cardiomyocytes.
Representative, confocal images of sGCα1 (A) and caveolin-3(B) in control and failing cardiomyocytes. Magnified representations of double staining of sGCα1(C) with caveolin-3 and of sGCα1 (D) with α-actinin. Quantification of sGC (E) and caveolin-3 and of sGC (F) and α-actinin co-localization in control and failing cells. Error bars represent standard error of the mean. Numbers of cells/hearts are shown above the bars. Statistical significance was analyzed via mixed ANOVA followed by χ2-test; **p<0.01, n.s. – not significant.
-
Figure 5—source data 1
Immunostaining data sGC and Cav3 colocalization control.
- https://cdn.elifesciences.org/articles/52221/elife-52221-fig5-data1-v2.xlsx
Heart failure impairs PDE2 mediated cGMP-to-cAMP cross-talk after β3-AR stimulation
To investigate whether the β3-AR signals we detected in the experiments above were able to influence cAMP signalling in cardiomyocytes, we expressed the cAMP biosensor Epac1-camps in healthy and failing cardiomyocytes. To analyse the cGMP-to-cAMP cross-talk, we treated the cell with the adenylyl cyclase activator forskolin with and without β3-AR agonist CL316,243 (Figure 6A,B). In healthy cells, stimulation of β3-AR led to a significant reduction of approximately 10.3% of the forskolin stimulated cAMP production (Figure 6C, p=0.040). The PDE2 inhibitor BAY60-7550 used in this setting was able to abolish the observed β3-AR agonist effects on cAMP levels (Figure 6A,C, p=0.0458). We used forskolin to directly activate adenylate cyclases downstream of β-ARs to allow us a direct comparison between control and MI cells, which might have been complicated otherwise due to β1-AR receptor desensitisation. Nonetheless, our result suggests that β3-ARs acts via increased PDE2 activation to attenuate cAMP responses. In failing myocytes, the effect of CL316,243 on the cAMP response was no longer significant (Figure 6B,C), suggesting that the PDE2 mediated cGMP-to-cAMP crosstalk is disrupted by disease.
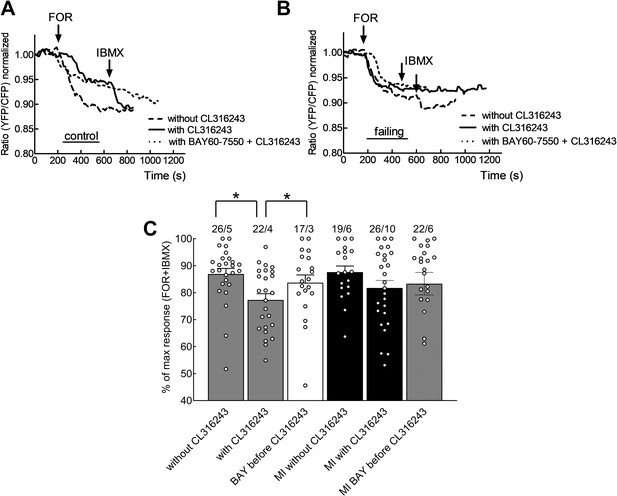
β3-AR signalling can affect cAMP levels via PDE2.
Representative FRET responses for control cardiomyocytes (A) or failing cardiomyocytes from myocardial infarction (MI) hearts (B) stimulated using forskolin (FOR, 10 µmol/L) applied with or without the β3-AR agonist CL316243 (1 µmol/L) and with or without pre-treatment with the PDE2 inhibitor BAY60-7550 (100 nmol/L). (C) Scatter plot/histogram presenting whole cell cAMP-FRET responses depicted as the percentage of the maximal possible cAMP FRET response (=Forskolin followed by IBMX). The measured Forskolin or IBMX responses were the respective maximal responses, equalling the lowest FRET ratio value, achieved after each stimulus. The effect of the β3-AR agonist on forskolin induced cAMP levels is no longer discernible after inhibition of PDE2 in control cardiomyocytes. This effect is no longer significant in failing cardiomyocytes. Error bars represent standard error mean. Statistical significance was calculated via mixed ANOVA followed by χ2-test* p<0.05.
-
Figure 6—source data 1
FRET microscopy data cAMP/cGMP crosstalk experiment.
- https://cdn.elifesciences.org/articles/52221/elife-52221-fig6-data1-v2.xlsx
Discussion
The pharmacological modulation of β3-AR for the treatment of cardiac hypertrophy and heart failure has recently emerged as a promising therapeutic route in translational research (Belge et al., 2014). Previous studies have suggested that the β3-AR are localized in caveolae, in close proximity to its signalling partners eNOS and sGC (Mongillo et al., 2006; Belge et al., 2014). However, the exact sub-membrane localization of β3-AR and the compartmentation of its signalling to cGMP were not well understood. Alterations in β3-AR signalling in disease states have been difficult to study due to the lack of appropriate imaging techniques and specific antibodies which work in situations of relatively low endogenous expression. In this work, we studied the exact submembrane localization of β3-AR and alterations to β3-AR/cGMP signalling in failing cells using new cutting-edge biophysical approaches such as SICM and FRET. We were able to directly visualize compartmentalized β3-AR/cGMP production in the cellular context of adult rat cardiomyocytes and its disruption in heart failure. Using FRET imaging in the presence of either β3-AR agonists or antagonists, we show that these cells can produce cGMP following direct agonist stimulation of β3-ARs (see Figure 2). In some, but not all cells, residual cGMP production was still detectable despite β3-AR and NOS inhibition. This is an observation which has previouslybeen reported in neonatal rat cardiomyocytes (Mongillo et al., 2006). β3-AR dependent cGMP pools are mainly formed in the T-tubules (see Figure 4C) due to the localization of β3-ARs in close proximity to the caveolar signalosome, which among other molecules is comprised of eNOS and sGC. To further substantiate the association of functional β3-AR to caveolae, we dissociated the signalosome of these lipid structures in healthy cardiomyocytes using a previously published peptide, which targets the caveolin-3 scaffolding domain (Macdougall et al., 2012). As a result, β3-AR-cGMP pools were induced outside of T-tubular domains (see Figure 4—figure supplement 1) which corroborates the importance of caveolar signalosomes for proper β3-AR regulation.
Using various family selective PDE inhibitors, we uncovered that β3-AR/cGMP signals are predominantly regulated by local pools of PDE2 and PDE5 (see Figure 3B,D and E). These findings are similar to what has been described for atrial natriuretic peptide-stimulated pools of submembrane cGMP in rat cardiomyocytes (Castro et al., 2006) and eNOS/sGC-dependent pools of cGMP in mouse cells (Takimoto et al., 2005). Further regulation via the cGMP specific and IBMX insensitive PDE9 cannot be completely excluded, although a recent study in mice suggests that PDE9 degrades cGMP pools generated downstream of natriuretic peptide receptors acting independently of NO (Lee et al., 2015). Interestingly, the overall β3-AR/cGMP response was significantly reduced in failing cells (Figure 2B,F) despite increased β3-AR expression reported in cardiac disease (Hammond and Balligand, 2012; Moniotte et al., 2001). This reduction in the signal could be in part be due to higher PDE activity on cGMP (Figure 3B,E) and an altered subcellular arrangement of sGC (Figure 5). sGC was found to redistribute away from caveolin-3 in heart failure, as demonstrated in this work by immunocytochemical double staining. We have observed a trend to an increased overlap between our sGCα and α-actinin staining in our confocal imaging, which could potentially represent an increased redistribution of sGCα to the areas of the Z-disc not directly associated with the T-tubules or caveolar signalosomes. Though the immunocytochemical method is limited in its spatial resolution and can therefore not resolve the caveolae structures themselves, it allows us to detect an alteration in sGC localization in heart failure, which could potentially be indicative of dysregulated caveolar signalosomes as reported previously in a pressure overload induced heart failure model using mice (Tsai et al., 2012). Decreased expression of eNOS in the caveolae, alongside increased expression of neuronal NOS (nNOS) at the sarcoplasmic reticulum has been reported in studies of tissue from humans with heart failure (Damy et al., 2004; Drexler et al., 1998). As eNOS function in cardiomyocytes has been shown to attenuate beta-adrenergic contractile responses (Farah et al., 2018), potential changes in local NOS activities for example due to decreased eNOS-caveolin-3 association (Feron et al., 1998) could further contribute to altered β3-AR/cGMP signal disruption. The slightly increased contributions of PDE2,3 and 5 to cGMP regulation which we observed in heart failure, are probably responsible for the overall reduction in cGMP levels, as can be seen when comparing total ISO plus IBMX responses in control and failing cells (Figures 2F and 3E). Increased expression and activity of the dual-specificity PDE2 has been shown in human heart failure (Mehel et al., 2013), as well as in a rodent model of aortic banding, where an increase in both cGMP and cAMP hydrolysis took place (Yanaka et al., 2003). At the same time as being degraded by PDE2, cGMP can also increase the effect of PDE2 on cAMP by binding to the PDE’s GAF-B domain and leading to a conformational change, thereby triggering the so-called cGMP-to-cAMP crosstalk. This crosstalk between the second messengers of which one (cAMP) has increasingly been associated with detrimental signalling pathways in the context of heart failure, which the other (cGMP) could potentially attenuate (Mongillo et al., 2006; Moniotte et al., 2001; Yanaka et al., 2003). Interestingly, we have also detected a decreased β3-AR induced attenuation of the forskolin induced cAMP response, due to PDE2 activity, which it was possible to abolished by blocking the PDE2 using a specific inhibitor (see Figures 6 and 7). This observed impairment of the cGMP/cAMP crosstalk further supports the hypothesis of dramatic spatial rearrangements in the β3-AR/sGC/PDE2 signalosome which impair cGMP dynamics and could potentially lead to depressed β3-AR effects on cardiac contractility demonstrated in similar animal models (Mongillo et al., 2006) and in human heart tissue samples (Moniotte et al., 2001). The observed response of β3-AR stimulation of about 10 percent on overall cAMP levels, as measured via the cytosolic FRET sensor Epac1-camps, could be of physiological relevance when brought into the context of lowering pathological cAMP signalling levels on a whole or in confined signalling compartments. We believe that altered compartmentation of subcellular cGMP may play a role in disease-associated changes in β-AR signalling. However, heart failure does not completely ablate β3-AR/cGMP responses, leaving room for a residual cardioprotective action of β3-ARs in the failing heart. This therapeutic potential is currently being addressed pharmacologically using the β3-AR agonist mirabegron in clinical studies to treat heart failure with preserved and reduced ejection fraction (Pouleur et al., 2018).
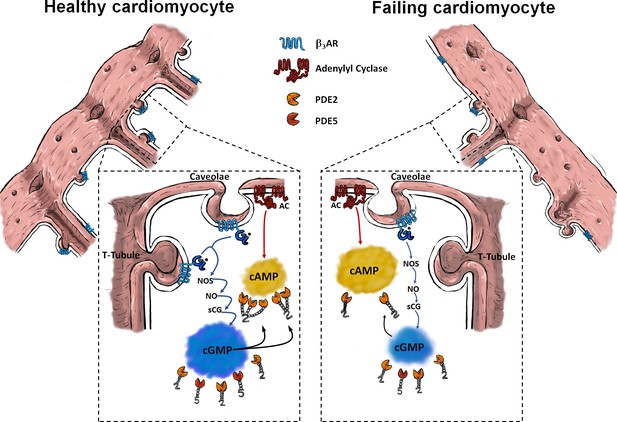
Schematic of β3-AR/cGMP signalling in healthy (left side) and failing (right side) cardiomyocytes.
In healthy cardiomyocytes, functional β3-ARs are associated with caveolar signalosomes and localized mostly in T-tubules. Via Gi/NOS/NO/sGC/cGMP signalling they can suppress strong cAMP responses by stimulating increased PDE2 dependent cAMP degradation through cGMP binding to GAF-B domain of PDE2. In heart failure, increased presence of β3-AR activity at non-tubular plasma membrane (Crest) and away from caveolin-3 associated membrane domains might disrupt receptor-associated cGMP signalosomes and lead to disrupted cGMP/cAMP-crosstalk.
In summary, our study reveals mechanisms of submembrane localization of cardiomyocyte β3-AR, which regulates the compartmentation of receptor coupling to cGMP production and disease-driven alterations in β3-AR/cGMP signalling. These data add insights to the growing body of data regarding the therapeutic implications for the potential treatment of heart failure by β3-AR agonists.
Materials and methods
Experimental reagents
Request a detailed protocolM199 medium (Invitrogen UK, 11150), taurine (Biochemica, A1141), creatine monohydrate (Sigma Aldrich, C3630), penicillin/streptomycin (Merck, A2212), carnitine hydrochloride (Sigma Aldrich, C9500) BSA (Sigma Aldrich, A6003), laminin (Sigma Aldrich L2020), isoproterenol hydrochloride (Sigma Aldrich, I6504), ICI118551 (Tocris UK, 0821), CGP 20712A (Tocris UK, 1024), SR 59230A hydrochloride (Tocris UK, 1511), L-NAME hydrochloride (Tocris UK, 0665), Vinpocetine (Sigma Aldrich, V6383), EHNA hydrochloride (Sigma Aldrich, E114), Cilostamide (Tocris UK, 0915), Tadalafil (Santa Cruz USA, sc-208412), IBMX (Santa Cruz sc-201188), self-made rabbit sGCα and β subunit antibodies (specificity tested in KO animals; Friebe et al., 2018), mouse α-actinin (Sigma Aldrich, A7732), mouse Caveolin-3 (BD Transduction Laboratories, 610421, specificity tested in KO animals; Woodman et al., 2002), secondary Alexa Fluor antibodies 488 nm, 514 nm, 568 nm and 633 nm (Life Technologies), BSA (Fisher Scientific UK, BPE9704), fluorescence mounting medium (Vectashield Germany, H-1000), MaTek glass-bottom dishes (MaTek USA, P35G-1.5–10 C), TAT-scram and TAT-Cav3 peptides (a gift from Dr. Sarah Calaghan from Leeds, England).
Myocardial infarction (MI) model
Request a detailed protocolAll animal experiments were performed in the United Kingdom (UK) according to the standards for the care and use of animal subjects determined by the UK Home Office (ASPA1986 Amendments Regulations 2012) incorporating the EU directive 2010/63/EU. The Animal Welfare and Ethical Review Body Committee of Imperial College London approved all protocols.
The parts of the investigation (on isolated cardiomyocytes from healthy rats) which were performed in Germany, conformed to the guide for the care and use of laboratory animals published by the National Institutes of Health (Bethesda, Maryland; Publication No. 85–23, revised 2011, published by National Research Council, Washington, DC). The experimental procedures were in accordance with the German Law for the Protection of Animals and with the guidelines of the European Community.
The following procedure was exclusively performed at Imperial College London in the UK: Left descending coronary artery ligation was performed as described (Nikolaev et al., 2010). Rats were monitored by echocardiography in M-mode under anaesthesia (2% isoflurane). Animals with induced MI were sacrificed 16 weeks after MI for ventricular cardiomyocyte isolation via enzyme digestion of the Langendorff perfused heart as described (Lyon et al., 2009). Age matched animals served as control. Echocardiographic and biometric data are summarized in Figure 1. All animals were male.
Peptide dissociation of caveolae signalosome
Request a detailed protocolFor disruption of the caveolar signalosome, cardiomyocytes were treated with a cell-membrane penetrating TAT peptide targeting the Caveolin-3 scaffolding domain (C3SD) (sequence: YGRKKRRQRRRGGGGDGVWRVSYTTFTVSKYWCYR) or with a scrambled control peptide without any cellular targets in cardiomyocytes (sequence: YGRKKRRQRRRGGGGYWTVYTKVDFCGSRYVRTSW) as described previously (Macdougall et al., 2012). Cells were incubated with peptides by directly putting the peptides into the cell medium for 30 min at 37°C.
Drug concentrations
Request a detailed protocolThe concentrations for pharmacological agonists and antagonists for the β-AR subtypes and the PDE subtypes investigated were taken from previously published work, which established the drug affinity through competitive radioligand binding assays, FRET sensor dose-response levels and PDE activity assays (Nikolaev et al., 2010; Nikolaev et al., 2006; Hoffmann et al., 2004; Johnson et al., 2012).
Whole cell and SICM/FRET cGMP measurements
Request a detailed protocolAfter isolation, adult rat ventricular myocytes were plated onto laminin-coated cover glasses or MatTek dishes and cultured in M199 media supplemented with creatine 5 mM, taurine 5 mM, carnitine 5 mM, bovine serum albumin 1%, ascorbate 1 mM and penicillin/streptomycin 1%, before being subjected to FRET and SICM/FRET measurements at room temperature as described (Nikolaev et al., 2010) 44–52 hr after transduction with adenovirus expressing the cGMP-FRET biosensor red cGES-DE5 (Götz et al., 2014) at a multiplicity of infection equal 300. The respective buffer, at pH = 7.4, in which the cells were imaged contained 144 mmol/L NaCl, 5.4 mmol/L KCl, 1 mmol/L MgCl2, 1 mmol/L CaCl2 and 10 mmol/L HEPES. Whole cell β3-AR/cGMP levels were measured by treating control and failing cardiomyocytes with 100 nmol/L isoproterenol. To determine the source of isoproterenol induced cGMP whole cell FRET measurements were performed by pre-blocking control cardiomyocytes either with 100 nmol/L of the β3-AR inhibitor SR 59230A for 5 min or with 100 nmol/L of the β1-AR blocker CGP 20712A and 50 nmol/L of the β2-AR blocker ICI 118,551 for 5 min, or with 300 µM Nω-Nitro-L-arginine methyl ester hydrochloride for 10 min, before applying 100 nmol/L of isoproterenol. For whole cell FRET measurements of phosphodiesterase dependent regulation of β3-AR/cGMP signals, cells were treated with 100 nmol/L isoproterenol followed by either 10 µmol/L of the specific phosphodiesterase blocker vinpocetine for PDE1, 10 µmol/L erythro-9-amino-β-hexylα-methyl-9H-purine-9-ethanol (EHNA) for PDE2, 10 µmol/L cilostamide for PDE3 or 100 nmol/L Tadalafil for PDE5, and 100 µmol/L of the unspecific PDE inhibitor 3-Isobutyl-1-methylxanthine (IBMX). To study β3-AR/cGMP localization on cardiomyocyte surface structures, cells were continuously superfused with FRET buffer containing 100 nmol/L of the β1-AR blocker CGP 20712A and 50 nmol/L of the β2-AR blocker ICI 118,551. SICM was used to scan and visualize cardiomyocyte T-tubule openings and crest surface structures. Next, the scanning nanopipette was lowered onto either T-tubule openings or crest structures and receptor ligand was locally applied from the nanopipette filled with 50 µmol/L Isoproterenol (ISO) and 50 µmol/L CGP 20712A and 25 µmol/L ICI 118,551 by switching SICM pipette holding potential from −200 to +500 mV as described (Schobesberger et al., 2016).
FRET-based cAMP measurements
Request a detailed protocolFreshly isolated cardiomyocytes were transduced with Epac1-camps (Nikolaev et al., 2004) adenovirus virus for 44–52 hr and exposed to whole cell FRET measurements for β3-AR effects on cAMP levels. Cells were kept in FRET buffer containing 50 nmol/L β2AR inhibitor ICI 118,551 before treating them with 100 nmol/L of the β3-AR agonist CL 316,243 plus 50 nmol/L ICI 118,551 or just adding FRET buffer with 50 nmol/L ICI 118,551 followed by addition of 10 µmol/L Forskolin and consequently 100 µmol/L IBMX into the solution. Additional cells were treated using the same protocol but with or without 100 nmol/L of the PDE2 inhibitor BAY 60–7550 in the cell bath.
Confocal imaging
Request a detailed protocolFreshly isolated cardiomyocytes were plated onto laminin coated coverslips and fixed in ice cold methanol for 10 min. Next, they were blocked with PBS based buffer containing 0.3% Triton X-100% and 5% fetal calf serum. Cells were then double stained overnight, at 4°C with primary antibodies, diluted in PBS containing 0.3% Triton and 1% BSA, for example against sGCα1 together with α-actinin or Caveolin-3. Cells were then washed three times for 5 min with PBS before they were exposed to secondary antibodies diluted in PBS containing 0.3% Triton and 1% BSA for 1 hr. Finally, cells were washed again in PBS three times for 5 min before being mounted onto cover slides with mounting medium. Imaging was performed using an inverted Zeiss LSM-800 laser scanning microscope equipped with a 40x oil immersion objective and controlled by ZEN imaging software which was used for the colocalization analysis and detection of the respective Pearson’s correlation coefficients.
Statistics
Statistical differences were analysed using OriginPro 8.6 and GraphPad Prism 7 software. Normal data distribution was determined by Kolmogorov-Smirnov test. For the comparison of two independent groups with skewed distribution Mann-Whitney U test was used. For the comparison of normally distributed data, a two-tailed T-test (for comparing morphometric and echocardiographic data from independent animals, Figure 1) or a mixed ANOVA followed by χ2-test (when data from several cells from multiple individual animals were compared) were applied. Differences were considered significant at p-values below 0.05. All data are presented as means ± s.e.m.
Data availability
All data generated or analysed during this study are included in the manuscript and supporting files. Source data files are provided for all figures.
References
-
sGC{alpha}1 mediates the negative inotropic effects of NO in cardiac myocytes independent of changes in calcium handlingAmerican Journal of Physiology. Heart and Circulatory Physiology 301:H157–H163.https://doi.org/10.1152/ajpheart.01273.2010
-
Expression, activity and functional significance of inducible nitric oxide synthase in the failing human heartJournal of the American College of Cardiology 32:955–963.https://doi.org/10.1016/S0735-1097(98)00336-2
-
Nitric oxide signalling in cardiovascular health and diseaseNature Reviews Cardiology 15:292–316.https://doi.org/10.1038/nrcardio.2017.224
-
Modulation of the endothelial nitric-oxide synthase-caveolin interaction in cardiac myocytes. implications for the autonomic regulation of heart rateThe Journal of Biological Chemistry 273:30249–30254.https://doi.org/10.1074/jbc.273.46.30249
-
NO-GC in cells 'off the beaten track'Nitric Oxide 77:12–18.https://doi.org/10.1016/j.niox.2018.03.020
-
The negative inotropic effect of beta3-adrenoceptor stimulation is mediated by activation of a nitric oxide synthase pathway in human ventricleJournal of Clinical Investigation 102:1377–1384.https://doi.org/10.1172/JCI2191
-
Transgenic mice for real-time visualization of cGMP in intact adult cardiomyocytesCirculation Research 114:1235–1245.https://doi.org/10.1161/CIRCRESAHA.114.302437
-
Nitric oxide synthase and cyclic GMP signaling in cardiac myocytes: from contractility to remodelingJournal of Molecular and Cellular Cardiology 52:330–340.https://doi.org/10.1016/j.yjmcc.2011.07.029
-
Comparative pharmacology of human beta-adrenergic receptor subtypes--characterization of stably transfected receptors in CHO cellsNaunyn-Schmiedeberg's Archives of Pharmacology 369:151–159.https://doi.org/10.1007/s00210-003-0860-y
-
Caveolae compartmentalise β2-adrenoceptor signals by curtailing cAMP production and maintaining phosphatase activity in the sarcoplasmic reticulum of the adult ventricular myocyteJournal of Molecular and Cellular Cardiology 52:388–400.https://doi.org/10.1016/j.yjmcc.2011.06.014
-
Phosphodiesterase-2 is Up-Regulated in human failing hearts and blunts β-Adrenergic responses in cardiomyocytesJournal of the American College of Cardiology 62:1596–1606.https://doi.org/10.1016/j.jacc.2013.05.057
-
Sepsis is associated with an upregulation of functional beta3 adrenoceptors in the myocardiumEuropean Journal of Heart Failure 9:1163–1171.https://doi.org/10.1016/j.ejheart.2007.10.006
-
The human beta 3-adrenergic receptor is resistant to short term agonist-promoted desensitizationMolecular Pharmacology 43:548–555.
-
Novel single chain cAMP sensors for receptor-induced signal propagationJournal of Biological Chemistry 279:37215–37218.https://doi.org/10.1074/jbc.C400302200
-
Rationale and design of a multicentre, randomized, placebo-controlled trial of Mirabegron, a Beta3-adrenergic receptor agonist on left ventricular mass and diastolic function in patients with structural heart disease Beta3-left ventricular hypertrophy (Beta3-LVH)ESC Heart Failure 5:830–841.https://doi.org/10.1002/ehf2.12306
-
Caveolin-3 knock-out mice develop a progressive cardiomyopathy and show hyperactivation of the p42/44 MAPK cascadeJournal of Biological Chemistry 277:38988–38997.https://doi.org/10.1074/jbc.M205511200
-
cGMP-phosphodiesterase activity is up-regulated in response to pressure overload of rat ventriclesBioscience, Biotechnology, and Biochemistry 67:973–979.https://doi.org/10.1271/bbb.67.973
Article and author information
Author details
Funding
British Heart Foundation (12/18/30088)
- Julia Gorelik
Wellcome (WT090594)
- Julia Gorelik
Deutsche Forschungsgemeinschaft (Fr 1725/3-2)
- Andreas Friebe
- Viacheslav O Nikolaev
National Institutes of Health (ROI-HL grant 126802)
- Julia Gorelik
Deutsche Forschungsgemeinschaft (NI 1301/3-2)
- Andreas Friebe
- Viacheslav O Nikolaev
The funders had no role in study design, data collection and interpretation, or the decision to submit the work for publication.
Acknowledgements
We thank Peter O’Gara for cardiomyocyte isolation. Confocal microscopy was performed at the FILM imaging facility of Imperial College London and at the Department of Pharmacology and Toxicology, University Medical Centre Hamburg-Eppendorf.
Ethics
Animal experimentation: All procedures performed in the UK were carried out according to the standards for the care and use of animal subjects determined by the UK Home Office (ASPA1986 Amendments Regulations 2012) incorporating the EU directive 2010/63/EU. The Animal Welfare and Ethical Review Body Committee of Imperial College London approved all protocols. The parts of the investigation, which were performed in Germany, conformed to the guide for the care and use of laboratory animals published by the National Institutes of Health (Bethesda, Maryland; Publication No. 85-23, revised 2011, published by National Research Council, Washington, DC). The experimental procedures were in accordance with the German Law for the Protection of Animals and with the guidelines of the European Community.
Copyright
© 2020, Schobesberger et al.
This article is distributed under the terms of the Creative Commons Attribution License, which permits unrestricted use and redistribution provided that the original author and source are credited.
Metrics
-
- 1,310
- views
-
- 223
- downloads
-
- 29
- citations
Views, downloads and citations are aggregated across all versions of this paper published by eLife.
Citations by DOI
-
- 29
- citations for umbrella DOI https://doi.org/10.7554/eLife.52221