Post-translational regulation of retinal IMPDH1 in vivo to adjust GTP synthesis to illumination conditions
Figures
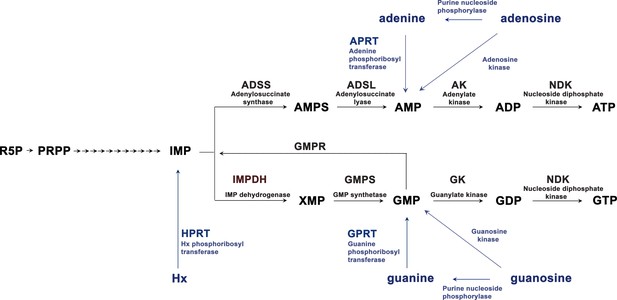
De novo and salvage pathways of purine synthesis in eukaryotic cells.
Metabolites and enzymes of the de novo pathway in black, and the salvage pathway in blue. R5P, ribose 5 -phosphate; PRPP, phosphoribosylpyrophosphate. The arrows from PRPP to IMP represent sequential enzymatic activities of the purinosome complex. IMPDH1 is responsible for the rate-limiting and first committed step in de novo GTP biosynthesis.
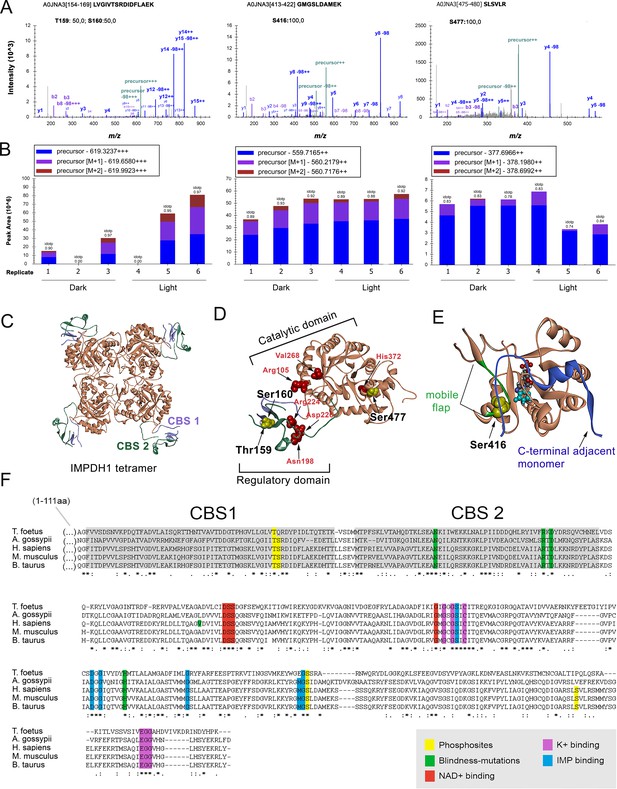
IMPDH1 phosphorylation sites identified in a phosphoproteomic study of dark/light-adapted bovine retinas.
(A) Mass spectra of identified IMPDH1 phosphorylated peptides. Residue numbers refer to the canonical bovine isoform β (514aa) [Uniprot A0JNA3]. For peptide 154–169 only one phosphorylation was detected, that could not be unequivocally assigned to T159 or S160. Phosphorylation at peptides 413–422 and 475–480 was at S416 and S477, respectively. (B) Peak areas of the precursor ions corresponding to the identified phosphopeptides of IMPDH1. Samples 1–3 are dark-adapted retinas; 4–6 light-exposed retinas (biological replicates). T159/S160 are preferentially phosphorylated in light, S477 in dark, and S416 indistinctly. (C) Ribbon diagram of IMPDH in its tetrameric conformation [pdb: 1jcn], showing the TIM barrel catalytic domain in coral, and the regulatory Bateman domain with two copies of cystathione beta-synthase (CBS) sequence in slate blue and green. (D) Monomer conformation [pdb: 1jcn] showing T159 and S160 at CBS1 in the Bateman domain; and S477 at the COOH-terminus. Disease associated mutations, depicted in red, are proximal to T159/S160 (Asn198; Arg224; Asp226) or to S477 (His372). (E) The catalytic domain of AgIMPDH, with the C319 loop in coral, the COOH-terminus of an adjacent monomer in blue and the mobile flap in green. IMP depicted in space fill model [pdb:4xfi]. (F) Alignment of IMPDH from T. foetus (prokaryotic), A. gossypii (filamentous fungus), H. sapiens, M. musculus and B. taurus IMPDH1 canonical isoforms. Phosphosites highlighted in yellow.
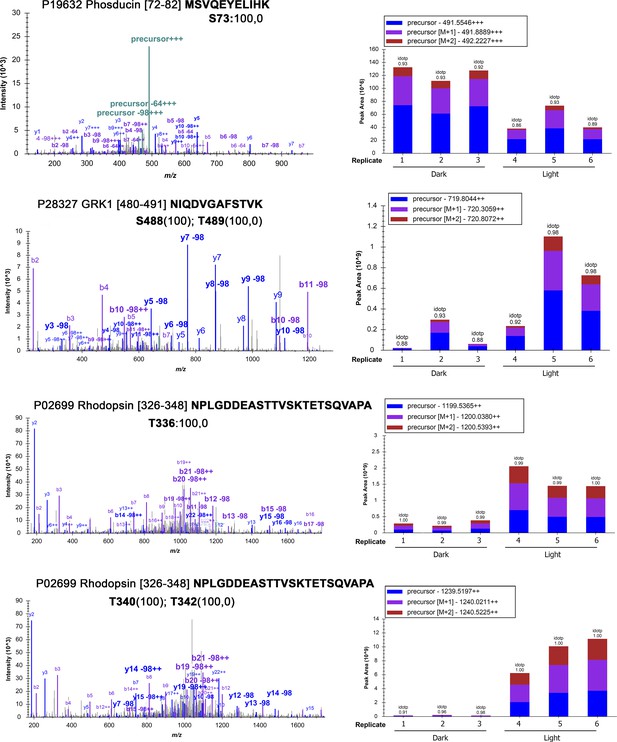
Dark/light fold-change of peak areas from control phosphopeptides from phosducin, GRK1 and rhodopsin shown as a quality control of the phosphoproteomic analysis.
Mass spectra of phosphorylated peptides of: phosducin 72–82 peptide MSVQEYELIHK [Uniprot F1MSE1]; GRK1 480–491 peptide NIQDVGAFSTVK [Uniprot P28327]; and rhodopsin 326–348 peptide NPLGDDEASTTVSKTETSQVAPA [Uniprot P02699]. For each peptide, the peak area of the precursor peptide ion was extracted and plotted for each sample. Samples 1–3 are dark-adapted retinas; 4–6 light-exposed retinas (biological replicates). Light/dark log2 fold-change values were: phosducin S73 (−1.39; p=0.01); GRK1 S488/T489 (2.70; p=0.08); Rhodopsin T336 (2.49; p=0); and Rhodopsin T340/T342 (5.53; p=0).
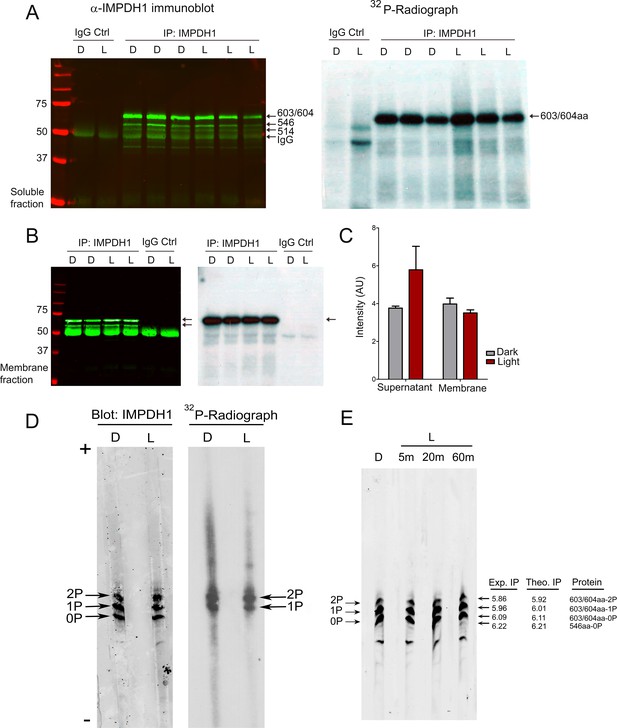
The predominant retinal spliced variant of IMPDH1 is phosphorylated to a high extent in intact retinas.
(A) Immunoblot of IMPDH1 immunoprecipitated from soluble fractions of 32P-labeled retinas, and corresponding autoradiograph. In situ metabolic labeling was performed in dim red light, with retinas from dark-adapted mice incubated in Locke´s with 32Pi for 90 min at 37°C, and then kept in the dark or exposed to light of 2000 lux for 5 min. IMPDH1 was immunoprecipitated in 3 biological replicates. 32Pi incorporation was observed at mIMPDH1-603/604aa isoform, in both dark and light conditions (autoradiograph). (B) Immunoblot and autoradiograph of IMPDH1 immunoprecipitated from membrane fractions. 32Pi incorporation was observed at IMPDH1-603/604aa, at dark and light conditions (2 biological replicates). (C) Average intensity of 32P-labeled 603/604aa bands normalized by immunoblot signal, for dark and light samples. IMPDH1 is substantially phosphorylated under dark and light, both in soluble and membrane fractions. A tendency to a higher phosphorylation in light in soluble fractions (p=0.180) and in dark in membrane fractions (p=0.308) was apparent, with no statistical significance. (D) Isoelectrofocusing (IEF) of immunoprecipitated IMPDH1 samples from 32Pi-labeled retinas revealed the presence of mono- and di-phosphorylated forms of IMPDH1-603/604aa in dark and light. Observed bands were: mIMPDH1-603/604-0P; mIMPDH1-603/604-1P and mIMPDH1-603/604-2P. Please note that the di-phosphorylated form incorporates 2 radiolabeled 32P atoms per protein molecule, and therefore the band intensity of the di-phosphorylated form in the autoradiograph reflects twice its abundance. (E) IEF of retinal homogenates (soluble fractions) from 16 hr dark-adapted mice or mice that were exposed to 5, 20 or 60 min of 2000 lux light after pupil dilation. Measured isoelectric points (IP) of bands correlated well with theoretical IP for the mIMPDH1 isoforms indicated.
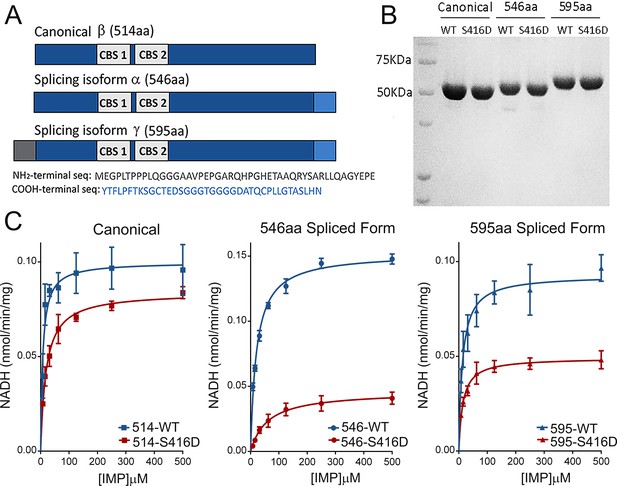
Effect of S416D phosphomimetic substitution on enzymatic activity.
(A) Main splice variants of hIMPDH1: hIMPDH1α (546aa) and hIMPDH1γ (595aa) contain extended sequences at the COOH- terminus (546aa) or both the NH2- and COOH-termini (595aa). (B) S416D mutant recombinant proteins were rigorously normalized to their wildtype counterparts preceding enzymatic analysis. (C) Effect of S416D substitution on the Michaelis-Menten kinetics of hIMPDH1β (514aa); hIMPDH1α (546aa); and hIMPDH1γ (595aa) isoforms. Mutation S416D increased the Km for IMP and decreased the Vmax significantly in hIMPDHα (546aa) and γ (595aa) splice variants, with the effect being maximal in the hIMPDH1α (546aa) isoform. Kinetic parameters were: hIMPDH1β (514aa) [Vmax = 0.099 ± 0.004, Km = 7.62 ± 1.69]; S416D/hIMPDH1β (514aa) [Vmax = 0.084 ± 0.002, Km = 19.13 ± 1.75]; hIMPDH1α (546aa) [Vmax = 0.152 ± 0.002, Km = 20.72 ± 1.32]; S416D/hIMPDH1α (546aa) [Vmax = 0.046 ± 0.002, Km = 59.59 ± 9.74]; hIMPDH1γ (595aa) [Vmax = 0.093 ± 0.003, Km = 12.86 ± 2.18]; S416D/hIMPDH1γ (595aa) [Vmax = 0.049 ± 0.001, Km = 13.95 ± 1.71]. Results represent the media and S.E.M of three independent experiments with three technical replicates each.
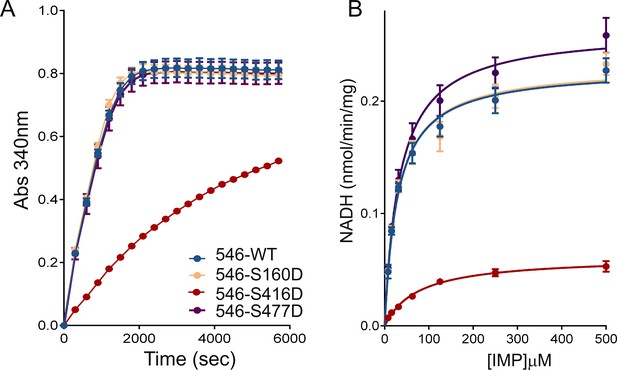
Effect of phosphomimetic substitutions on enzymatic activity.
(A) Reaction kinetics of wildtype; S160D; S416D and S477D mutants of hIMPDH1α (546aa). Assays were performed with 3,6 μM of recombinant protein in assay buffer with 125 mM IMP and 0.5 mM NAD+. Results represent the mean and S.E.M of three independent experiments with three technical replicates each. S160D and S477D mutants showed wildtype kinetics; while S416D mutant showed delayed kinetics. (B) Michaelis-Menten plots for the wildtype and phosphomimetic mutants. NADH production plotted to concentration of IMP. Assays were performed at 0.5 mM NAD+ and varying concentrations of IMP, from 0 to 500 μM. NADH production (nmol/min/mg) was obtained from ΔAbs/Δt by applying Lambert- Beer’s law (εNADH340nm=6220M−1cm−1, l = 0.58 cm), and results were fitted to Michaelis-Menten curves with GraphPad Prism [Mean and S.E.M of three independent experiments, three technical replicas each].
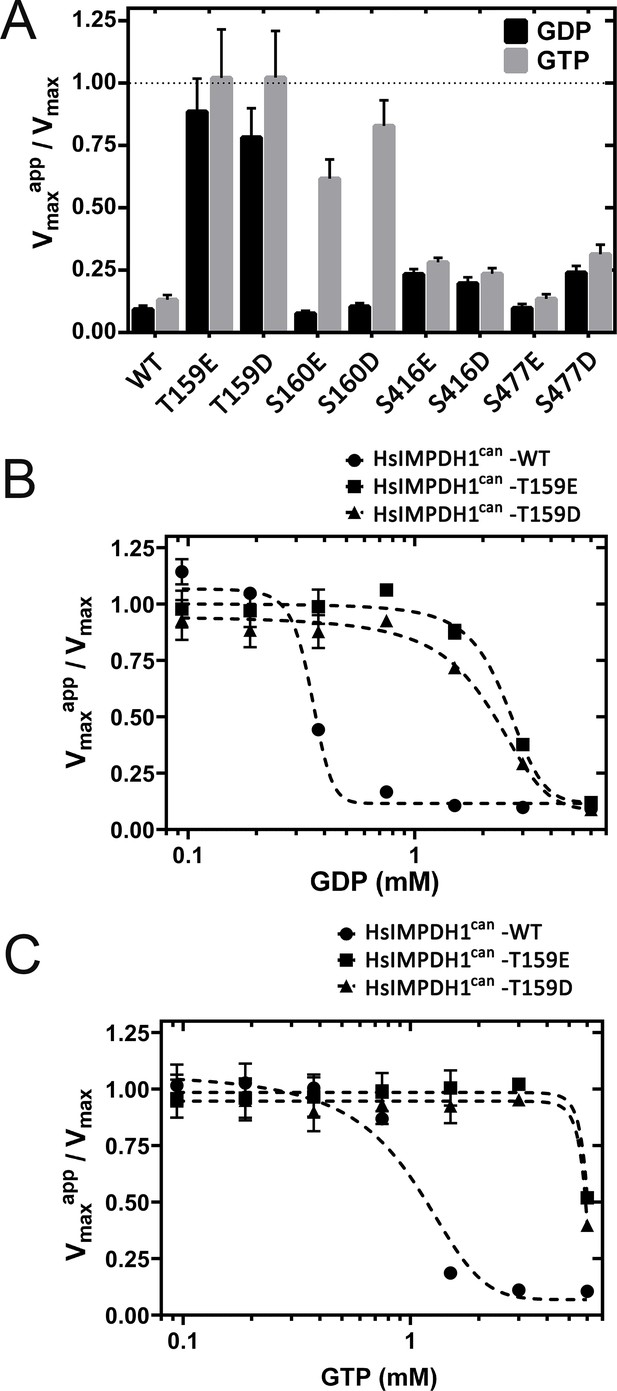
Effect of phosphomimetic mutations on GDP and GTP allosteric regulation of IMPDH1 activity.
(A) Vmaxapp/Vmax for the indicated phosphomimetics mutants. (B) Vmaxapp/Vmax for the T159E and T159D mutants, as a function of GDP concentration. (C) Vmaxapp/Vmax for the T159E and T159D mutants, as a function of GTP concentration. Results shown are representative of two independent experiments.
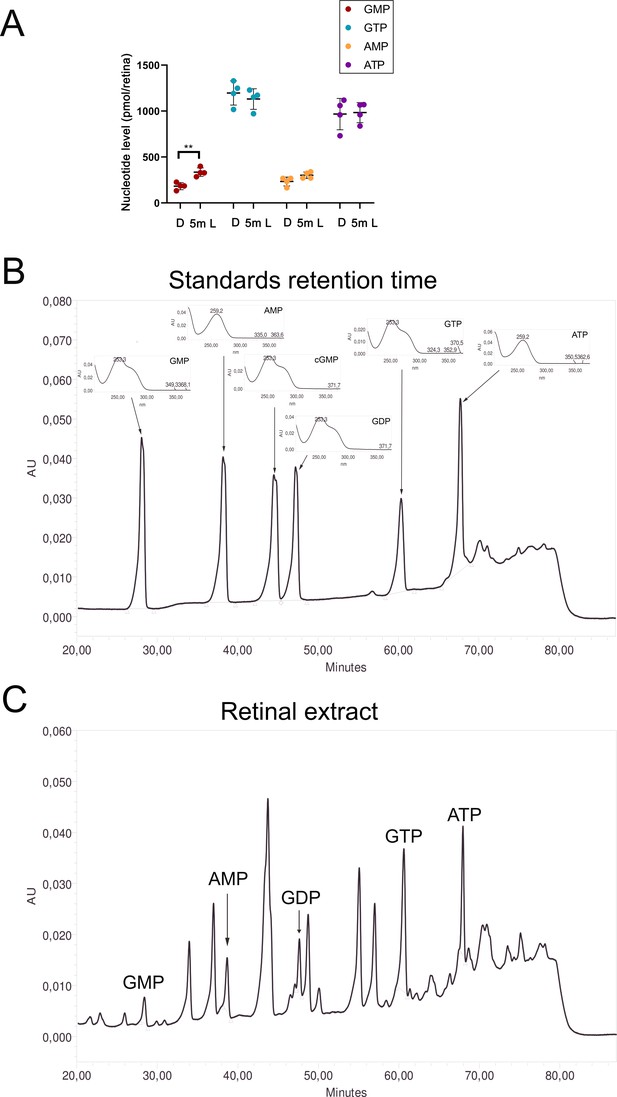
HPLC determination of nucleotide levels in dark-adapted or light-exposed in situ retinas.
(A) Nucleotide levels (pmol/retina) in whole retinas obtained from dark-adapted mice that were maintained in DMEM in situ for 5 min, either in darkness or exposed to 2,000 lux white light. Note that GMP levels increased with light exposure, while GTP and ATP levels were maintained. Individual values and the Mean ± STDEV is indicated (4 biological replicates). Unpaired t-test for GMP values in dark and light resulted in p=0.002; while other nucleotides showed not significant changes between dark and 5 min light. Note that GTP levels were higher than ATP levels in the retina, both in dark and light conditions. (B) Chromatogram of a mix of nucleotide standards, showing each nucleotide retention time and typical spectral sensitivity. (C) Representative chromatogram of a retinal extract showing the retention time and relative abundances of the different nucleotides. cGMP was below detection in retinal extracts.
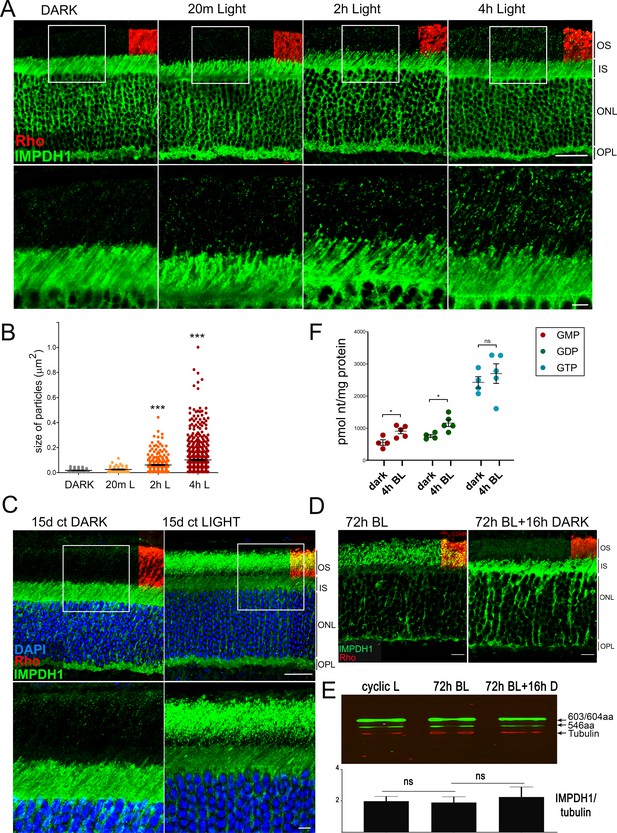
Accumulation of IMPDH1 aggregates at the outer segment layer in living mice exposed to constant bright light.
(A) Murine retinal sections from dark-adapted mice or mice exposed to 1600lux-light for 20 m, 2 hr or 4 hr were immunostained for IMPDH1 (green) and co-immunostained for rhodopsin (red). Confocal images are average z-projections of 5 optical slices with 0.13μm-step size. Magnified frames from the outer segment layer show gradual accumulation of IMPDH1 aggregates with time of bright light exposure. (B) Size and number of IMPDH1 aggregates with time (Dark vs 2 hr light, p<0.0001; Dark versus 4 hr light, p<0.0001). Results are representative of two independent experiments. (C) Retinal sections from constant dark or 1600 lux constant light-reared mice for 15d, immunostained for IMPDH1 (green) and rhodopsin (red). Magnified inner/outer segment layers show a prominent accumulation of IMPDH1 signal at the outer segment layer, representing 30% of the total IMPDH1 signal in the retina. A basal IMPDH1 signal is already detected at the outer segment layer in dark-adapted mice. Results shown are representative of results obtained with two independent groups of mice. (D) IMPDH1 aggregate formation at the outer segment by 72 hr of bright light could be reversed by subsequent dark-adaptation (16 hr). Shown is a representative result observed in three independent groups of mice. (E) IMPDH1 expression in the retinas from these three groups of mice does not change, indicating that changes in IMPDH1 signal at the outer segment layer are due to protein translocation rather than to transcriptional regulation. (F) Retinal GMP, GDP and GTP levels (pmol/mg prot) determined by HPLC from retinal extracts from mice that had been dark-adapted or exposed to bright light (BL, 1600 lux) for 4 hr. GMP and GDP levels increased with light [p=0.021 for GMP and p=0.016 for GDP, with n = 4] whereas the GTP levels were maintained [p=0.802 for GTP, with n = 4]. OS: outer segment, IS: inner segment, ONL: outer nuclear layer, OPL: outer plexiform layer. Scale bar upper panels (20 μm) and bottom panels (5 μm).
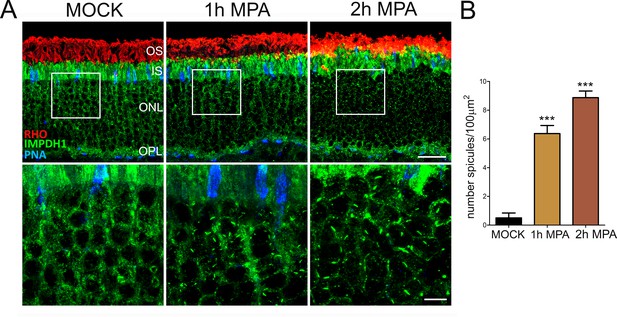
Formation of IMPDH1 spicules in intact retinas in response to mycophenolic acid-treatment.
(A) Murine retinal sections from dark-adapted mock- or 10 μM MPA-treated retinas for 1 or 2 hr. MPA inhibition of IMPDH1 resulted in the formation and accumulation of IMPDH1 spicules with time, particularly noticeable at the perinuclear region of photoreceptor cells (8.5x Zoom images) at the outer nuclear layer. IMPDH1 immunolocalization shown in green; PNA staining of cones in cyan. Confocal images are the average z-projections of three optical slices acquired with a step size of 0,13 μm. (B) Histogram showing the number of spicules/100 μm2. (Mock vs 1 h MPA, p=0.0001; MOCK vs 2 h MPA p<0.0001), with at least 6 planes from 2 animals analyzed per condition. OS: outer segment, IS: inner segment, ONL: outer nuclear layer, OPL: outer plexiform layer. Scale bar upper panels (20 μm) and bottom panels (5 μm).
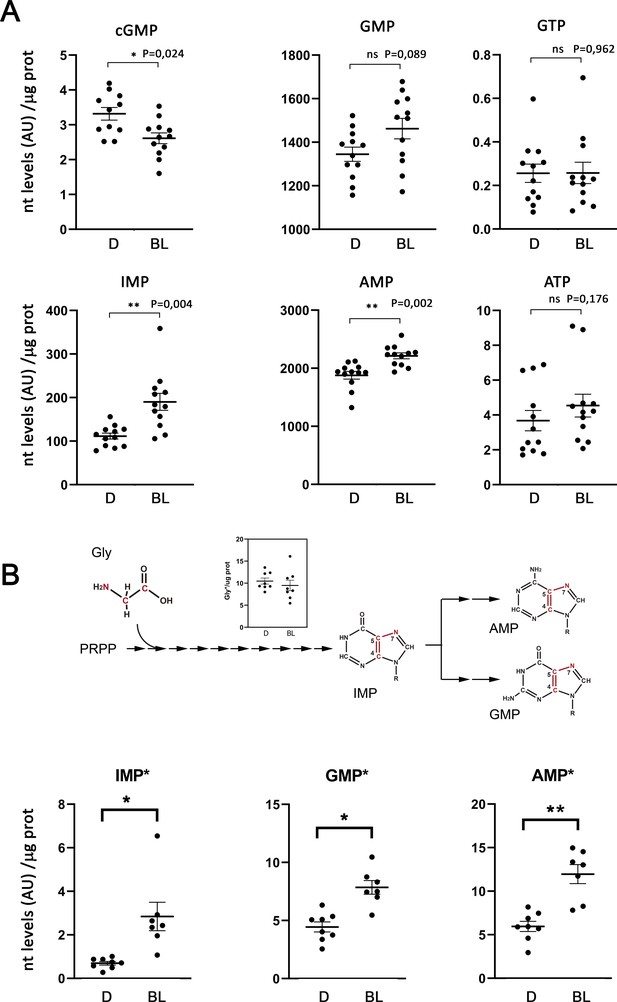
Light exposure increases the flux through de novo purine nucleotide synthesis in the retina.
Metabolic flux analysis through de novo purine nucleotide synthesis was performed by injecting 13C2;15N-Glycine intravitreally (to 4 mM final concentration in the vitreous) to dark-adapted mice, that were then kept in the dark or exposed to 1600 lux light for 4 hr. Total nucleotide levels and labeled nucleotide levels (that incorporated the labeled carbon and nitrogen atoms from labeled glycine) were determined by LC/MS-MS, and normalized per μg of protein in each sample. (A) Retinal levels of total cGMP, IMP, AMP, GMP, ATP and GTP in dark-adapted mice (D); or bright-light-exposed mice (BL). Nucleotide levels are expressed in arbitrary units (LC-MS/MS peak integration values) normalized per μg of protein in each sample. cGMP levels decreased with light exposure, as expected [p=0,024, n = 12]. IMP levels showed a clear increase with light exposure [p=0004]; that was accompanied by an increase in AMP and GMP levels [p=0002 for AMP; p=0089 for GMP]; whereas the ATP and GTP levels were maintained. (B) Retinal levels of labeled IMP, GMP and AMP, normalized per μg prot. The labeled atoms from Gly* that are incorporated into the purine ring scaffold are shown in the diagram. The inset on the diagram shows that the levels of Gly* measured at the retinas of injected eyes were similar in dark and bright light samples. Incorporation of Gly* into IMP, AMP and GMP increased under bright-light exposure, indicative of an increase in the overall flux of de novo purine nucleotide synthesis [IMP*, p=0,020; AMP*, p=0,003; GMP*, p=0,013, with n > 7]. In four eyes of the ‘dark’ group of mice, and five eyes of the ‘bright light’ group of mice the injection failed [the injected Gly* did not reach the retina, and Gly*~0 in retinal extracts] and therefore could not be taken into account for determination of labeled nucleotides.
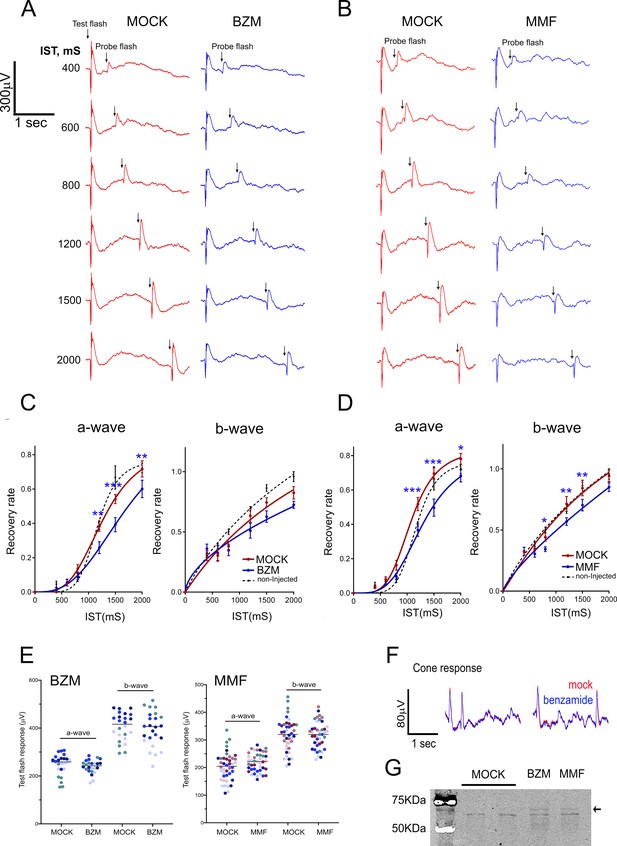
Effect of IMPDH inhibition on mass rod response recovery.
(A) Simultaneous electroretinogram (ERG) recordings from both eyes of a mouse injected with benzamide (BZM, non-competitive IMPDH inhibitor) or drug dilution buffer (mock), in response to a probe flash triggered at increasing interstimulus times (IST) after a test flash. A slight but statistically significant delay in the recovery of the a-wave is observed at three interstimulus times. (B) A similar result is obtained when mycophenolate mofetil (MMF) -an ester prodrug of mycophenolic acid (MPA)- is injected instead of benzamide riboside. (C) Statistical analysis of mass rod response recovery (recovery rate plotted to interstimulus time, ERG paired flash paradigm) in benzamide-injected eyes versus corresponding control eyes. Overimposed black dashed lines are the curves obtained for control non-injected mice. Two-way ANOVA (mock vs drug injections and IST as factors) with uncorrected Fisher´s test for multiple comparisons, a-wave benzamide vs mock [1200 ms (p=0.0027); 1500 ms (p=0.0004); 2000 ms (p=0.0046), 4 mice analyzed]. (D) Statistical analysis for mycophenolate mofetyl (MMF)-injected mice: MMF vs mock [1200 ms (p=0.0002); 1500 ms (p<0.0001); 2000 ms (p=0.0132), 7 mice analyzed]. (E) Statistical comparison of a- and b-wave amplitudes of the test flash in the mock-injected left eye versus the drug-injected right eye in four BZM mice and seven MMF mice. Each mouse shown in a different colour. Mean a-wave and b-wave of the test flash (and SD) were: a-wave [MOCK: 246.8 ± 44.5 and BZM: 239.1 ± 28.2, n = 4]; and b-wave [MOCK: 405.0+58.5 and BZM: 395.0 ± 73.1]; and a-wave [MOCK: 203.0 ± 51.1 and MMF: 218.8 ± 33.4, n = 7]; and b-wave [MOCK: 318.3+58.9 and MMF: 318.2 ± 47.3]. (F) Superimposed pure-cone responses of the same mouse. (G) IMPDH1 mobility by SDS-PAGE is shifted by both benzamide and MMF in retinal extracts from intravitreally injected eyes.
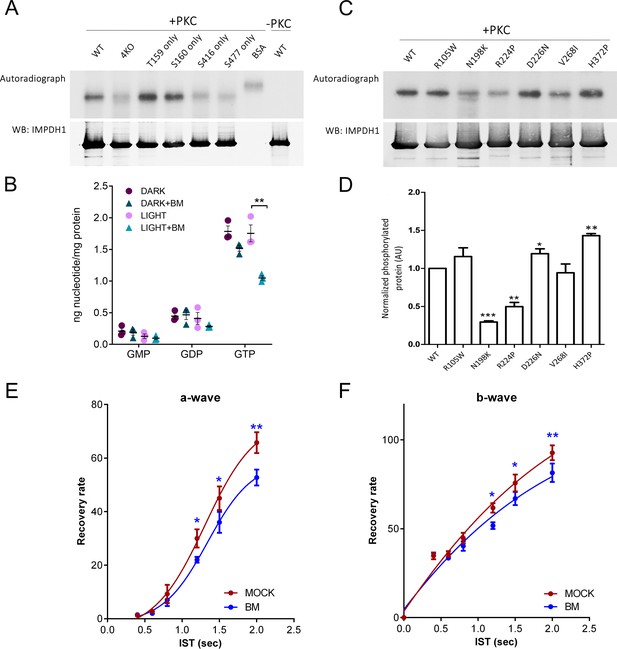
PKC phosphorylates T159 and S160 of hIMPDH1 in in vitro phosphorylation assays.
(A) In vitro phosphorylation assays with PKCα kinase of hIMPDH1α (546aa); T159G/S160G/S416G/S477G-hIMPDH1α (546aa) (4KO); and forms of the protein with phosphorylation sites individually restored. 5 μg of purified protein were incubated with 4 μg/ml recombinant hPKCα in assay buffer with 0.05 mM PMA and 1 μl of 32P-γ-ATP (3000 Ci/mmol), for 30 min at 30°C. Samples were resolved by SDS-PAGE, transferred to a nitrocellulose membrane, exposed to an X-ray film (autoradiograph), and immunoblotted for IMPDH1. PKC phosphorylated hIMPDH1α (546aa) selectively at T159 and S160. Results shown are representative of two independent experiments. (B) GMP, GDP and GTP nucleotide levels (nmol nt/mg of retinal protein) determined by ion-pairing high performance liquid chromatography (HPLC) in in situ dark-adapted or light-exposed retinas. Individual values and the Mean ± STDEV are indicated (3 biological replicates). In light-exposed retinas, GTP levels decreased substantially in the presence of 50 mM bisindolylmaleimide (unpaired t-test p=0.007); while other nucleotides did not show statistically significant changes between the dark and light conditions (n = 3). (C) In vitro PKC phosphorylation assay of hIMPDH1α (546aa) disease mutants R105W; N198K; R224P; D226N; V268I; H372P. (D) Average intensity of autoradiograph signal normalized by the immunoblot signal for blindness-associated mutations, expressed as a function of the wildtype levels. N198K and R224P mutations resulted in a decrease of phosphorylation [p=0.0001 and p=0.004 versus the wildtype, n = 3 independent experiments]; while H372P led to an statistically significant increase in phosphorylation [p=0.0013, n = 3 independent experiments]. (E–F) Mass rod response recovery (recovery rate plotted to interstimulus time, ERG paired flash paradigm) in bisindolylmaleimide-injected eyes (to 1,25 μM final concentration in the vitreous) versus corresponding control eyes. Two-way ANOVA (mock vs drug injections and IST as factors) with uncorrected Fisher´s test for multiple comparisons, a-wave bisindolylmaleimide vs mock [1200 ms (p=0.0468); 1500 ms (p=0.0263); 2000 ms (p=0.0019), 4 mice analyzed]; and b-wave bisindolylmaleimide vs mock [1200 ms (p=0.0182); 1500 ms (p=0.0374); 2000 ms (p=0.0085), 4 mice analyzed].
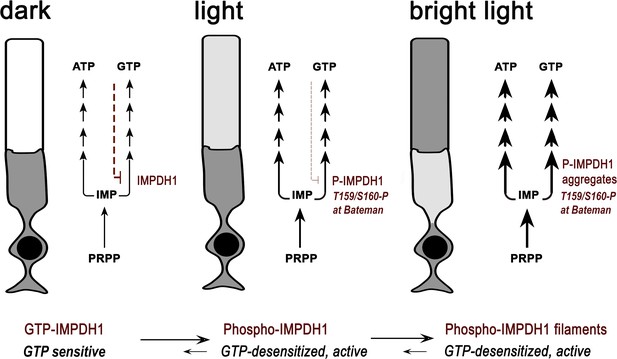
Proposed model of regulation of IMPDH1 activity in photoreceptor cells by light-dependent phosphorylation at T159/S160.
In the dark, IMPDH1 is sensitive to GTP. GTP can bind to the nucleotide binding sites at the Bateman domain and shift IMPDH1 conformation towards GTP-bound IMPDH1 compacted octamers that are inactive (negative feedback regulation in red). Upon light exposure, phosphorylation at T159/S160 desensitizes the enzyme to GTP, coinciding in time with the increase in IMP levels. We propose that these concurring conditions would cause filamentation of the enzyme, leading to protein aggregation at the outer segment layer. In this way, light exposure would stabilize IMPDH1 in an active conformation permissive to the increased flux towards guanine and adenine nucleotide synthesis (thicker arrows in light). This increased flux is likely required to compensate for ATP and GTP consumption in the phototransduction process, to sustain the pools of ATP and GTP. IMPDH1 localization at the outer segment layer in these macrostructures would be reverted by dephosphorylation and gradual decrease of IMP levels during dark-adaptation. The model would predict a concerted modulation of ADSS/ADSL and the purinosome.
Tables
K1/2 values (mM) for GDP and GTP.
Enzyme kinetics data were fitted by non-linear regression to the Michaelis-Menten equation to derive Vmax and KM values. The Vmax values versus GTP/GDP concentration were then adjusted to a sigmoidal dose-response function using the GraphPad software package.
GDP | GTP | |
---|---|---|
HsIMPDH1β (514aa)-WT | 0.45 ± 0.03 | 0.97 ± 0.1 |
HsIMPDH1β (514aa)-T159D | 2.19 ± 0.2 | ≈ 6 |
-
K1/2 values are given in mM units (Mean ± SD), and are representative of two independent experiments.
Nucleotide levels in dark- or light-adapted murine retinas, determined by HPLC.
Retinas were obtained from 16 dark-adapted mice that were either kept in the dark for 4 hr, or exposed to 4 hr bright light (1600 lux). HPLC determinations, with numbers indicating Mean ± S.E.M, with n = 4 biological replicates.
Pmol/mg protein | ||
---|---|---|
DARK | 4 hr BL | |
GMP | 557.21 ± 88.5 | 916.31 ± 81.1 |
GDP | 762.57 ± 47.8 | 1174.11 ± 109 |
GTP | 2461.53 ± 178.1 | 2737.29 ± 310.6 |
AMP | 731.84 ± 125.4 | 1246.16 ± 69.8 |
ATP | 1579.69 ± 110.5 | 2759.18 ± 226 |
GTP/ATP | 1.56 | 0.99 |
Multiple reaction monitoring (MRM) transitions in LC-QqQ MS.
Compound | RT (min) | Quantitation transition (CE) | Confirmation transition (CE) |
---|---|---|---|
AMP | 3.21 | 348 → 136 (16) | 348 → 119 (56) |
AMP* | 3.21 | 351 → 139 (16) | 351→ 122 (56) |
ATP | 5.91 | 508 → 136 (32) | 508 → 410 (12) |
ATP* | 5.91 | 511 → 139 (32) | 511 → 413 (12) |
GMP | 4.60 | 364 → 152 (8) | 364 → 135 (52) |
GMP* | 4.60 | 367 → 155 (8) | 367 → 138 (52) |
GDP | 6.13 | 444 → 152 (32) | 444 → 135 (60) |
GDP* | 6.13 | 447 → 155 (32) | 447 → 138 (60) |
GTP | 7.07 | 524 → 152 (32) | 524 → 135 (56) |
GTP* | 7.07 | 527 → 155 (32) | 527 → 138 (56) |
cGMP | 1.38 | 346 → 152 (12) | 346 → 135 (54) |
cGMP* | 1.38 | 349 → 155 (12) | 349 → 138 (54) |
IMP | 3.82 | 349 → 137 (16) | 349 → 110 (60) |
IMP* | 3.82 | 352 → 140 (16) | 352 → 113 (60) |
Gly | 1.79 | 76 → 30 (8) | 76 → 58 (20) |
Gly* | 1.79 | 79 → 32 (8) | 79 → 61 (20) |
-
*labeled (isotopologue M+3); RT: retention time; CE: collision energy (eV).