Downregulation of the tyrosine degradation pathway extends Drosophila lifespan
Abstract
Aging is characterized by extensive metabolic reprogramming. To identify metabolic pathways associated with aging, we analyzed age-dependent changes in the metabolomes of long-lived Drosophila melanogaster. Among the metabolites that changed, levels of tyrosine were increased with age in long-lived flies. We demonstrate that the levels of enzymes in the tyrosine degradation pathway increase with age in wild-type flies. Whole-body and neuronal-specific downregulation of enzymes in the tyrosine degradation pathway significantly extends Drosophila lifespan, causes alterations of metabolites associated with increased lifespan, and upregulates the levels of tyrosine-derived neuromediators. Moreover, feeding wild-type flies with tyrosine increased their lifespan. Mechanistically, we show that suppression of ETC complex I drives the upregulation of enzymes in the tyrosine degradation pathway, an effect that can be rescued by tigecycline, an FDA-approved drug that specifically suppresses mitochondrial translation. In addition, tyrosine supplementation partially rescued lifespan of flies with ETC complex I suppression. Altogether, our study highlights the tyrosine degradation pathway as a regulator of longevity.
Introduction
Aging is the primary risk factor for many major human pathologies, including cancer, diabetes, cardiovascular disorders, and neurodegenerative diseases (López-Otín et al., 2013). Untargeted and targeted metabolomics analysis in worms (Fuchs et al., 2010), flies (Hoffman et al., 2014; Avanesov et al., 2014; Parkhitko et al., 2016), mice (Tomás-Loba et al., 2013), and humans (Yu et al., 2012) have documented changes in the metabolome during the aging process. Manipulations of metabolic pathways that change with age might suppress aging and extend lifespan (Parkhitko et al., 2020). For example, perturbation of mitochondrial function (Copeland et al., 2009; Owusu-Ansah et al., 2013; Fridell et al., 2005), activation of the pentose phosphate pathway (Legan et al., 2008), suppression of purine nucleotide metabolism (Stenesen et al., 2013), suppression of fatty acid oxidation (Mourikis et al., 2006), and inhibition of glycogen metabolism (Sinadinos et al., 2014; Post et al., 2018) have been shown to extend lifespan. Moreover, interventions that are known to extend lifespan, like dietary restriction, genetic selection or manipulations of specific pathways might reverse age-dependent metabolic reprogramming (Laye et al., 2015). In addition, key master regulators of metabolism such as DILPs/Insulin signaling (Post et al., 2019; Bai et al., 2012; Broughton et al., 2005; Grönke et al., 2010; Clancy et al., 2001), Tor (Kapahi et al., 2004), AMPK (Ulgherait et al., 2014; Burkewitz et al., 2014), JNK (Wang et al., 2003), Spargel (Rera et al., 2011), Nrf2 (Sykiotis and Bohmann, 2008), Activin/TGFβ (Bai et al., 2013), and Sirt4 (Wood et al., 2018) are known to extend lifespan, although whether they extend lifespan via their effect on metabolism or other processes is unknown. While numerous studies have shown that metabolism changes with age and the role of several metabolic pathways in aging has been characterized, we do not completely understand what drives these metabolic alterations and how they affect other biological processes.
Amino acids serve as building blocks for proteins and fuel different metabolic pathways. In addition to a well-known role of amino acids in lifespan extension by dietary restriction, manipulating metabolism of specific amino acids can extend lifespan in flies and other organisms. For example, methionine restriction (Lee et al., 2014) or activation of methionine flux (Parkhitko et al., 2016; Parkhitko et al., 2019) prolongs health and lifespan in flies and other species. Increased homocysteine processing via overexpression of cystathionine β-synthase (dCBS) extends Drosophila lifespan and is required for the beneficial effects of dietary restriction (Kabil et al., 2011). Genetic and pharmacological impairment of the tryptophan/kynurenine pathway promotes Drosophila lifespan (Oxenkrug et al., 2012) and tryptophan restriction extends rat lifespan (Ooka et al., 1988). Impairing threonine catabolism via glycine-C-acetyltransferase suppression promotes Caenorhabditis elegans lifespan (Ravichandran et al., 2018). Glycine supplementation can extend lifespan in both C. elegans (Liu et al., 2019) and mice (Miller et al., 2019). Despite the accumulating evidence, the role of non-proteogenic metabolism of specific amino acids in the regulation of aging and lifespan and their mechanisms are still poorly characterized.
One approach to identify new traits responsible for aging is to compare how these traits change with age in control and long-lived animals of the same species (Milman and Barzilai, 2016). For example, centenarians have a distinctive epigenetic profile compared to an age-matched control population (Horvath et al., 2015). Similarly, we previously showed that flies with increased longevity have dramatic differences in many metabolites associated with methionine metabolism even at 1 week of age when 100% of both control- and long-lived flies are still alive (Parkhitko et al., 2016). To identify novel metabolic pathways that correlate with lifespan and that can be responsible for aging, we compared the metabolome of 1-week- and 4-week-old wild-type and long-lived flies to identify changes in metabolites that correlate with lifespan and identified tyrosine as an age-dependent metabolite. We demonstrate that Drosophila has a single tyrosine aminotransferase (TAT). Whole-body or neuronal-specific downregulation of TAT as well as other downstream enzymes in the tyrosine degradation pathway significantly extend Drosophila lifespan, cause alterations of multiple metabolites associated with increased lifespan, and lead to an increase in tyrosine and tyrosine-derived neuromediators (dopamine, octopamine, and tyramine). We further demonstrate that mitochondrial dysfunction may serve as an age-dependent stimulus that redirects tyrosine from neuromediator production into mitochondrial metabolism. In conclusion, our studies highlight the important role of the tyrosine degradation pathway and position TAT as a link between neuromediator production, dysfunctional mitochondria, and aging.
Results
Age-dependent changes in tyrosine levels
We previously demonstrated that many metabolites associated with methionine metabolism, a metabolic pathway playing a key role in regulation of aging, are dramatically different between 1-week-old wild-type and long-lived flies (Parkhitko et al., 2016). These long-lived flies have been selected for delayed reproductive senescence over 170 generations and maintained on a generation interval of 70 days, while control lines were maintained on a 2-week generation interval (Carnes et al., 2015). To further extend our metabolic analysis and reveal new metabolites involved in the regulation of aging and lifespan, we compared differences in metabolomes in 1-week and 4-week-old wild-type (B3) and long-lived (O1 and O3) flies, searching for metabolites that are either different between control vs. long-lived flies of the same age and/or metabolites that change differently with age between control vs. long-lived flies. Although longevity is not the only trait that is different between these lines and these metabolites can be linked to other traits such as reproduction; we used this list of candidate metabolic pathways for the further analysis in wild-type flies. Including metabolites in methionine metabolism, we identified 49 metabolites whose changes were significantly different with age between control and both lines of long-lived flies (Figure 1A). While many of these metabolites belong to the metabolic pathways that have been previously described as being important players in the regulation of aging and longevity (tryptophan metabolism, NADPH, nucleotide metabolism etc.), many of them have not been studied before, including tyrosine.
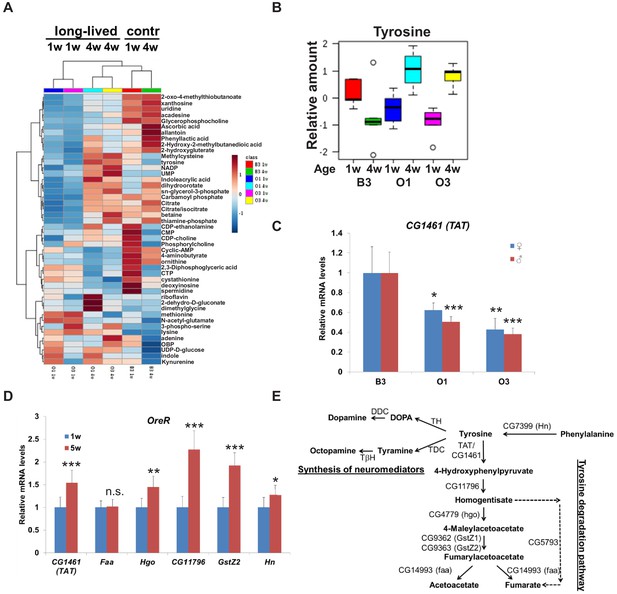
Tyrosine is a new lifespan-dependent metabolite.
(A) Heat map showing the metabolites that significantly changed in 1-week and 4-week-old wild-type (B3) and long-lived (O1 and O3) flies. Each row represents a mean of five biological replicates. (B) Box plots of relative levels of tyrosine in 1-week and 4-week-old wild-type (B3) and long-lived (O1 and O3) flies extracted from the heat map (A). (C) Relative mRNA levels of CG1461 in 1-week-old control (B3) and long-lived (O1, O3) flies. Means ± SD. (D) Relative mRNA levels of CG1461, Faa, Hgo, CG11796, GstZ2, and Hn from 1-week and 5-week-old wild-type (OreR) flies. Means ± SD. (E) Tyrosine metabolism pathway. *p<0.05, **p<0.01, ***p<0.001.
Interestingly, levels of tyrosine significantly increased with age in both lines of long-lived flies (Figure 1B). In addition to its proteogenic function, tyrosine can be used either as a precursor for the synthesis of neuromediators, such as dopamine, octopamine, and tyramine, or can be degraded via the tyrosine degradation pathway producing acetoacetate and fumarate (Figure 1E). The first and rate-limiting enzyme in the tyrosine degradation pathway is tyrosine aminotransferase (TAT). Based on the DIOPT ortholog prediction tool (Hu et al., 2011), Drosophila has a single TAT ortholog, CG1461. We tested whether levels of CG1461/TAT were different between control and long-lived flies. Strikingly, mRNA levels of CG1461/TAT were significantly decreased in both long-lived O1 and O3 female and male flies compared to B3 control flies (Figure 1C), suggesting that TAT levels may explain age-dependent differences in the level of tyrosine.
Next, we examined CG1461/TAT expression levels in young (1 w) vs. old (5 w) wild-type Oregon R (OreR) flies and detected a significant mRNA increase in older flies (Figure 1D). Consistent with this observation, using a GFP-tagged transgenic line from the fly-TransgeneOme (fTRG) library (Sarov et al., 2016), we observed an increase in TAT with age (Figure 1—figure supplement 1). In addition, mRNA levels of other enzymes in the tyrosine degradation pathway, Hgo, CG11796, GstZ2, and Hn, were significantly increased with age in wild-type flies (Figure 1D,E). Altogether, these findings suggest that tyrosine catabolism increases during aging.
Supplementing tyrosine extends lifespan and CG1461 functions as TAT to cope with high tyrosine levels
TAT catalyzes the conversion of tyrosine to 4-hydroxyphenylpyruvate and is the first and rate-limiting enzyme in the tyrosine catabolic pathway (Figure 1E). We first tested whether the Drosophila ortholog of TAT, CG1461, is involved in tyrosine degradation. We confirmed the knockdown efficiency of three independent CG1461 RNAi lines by qRT-PCR (CG1461 RNAi-1, ~50% [weak line]; CG1461 RNAi-2 and 3, ~80% [strong lines]) (Figure 2A) and generated CG1461-deficient flies using CRISPR/Cas9 (Figure 2B). To test the functional significance of CG1461 for tyrosine degradation, we ubiquitously downregulated CG1461 in adult flies using the tubulin-Gal4, tubulin-Gal80ts temperature-inducible system. Gal80ts is active at 18°C and represses Gal4, whereas at 29°C, Gal80ts is inactivated, allowing Gal4-dependent expression of CG1461 RNAi. Flies were grown at 18°C, switched to 29°C after eclosion to induce expression of CG1461 RNAi and after 2 weeks switched on food supplemented with 5 g/L of tyrosine, which represents approximately a 5- to 10-fold increase of tyrosine compared to regular food (Piper, 2017). Extra tyrosine caused acute toxicity and death when CG1461 expression was downregulated and the rate of death correlated with the strength of the RNAi lines, suggesting that CG1461 is critical in the degradation of excess tyrosine (Figure 2C). Similarly, when we switched CG1461 wild-type, heterozygous, or mutant flies on food supplemented with 5 g/L of tyrosine it caused acute toxicity and death of CG1461 mutant but no acute toxicity was observed in wild-type or heterozygous flies (Figure 2D). We further tested a range of different concentrations of tyrosine (1X, 2.5X, 5X, and 10X) and observed a gradual and significant decrease in the lifespan of mutant flies (−53%, −54%, −39%, −20% for 10X, 5X, 2.5X, and 1X concentrations of tyrosine in male flies and −69%, −67%, −48%, −37% for 10X, 5X, 2.5X, and 1X concentrations of tyrosine in female flies, respectively) (Figure 2—figure supplement 1A,B); while the lifespan was either non-affected in male wild-type flies (Figure 2—figure supplement 1A), or decreased at the highest (5X and 10X) concentrations by a much lower extent in female wild-type flies (−38% and −24% for 10X and 5X concentrations of tyrosine) (Figure 2—figure supplement 1B). In addition, accordingly with the beneficial role of tyrosine, feeding flies with low concentrations of tyrosine significantly increased their lifespan by 9% in male flies (1X concentration of tyrosine, p<0.0001, log-rank test) (Figure 2—figure supplement 1A) and by 11.5% in female flies (1X concentration of tyrosine, p=0.002, log-rank test) (Figure 2—figure supplement 1B). Tyrosine is a conditionally essential amino acid because it can be synthetized from phenylalanine (but not vice versa). To examine whether CG1461 regulates levels of tyrosine and phenylalanine, we performed metabolomic profiling of CG1461 wild-type and mutant flies fed with either control or high-tyrosine diets. Feeding control flies with tyrosine increased the level of tyrosine and phenylalanine because phenylalanine is degraded via its conversion into tyrosine (Figure 2E,F); however, CG1461 mutant flies on control diet had significantly higher levels of tyrosine, suggesting that a significant portion of tyrosine from regular food undergoes degradation via the tyrosine degradation pathway. Moreover, feeding CG1461 mutant flies with tyrosine further increased levels of tyrosine and phenylalanine (Figure 2E,F) and caused their death (Figure 2D). We further tested whether the level of a GFP-tagged CG1461 changed in response to high tyrosine feeding, as we previously observed increased levels of CG1461 with age. Feeding flies with 5 g/L of tyrosine increased the level of GFP-tagged CG1461 (Figure 2—figure supplement 1C). Interestingly, male flies had higher levels of GFP-tagged CG1461 both on control and tyrosine supplemented food (Figure 2—figure supplement 1C) that may explain the differences in response to the high concentration of tyrosine in male and female flies (Figure 2—figure supplement 1A,B). Moreover, mRNA levels of other enzymes from the tyrosine degradation pathway, CG11796, Hgo, Faa, Hn, were increased in male flies, whereas the level of RP49 did not change (Figure 2—figure supplement 1D). Altogether, these data suggest that CG1461 is a functional ortholog of mammalian TAT that is required for the degradation of excess tyrosine to maintain stable levels of tyrosine on regular diet, and it is strongly induced when excess tyrosine is present. These data also point to the beneficial role of tyrosine in the regulation of lifespan in wild-type flies.
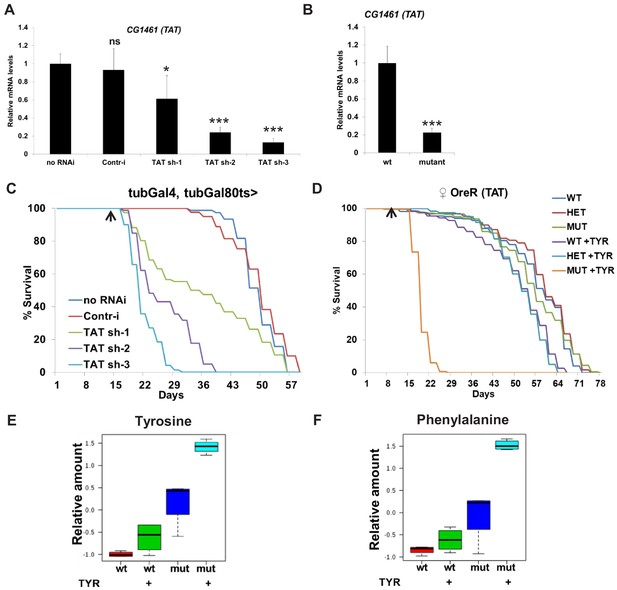
CG1461 functions as Tyrosine Aminotransferase/TAT and is necessary to degrade tyrosine.
(A) Relative mRNA levels of CG1461 in tubulin-Gal80ts, tubulin-Gal4 flies expressing either no RNAi, control RNAi or three different CG1461 RNAi for 10 days. Means ± SD. *p<0.05, ***p<0.001 (B) Relative mRNA levels of CG1461 in wild-type and CG1461-deficient flies (both backcrossed to wild-type OreR flies). Means ± SD. ***p<0.001 (C) Feeding adult flies with 5 g/L of tyrosine significantly suppresses lifespan of flies with ubiquitous adult-onset downregulation of CG1461. Arrow indicates the beginning of tyrosine feeding. p<0.001. (D) Feeding adult flies with 5 g/L of tyrosine significantly suppresses lifespan of CG1461 mutant but not wild-type or heterozygous flies. Arrow indicates the beginning of tyrosine feeding. p<0.001. Box plots of relative levels of tyrosine (E) and phenylalanine (F) in wild-type and CG1461-deficient flies fed either control or high level of tyrosine (5 g/L) diet.
The tyrosine degradation pathway regulates lifespan
Since supplementing tyrosine to wild-type flies increased their lifespan, levels of TAT and other enzymes in the tyrosine degradation pathway increased with age, and the levels of tyrosine were higher in the long-lived flies; we evaluated the effects of TAT and other enzymes in the tyrosine degradation pathway on lifespan using RNAi. To avoid developmental effects and differences in genetic backgrounds, we used the Actin Gene-Switch (Actin-GS) inducible Gal4/UAS expression system (Roman et al., 2001; Osterwalder et al., 2001), whereby UAS-RNAi expression is driven by Gal4 when flies are fed mifepristone (RU486). Expression of different control RNAi lines did not affect lifespan (Parkhitko et al., 2016), while two independent RNAi lines against TAT significantly extended lifespan (TAT RNAi-1 (weak), 9% increase in Mean Lifespan, p<0.0001, log-rank test; TAT RNAi-3 (strong), 17% increase in Mean Lifespan, p<0.0001, log-rank test) (Figure 3A,B), and better RNAi efficiency was consistent with more robust lifespan extension. While downregulation of TAT can result in lifespan extension due to a general inhibition of the tyrosine degradation pathway, it can also be due to a potential (although unknown) alternative function of TAT. To confirm that modulation of tyrosine degradation was responsible for lifespan extension, we downregulated two additional enzymes: CG11796 and Hgo. CG11796 is a 4-hydroxyphenylpyruvate dioxygenase that catalyzes the conversion of 4-hydroxyphenylpyruvate to homogentisate, the second step in the tyrosine degradation pathway (Figure 1E). Hgo is a homogentisate 1,2-dioxygenase that catalyzes the conversion of homogentisate to 4-maleylacetoacetate (Figure 1E). Downregulation of both CG11796 (Figure 3C) and Hgo (Figure 3D) moderately but significantly increased lifespan (CG11796 RNAi, 10% increase in Mean Lifespan, p<0.0001, log-rank test; Hgo RNAi, 12% increase in Mean Lifespan, p<0.0001, log-rank test).
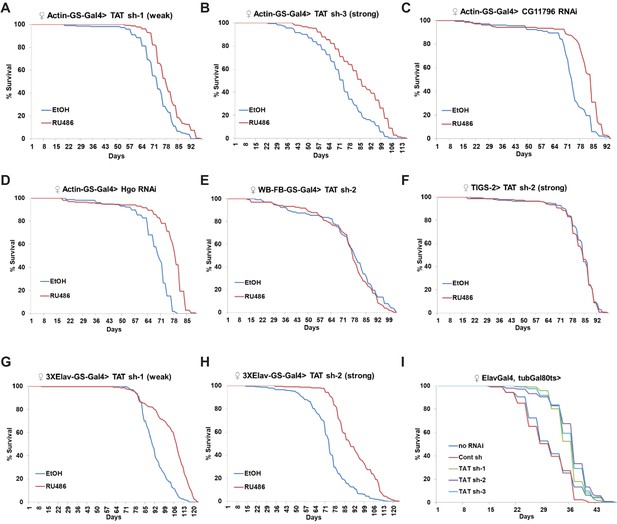
Whole-body and neuronal-specific downregulation of CG1461/Tyrosine Aminotransferase extends lifespan.
(A) Ubiquitous adult-onset expression of CG1461 RNAi-1 increases lifespan in females. p<0.0001. (B) Ubiquitous adult-onset expression of CG1461 RNAi-3 increases lifespan in females. p<0.0001. (C) Ubiquitous adult-onset expression of CG11796 RNAi increases lifespan in females. p<0.0001. (D) Ubiquitous adult-onset expression of Hgo RNAi increases lifespan in females. p<0.0001. (E) Fat body-specific adult-onset expression of CG1461 RNAi does not affect lifespan in females. (F) Intestine-specific adult-onset expression of CG1461 RNAi does not affect lifespan in females. (G) Neuronal-specific adult-onset expression of CG1461 RNAi-1 increases lifespan in females. p<0.0001. (H) Neuronal-specific adult-onset expression of CG1461 RNAi-2 increases lifespan in females. p<0.0001. (I) Neuronal-specific adult-onset expression of CG1461 RNAi-1, -2, and -3 increases lifespan in females. p<0.0001.
To further dissect the role of TAT in lifespan extension, we next tested whether specific tissues were responsible for lifespan extension. Expression of a strong TAT RNAi in the entire fat-body of adult flies starting at 1 week of age using Geneswitch driver strain WB-FB-GS, which contains both a head fat-body driver (S1-32) and a body-fat-body driver (S1-106) (Giannakou et al., 2007; Shen et al., 2009), did not affect lifespan (Figure 3E). Similarly, expression of strong TAT RNAi using the TIGS-2 Geneswitch driver (TIGS-2), which is associated with digestive tract-specific expression (Rera et al., 2011; Poirier et al., 2008), did not affect lifespan (Figure 3F). However, the 3XElav Geneswitch driver (3XElav-GS), which drives nervous-system-specific expression (Osterwalder et al., 2001; Shen et al., 2009), led to a significant extension of lifespan when two different TAT RNAi were expressed starting at 1 week (TAT RNAi-1 (weak), 12.6% increase in Mean Lifespan, p<0.0001, log-rank test; TAT RNAi-3 (strong), 24.5% increase in Mean Lifespan, p<0.0001, log-rank test) (Figure 3G,H). Similar results were obtained when TAT RNAi lines were expressed in the nervous system of adult flies using ElavGal4 and the temperature-sensitive tubulin-Gal80ts repressor. Flies were allowed to develop at 18°C and then switched to 29°C after eclosion to induce RNAi expression. Adult onset neuronal-specific TAT RNAi expression resulted in strong lifespan extension compared to no RNAi or control RNAi expression (TAT RNAi-1, 15% increase in Mean Lifespan, p<0.0001, log-rank test; TAT RNAi-2, 18% increase in Mean Lifespan, p<0.0001; TAT RNAi-3, 17% increase in Mean Lifespan, p<0.0001, log-rank test) (Figure 3I). Interestingly, nervous-system-specific TAT downregulation resulted in stronger lifespan extension than with a ubiquitous driver. While differences in genetic background or/and the strength of Gal4 induction by mifepristone could explain these effects, one possibility is that TAT downregulation can be both beneficial and detrimental depending on tissue and cell type. Altogether, our results suggest that ubiquitous and tissue-specific suppression of the tyrosine degradation pathway is sufficient to extend lifespan.
Ubiquitous downregulation of TAT causes metabolic reprogramming by affecting mitochondrial/antioxidant pro-longevity metabolic factors
To understand the mechanisms of lifespan extension by TAT downregulation, we performed metabolomic profiling of flies that expressed either control RNAi or two different strong TAT RNAi under the control of the ubiquitous temperature-sensitive (tubulin-Gal4, tubulin-Gal80ts) driver for 10 days. Principal component analysis (PCA) of the measured metabolites clearly distinguished flies with control and TAT RNAi but clustered the two independent TAT RNAi lines together (Figure 4A). We identified 24 metabolites that were significantly and commonly changed in flies expressing two different strong TAT RNAi compared to control flies (Figure 4B). As expected, one of the significantly altered metabolites was tyrosine (Figure 4B,C). Some of the other significantly changed metabolites have been previously connected to lifespan regulation. Normal aging and premature aging in mtDNA mutator mice exhibit increased brain lactate (Ross et al., 2010) and cerebrospinal fluid lactate is elevated in aging humans (Yesavage et al., 1982). Dietary supplementation with D-glucosamine-6-phosphate (GlcN-6-phosphate) extends lifespan of nematodes and aging mice acting as an inhibitor of glycolysis and promoting mitochondrial function (Weimer et al., 2014). Downregulation of TAT led to a significant increase of GlcN-6-phosphate (Figure 4D) and decrease in products of glycolysis - lactate (Figure 4E) and NADH (Figure 4F). Another lifespan-related metabolite that was significantly upregulated after downregulation of TAT was nicotinamide (Figure 4J). Nicotinamide supplementation improves healthspan in mice, reduces oxidative stress and inflammation (Mitchell et al., 2018). In flies, overexpression of Nicotinamide mononucleotide adenylyltransferase (Nmnat) and Nicotinamidase (Naam), which encode enzymes involved in conversion of nicotinamide to NAD promotes longevity, improves mitochondrial function and protects against oxidative stress (Balan et al., 2008; Liu et al., 2018). Moreover, downregulation of TAT led to a significant increase of methylcysteine (Figure 4G) and decrease of the oxidized form of methionine, methionine sulfoxide (target of the MSRA antioxidant system). Dietary supplementation with S-methyl-L-cysteine has been shown to enhance the MSRA antioxidant system in Drosophila and to delay the progression of the movement defect in flies overexpressing α-synuclein in the nervous system (Wassef et al., 2007). Strikingly, five of the significantly altered metabolites, NADH, thiamine pyrophosphate, NADP, glutathione and lactate, belong to the pyruvate metabolism (Figure 4B,E,F,I,K) and point to metabolic pathways associated with mitochondrial function. Pyruvate is ultimately destined for transport into mitochondria as a major fuel to drive ATP production by oxidative phosphorylation and feed into multiple biosynthetic pathways intersecting the TCA cycle. The major sources of pyruvate in the cytoplasm are phosphoenolpyruvate, alanine and lactate (Gray et al., 2014).
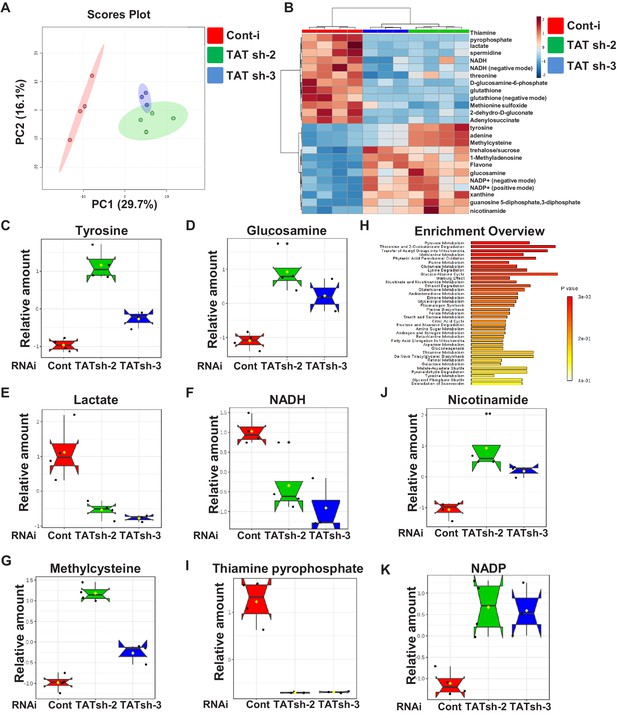
Downregulation of CG1461/Tyrosine Aminotransferase leads to reprogramming of metabolism related to mitochondrial function.
(A) Principal component analysis of tubulin-Gal4, tubulin-Gal80ts flies expressing either control RNAi or two different TAT RNAi. (B) Heat map showing the significantly and commonly changed metabolites in flies expressing two different TAT RNAi. Box plots of relative levels of tyrosine (C), lactate (D), glucosamine (E), nicotinamide (F), methylcysteine (G) in tubulin-Gal4,tubulin-Gal80ts flies expressing either control RNAi or two different TAT RNAi. (H) Metabolic Set Enrichment Analysis of the metabolites that changed significantly and commonly in flies expressing two different TAT RNAi. Box plots of relative levels of NADH (I), thiamine pyrophosphate (J), NADP (K) in tubulin-Gal4,tubulin-Gal80ts flies expressing either control RNAi or two different TAT RNAi.
We further tested whether neuronal-specific downregulation of TAT would cause metabolic alterations in whole flies similar to the whole-body downregulation of TAT. We performed metabolomics of flies that expressed either control RNAi or strong TAT RNAi (shRNA-2) under the control of the neuronal-specific temperature sensitive (elav-Gal4, tubulin-Gal80ts) driver for 10 days. Neuronal-specific downregulation of TAT caused the upregulation of tyrosine (Figure 3—figure supplement 1A) and metabolic reprogramming similar to the whole-body downregulation of TAT. We detected several metabolites (glucosamine, methylcysteine, and NADH) that were similarly altered as a result of whole-body-driven and neuronal-specific downregulation of TAT (Figure 3—figure supplement 1B–D). Altogether, our results suggest that downregulation of TAT causes global metabolic reprogramming involving metabolites belonging to mitochondrial metabolism and antioxidant defense that have known roles in the regulation of lifespan.
Whole-body downregulation of TAT elevates levels of tyrosine-derived neurotransmitters
Tyrosine is a precursor for biogenic amine neurotransmitters: dopamine, tyramine, and octopamine. Tyrosine can be hydroxylated by tyrosine hydroxylase (TH/ple) to produce DOPA, and DOPA can be decarboxylated by Dopa decarboxylase (Ddc) to produce dopamine. Alternatively, tyrosine can be decarboxylated by tyrosine decarboxylase (Tdc1/Tdc2) to produce tyramine. Tyramine can be further converted by tyramine-β-hydroxylase (TβH) to octopamine. Octopamine and tyramine are the invertebrate counterparts of the vertebrate adrenergic transmitters adrenaline and noradrenaline. We hypothesized that redirection of tyrosine from the production of neurotransmitters into the tyrosine degradation pathway could result in either decreased levels of neurotransmitters or aggravation of mitochondrial function via feeding of tyrosine into the TCA cycle. To test whether TAT is involved in the regulation of the levels of tyrosine-derived neurotransmitters, we measured levels of tyrosine, DOPA, Dopamine, Tyramine, and Octopamine in heads of TAT wild-type, heterozygous, and mutant middle-age flies when the levels of TAT and other enzymes in the tyrosine degradation pathway are significantly increased. Loss of TAT led to the increase of tyrosine (Figure 5A) and all tyrosine-derived neurotransmitters (Figure 5B,C,D,E), but did not affect the levels of Histamine or GABA (Figure 4—figure supplement 1A,B). We further tested whether neuronal-specific downregulation of TAT would also increase the levels of tyrosine-derived neurotransmitters. We measured levels of DOPA, Dopamine, Tyramine, and Octopamine in heads of flies that expressed either control RNAi or strong TAT RNAi under the control of the neuronal-specific temperature sensitive (elav-Gal4, tubulin-Gal80ts) driver for 7 days and that were maintained either on regular food or food containing 5 mM of tyrosine for 2 days before the analysis. Neuronal-specific downregulation of TAT in the combination with supplementation of tyrosine caused upregulation of DOPA and Octopamine (Figure 4—figure supplement 1C,D). We have not detected significant increase in the levels of either tyramine or dopamine (Figure 4—figure supplement 1E,F), potentially, due to compensatory degradation of tyrosine in non-neuronal tissues or insufficient timing of TAT downregulation.
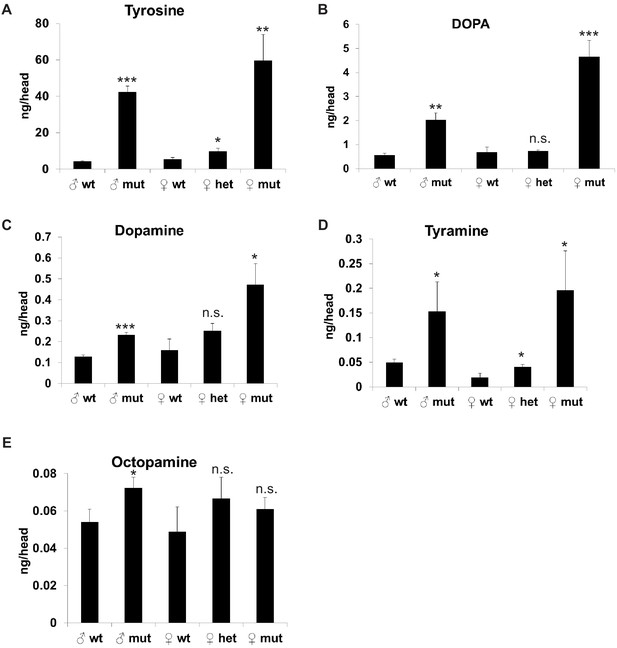
Whole-body downregulation of Tyrosine Aminotransferase/TAT elevates levels of tyrosine-derived neurotransmitters in fly heads.
Head levels of Tyrosine (A), DOPA (B), Dopamine (C), Tyramine (D), and Octopamine (E) in CG1461/TAT wild-type (wt), heterozygous (het), and mutant (mut) flies. Means ± SD. *p<0.05, **p<0.01, ***p<0.001.
We then tested whether supplementation of neurotransmitters via feeding can prolong lifespan. It has been previously demonstrated that octopamine-deficient Tβh-null flies are sterile because they retain fully developed eggs, a defect that can be rescued by transferring flies onto octopamine- or norepinephrine- supplemented food (Monastirioti et al., 1996). Also, intermittent octopamine feeding to adult flies can substitute for exercise in sedentary flies, providing a number of pro-healthspan benefits (Sujkowski et al., 2017). Similarly, behavioral defect (sensitization to cocaine) in iav mutant flies that have significantly reduced levels of tyramine due to reduced activity of the enzyme tyrosine decarboxylase can be rescued by supplementing the food with tyramine (McClung and Hirsh, 1999). In addition, L-DOPA feeding rescues disrupted behaviors in neural dopamine-deficient flies (Riemensperger et al., 2011). To test whether age-dependent decrease in the levels of neurotransmitters are related to aging, we fed wild-type OreR flies with 5 mM of tyramine, octopamine, and L-DOPA starting at week 2 of age (choosing the concentration that was able to rescue genetic defects associated with loss of these neurotransmitters). Although this supplementation marginally (but statistically significantly) increased Drosophila lifespan (Figure 4—figure supplement 1G,H), the effect was weaker as compared to the lifespan extension observed following neuronal TAT downregulation, suggesting potential involvement of additional mechanisms of lifespan extension by downregulation of TAT.
Inhibition of the electron transport chain upregulates expression of components of the tyrosine degradation pathway that can be rescued by Tigecycline
While most cells use glucose/pyruvate/lactate for ATP synthesis, changes in cellular homeostasis can lead to a switch in fuel utilization that results in the oxidation of fatty acids and amino acids to produce NADH and FADH2 to feed the mitochondrial electron transport chain (ETC) (Area-Gomez et al., 2019). Tyrosine can be degraded via the tyrosine degradation pathway and generate two fragments, each of which can enter the TCA cycle. Four of the nine carbon atoms of tyrosine generate free acetoacetate, which is converted into acetoacetyl-CoA, and the second four-carbon fragment is recovered as fumarate. Eight of the nine carbon atoms of these two amino acids thus enter the citric acid cycle and the remaining carbon is lost as CO2. We hypothesized that aging and neurodegeneration can serve as a signal for the switch in tyrosine metabolism from production of neurotransmitters into the tyrosine degradation pathway and further aggravate mitochondrial dysfunction. To test our hypothesis, we expressed RNAi against different components of ETC (CG9762 – Complex I, SDHC – Complex II, CG18809 – Complex IV, and ms [Hoffman et al., 2014] 72Dt – Complex V) in young flies under the control of a ubiquitous temperature-sensitive driver (tubulin-Gal4, tubulin-Gal80ts) for 10 days. Suppression of all components of ETC resulted in a profound increase of mRNA levels of TAT (between 1.5- and two fold induction) with the greatest effect seen with downregulation of CG9762 (Complex I) (Figure 6A). We tested eight additional different subunits of complex I (CG9172, NP15.6, CG8680, CG1970, CG3214, mtacp1). Downregulation of each of them resulted in a similar increase in mRNA levels of TAT (up to fivefold induction) (Figure 6A). We then tested whether other enzymes in the tyrosine degradation pathway respond to the suppression of complex I of ETC. Similar to TAT, downregulation of different subunits of complex I caused a strong increase in mRNA levels of faa, Hgo, and CG11796, enzymes that act downstream of TAT in the tyrosine degradation pathway (Figure 5—figure supplement 1A). We also tested whether neuronal-specific suppression of complex I of ETC via downregulation of NP15.6 would phenocopy the effect of the whole body downregulation of NP15.6. We measured levels of TAT and other enzymes in the tyrosine degradation pathway in whole flies that expressed either control RNAi or strong TAT RNAi under the control of the neuronal-specific temperature-sensitive (elav-Gal4, tubulin-Gal80ts) driver for 10 days. Suppression of complex I of ETC only in neuronal cells was not enough to increase the expression of TAT or other enzymes in the tyrosine degradation pathway (Figure 5—figure supplement 1B). Interestingly, downregulation of components of complex I ETC can either extend or suppress the Drosophila lifespan depending on the strength and/or duration of this suppression (Copeland et al., 2009; Foriel et al., 2019; Rea et al., 2007). We further tested whether supplementation of tyrosine to flies with the whole-body downregulation of a component of Complex I ETC - NP15.6 would affect their lifespan. Expression of NP15.6 RNAi under the control of the ubiquitous temperature sensitive (tubulin-Gal4, tubulin-Gal80ts) driver caused significant reduction of lifespan with a stronger effect in female flies (−13% in male flies, p<0.0001, log-rank test; −33% in female flies, p<0.0001, log-rank test) (Figure 5—figure supplement 1C,D). Supplementation of different concentrations of tyrosine partially rescued lifespan in both male (+8.7%, + 11%, +11% for 1X, 2.5X, and 5X concentrations of tyrosine, p<0.0001, log-rank test) (Figure 5—figure supplement 1C) and female flies (+9.5%, + 10%, +10.5% for 1X, 2.5X, 5X concentrations of tyrosine, p<0.0001, p=0.0016, p=0.0002, log-rank test) (Figure 5—figure supplement 1D). In summary, inhibition of mETC Complex I function can decrease lifespan, which can be partially rescued by tyrosine supplementation.
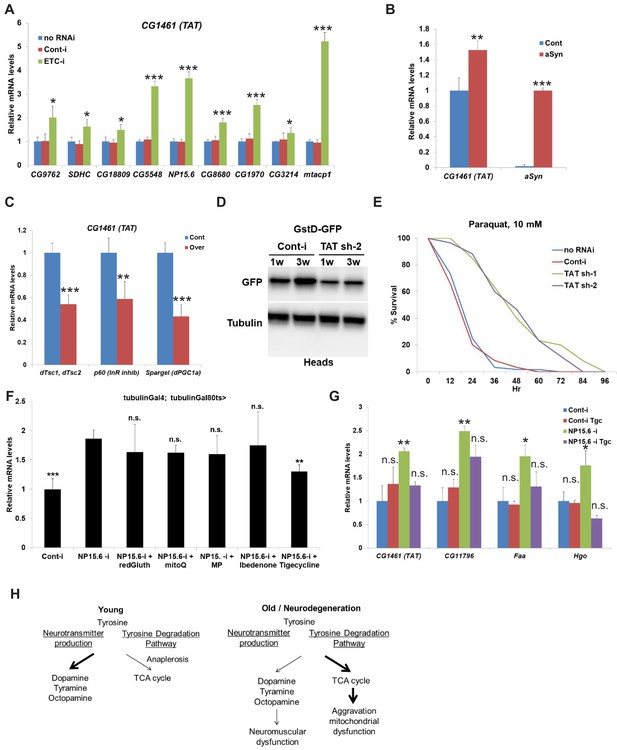
Mitochondrial dysfunction/neurodegeneration upregulates the level of CG1461/tyrosine aminotransferase.
(A) Relative mRNA levels of CG1461/TAT in Gal80ts; tubulin-Gal4 flies expressing either no RNAi, control RNAi or RNAi against different subunits of mitochondrial ETC – CG9762, SDHC, CG18809, CG5548, NP15.6, CG8680, CG1970, CG3214, mtacp1 for 10 days. Means ± SD. (B) Relative mRNA levels of CG1461/TAT and α -synuclein in heads of flies with or without expression of wild-type human α-synuclein. Means ± SD. (C) Relative mRNA levels of CG1461/TAT in tubulin-Gal80ts, tubulin-Gal4 flies overexpressing control or p60 (inhibitory subunit of InR), dTsc1/dTsc2 (TSC complex, dTOR inhibitor) or PGC1a/Spargel. Means ± SD. (D) Immunoblot analysis of GFP and tubulin in heads of 1-week and 3-week-old flies expressing either control or TAT RNAi under pan-neuronal driver (ElavGal4) in the presence of GstD-GFP. (E) Ubiquitous adult-onset downregulation of TAT prolongs lifespan under oxidative stress (10 mM Paraquat). (F) Relative mRNA levels of CG1461/TAT in tubulin-Gal80ts, tubulin-Gal4 flies expressing either control or NP15.6 RNAi and fed with 10 mM reduced Glutathione, 100 µM mitoQ, 10 mM methyl pyruvate, 100 µM Ibedenone, or 100 µM Tigecycline. Means ± SD (G) Relative mRNA levels of CG1461/TAT, CG11796, faa, and Hgo in tubulin-Gal80ts, tubulin-Gal4 flies expressing either control or NP15.6 RNAi and fed with either control or 100 µM Tigecycline. (H) Working model. *p<0.05, **p<0.01, ***p<0.001.
There is extensive evidence for the involvement of mitochondrial dysfunction in the pathogenesis of neurodegenerative diseases such as Alzheimer’s disease (AD) and Parkinson’s disease (PD) (Area-Gomez et al., 2019). Thus, we further tested whether pathological conditions associated with mitochondrial dysfunction would also cause the upregulation of TAT. To investigate whether expression of α-synuclein previously shown to promote mislocalization of the mitochondrial fission protein Drp1 leading to mitochondrial dysfunction and neuronal death also induced expression of TAT, we used a Drosophila α-synucleinopathy model (Ordonez et al., 2018). As expected and similar to ETC complex I inhibition, expression of wild-type human α-synuclein using the Syb-QF2 panneuronal driver resulted in the increase of TAT mRNA levels (Figure 6B). We further tested whether downregulation of TAT would rescue the neuronal loss associated with α-synuclein expression. We downregulated TAT using three different RNAi lines in the presence of α-synuclein expression. As expected, α-synuclein expression caused strong neurodegeneration and dramatic neuronal loss; however, downregulation of TAT with three different RNAi lines did not suppress this phenotype (Figure 5—figure supplement 1E,F). PD is characterized by extensive reprogramming of metabolism and targeting of multiple metabolic pathways may be required to prevent neurodegeneration (Shao and Le, 2019).
To test whether stimulation of mitophagy/mitochondrial biogenesis decreases the level of TAT in young flies, we tested how overexpression of Spargel (Drosophila orthologue of PGC1α), p60 (inhibitory subunit of InR), or dTsc1/dTsc2 (TSC complex, dTOR inhibitor) affects the level of TAT. Both overexpression of Spargel (Rera et al., 2011) and suppression of InR (Clancy et al., 2001) or TOR (Kapahi et al., 2004) signaling are critical regulators of mitophagy/mitochondrial biogenesis and extend Drosophila lifespan (Figure 6—figure supplement 1A). Overexpression of Spargel, p60, and dTsc1/dTsc2 significantly suppressed expression of TAT (Figure 6C). To test how mechanistically downregulation of TAT extends lifespan, we crossed flies expressing either control RNAi or TAT RNAi with a pan-neuronal driver (ElavGal4) to a number of GFP reporter lines relevant to different age-dependent processes—GstD-GFP (oxidative stress) (Sykiotis and Bohmann, 2008), GFP-CL1 (proteosomal activity) (Pandey et al., 2007), hsp22-GFP (mitochondrial stress) (Yang and Tower, 2009), STAT92E-GFP (Stat pathway) (Bach et al., 2007), and Drs-GFP (antimicrobial response) (Ferrandon et al., 1998)—and tested their activity in fly heads. We found that the amount of GFP under the control of the GstD promoter increased with age in fly brains, and this increase was suppressed by downregulation of TAT (Figure 6D),while we have not detected differences between control and TAT RNAi with other reporters, at least at the tested conditions (Figure 6—figure supplement 1B–E). This suggests that downregulation of TAT may regulate lifespan at least partially via counteracting the accumulation of oxidative stress in neuronal tissue. We further found that downregulation of TAT strongly increased resistance to 10 mM paraquat (redox cycler which causes oxidative damage) (Figure 6E). Downregulation of ETC complex I can have multiple effects on mitochondria leading to increased mitoROS production, mtUPR, decreased NAD+/NADH ratio, decreased ATP production and other effects (Rhooms et al., 2020). To dissect a potential mechanism of how downregulation of ETC Complex I and potentially aging cause upregulation of TAT, we fed flies with an available toolbox of chemical inhibitors: mitoQ dye (quencher of mitochondrial ROS), methyl pyruvate (membrane permeable pyruvate), idebenone (a bypass of mitochondrial complex I), Tigecycline (antibiotic that can inhibit mitochondrial translation), and reduced glutathione (antioxidant) (Wisnovsky et al., 2016). Only treatment with Tigecycline suppressed upregulation of TAT induced by inhibition of ETC Complex I (Figure 6F), although we cannot rule out that other chemicals did not rescue TAT upregulation because of technical limitations associated with feeding flies with chemicals (poor distribution in fly body, wrong concentration, instability in fly food, etc.). The effect observed for Tigecycline suggests that downregulation of ETC Complex I and potentially aging causes an integrated stress response that can be rescued by inhibition of mitochondrial protein translation. Moreover, treatment with Tigecycline also suppressed other enzymes in the tyrosine degradation pathway (CG11796, Hgo, Faa) that were upregulated by the inhibition of ETC Complex I but did not affect their levels in control flies (Figure 6G). Interestingly, although inhibition of mitochondrial translation would be expected to cause mitochondrial stress, at this concentration/timing, we have not observed upregulation of markers of mitochondrial stress (ClpX, hsp10, hsp60, or hsp22) in both control flies and in flies with inhibition of ETC Complex I (Figure 6—figure supplement 1F). Altogether, our results suggest that, similar to aging, mitochondrial dysfunction serves as a signal to elevate expression of TAT and other enzymes in the tyrosine degradation pathway and that this effect can be rescued by the FDA-approved drug Tigecycline.
Discussion
By comparing metabolic changes in control and long-lived flies during aging, we identified the amino acid tyrosine and the tyrosine degradation pathway as potential targets for lifespan extension. Supplementing flies with tyrosine or whole-body and tissue-specific downregulation of tyrosine aminotransferase/TAT, the first and rate-limiting enzyme in the tyrosine degradation pathway, significantly extended lifespan. Moreover, upregulation of TAT might serve as a switch between neurotransmitter production and anaplerosis under mitochondrial dysfunction and aging. In addition, we found that the FDA-approved drug Tigecycline can suppress the upregulation of enzymes in the tyrosine degradation pathway under the conditions of mitochondrial dysfunction.
Age-dependent metabolic reprogramming
We searched for changes in the fly metabolome caused by aging in control and long-lived flies and hypothesized that preventing some of these changes would increase lifespan and prevent age-dependent health deterioration. Previously, we found striking differences in multiple methionine metabolism intermediates between control and long-lived flies, including S-adenosylhomocysteine and homocysteine, and showed that modulating levels of these metabolites extended lifespan (Parkhitko et al., 2016). Here, we extended our analysis and identified 49 metabolites that changed significantly with age between control and long-lived flies. Some of these metabolites represent metabolic pathways that were previously implicated in lifespan extension and the role of others is unknown. Systematic interrogation of the enzymes regulating these metabolites should lead to identification of previously unknown regulators of lifespan in Drosophila.
Amino acid metabolism and lifespan extension
Amino acids play a key role in lifespan extension by calorie/dietary restriction. In Drosophila, restricting dietary protein extends lifespan, whereas carbohydrate and lipid restriction have little effect on survival (Mair et al., 2005). In addition, manipulation of metabolism of specific amino acids can extend lifespan in flies and other organisms. For example, methionine restriction (Lee et al., 2014) or activation of the methionine flux (Parkhitko et al., 2016; Parkhitko et al., 2019) prolongs health- and lifespan in flies and other species, whereas the mechanisms of lifespan extension do not overlap. This effect could be due to the processing of harmful metabolites, as activation of the methionine flux would promote processing of SAH, which accumulates with age and inhibits methyltransferases (Parkhitko et al., 2019). Impairing threonine catabolism by downregulation of glycine-C-acetyltransferase promotes the lifespan of C. elegans via stimulation of methylglyoxal formation (Ravichandran et al., 2018). Methylglyoxal is a reactive dicarbonyl inducing oxidative stress and while its high concentration is harmful, low concentration stimulates stress-responsive pathways and hormetic activity on lifespan in C. elegans (Ravichandran et al., 2018). Increased homocysteine processing via the transsulfuration pathway (Kabil et al., 2011) and glycine supplementation extend Drosophila and C. elegans lifespan, respectively, partially via stimulating the flux through methionine metabolism. Here, we identified a new branch of amino acid metabolism, namely the tyrosine degradation pathway that can regulate lifespan. In agreement with our data showing that levels of TAT are decreased in flies with overexpression of dTsc1/Tsc2, Yuan et al. found that the level of hpd-1, the worm orthologue of 4-hydroxyphenylpyruvate dioxygenase/CG11796, is decreased in long-lived eat-2 mutant worms (genetic model of calorie restriction in worms). Moreover, downregulation of hpd-1 increased worm lifespan, which is consistent with increased Drosophila lifespan observed with downregulation of CG11796 (Yuan et al., 2012). In addition, the C. elegans ortholog of TAT, tatn-1, influences insulin signaling, development, and lifespan via modulation of aak-2/AMPK signaling (Ferguson et al., 2013). Also, in worms, AMPK/calcineurin-mediated longevity was regulated cell-nonautonomously via regulation of octopamine (Burkewitz et al., 2015). Altogether, we propose two potential mechanisms of lifespan extension: preservation of the production of neurotransmitters and prevention of increased tyrosine feeding into the TCA cycle (Figure 6H).
Amino acid catabolism and mitochondria
Aging is characterized by progressive accumulation of dysfunctional mitochondria and a reduced capacity to produce ATP. Mitochondrial ETC generates a proton gradient derived from NADH and FADH2 that are produced in the TCA cycle, and ATP synthase uses this gradient for the production of ATP from ADP. Changes in cellular homeostasis can result in the oxidation of amino acids to produce NADH and FADH2 to feed the mitochondrial electron transport chain (ETC) (Area-Gomez et al., 2019). Due to the fact that: (a) metabolism of different amino acids has recently been shown to play an important role in the regulation of aging; (b) mitochondrial dysfunction is evident in aging and neurodegeneration; (c) tyrosine can play anaplerotic role via the tyrosine degradation pathway and production of acetoacetyl CoA and fumarate; and (d) enzyme levels in the tyrosine degradation pathway increase with age, we hypothesize that, at least in flies, aging drives upregulation of tyrosine catabolism and redirects tyrosine from neurotransmitter production into the tyrosine degradation pathway to compensate for age-driven mitochondrial dysfunction (Figure 6H). Moreover, we demonstrate that these changes can be phenocopied by the inhibition of mETC with the strongest effect seen with inhibition of mETC complex I. Although it is unknown how the activity of the mETC complex I is changed with age in neuronal cells in flies, complex I activity declines with age in both rodents and human in different organs including brain (Gómez and Hagen, 2012). Moreover, overexpression of NDI1, an alternative NADH dehydrogenase that can bypass complex I, prolonged Drosophila lifespan when it was either expressed in whole flies or only in neuronal cells (Sanz et al., 2010; Bahadorani et al., 2010), raising the question of how downregulation of mETC complex I extends lifespan while it can also phenocopy aging and reduce lifespan. It has been shown in worms that lifespan extension caused by inhibition of ETC is limited by the narrow window of timing of treatment and strength of knockdown (Rea et al., 2007). Similarly in flies, lifespan extension by inhibition of mETC was achieved with the da-GeneSwitch driver, which is weaker and mosaic compared with non-inducible Gal4 drivers (Copeland et al., 2009), whereas usage of stronger and ubiquitous drivers caused severe lifespan shortening (Foriel et al., 2019). It is still an open question why inhibition of mETC is beneficial in one context and detrimental in another.
Tyrosine aminotransferase and tyrosinemia
Tyrosinemia type II, also known as Richner-Hanhart Syndrome (OMIM # 276600), is a rare autosomal recessive disorder, caused by mutations in the gene encoding tyrosine aminotransferase and is manifested by eye, skin, and central nervous system alterations (Scott, 2006). Why would downregulation of TAT extend lifespan in flies but cause disease in humans? The degree of downregulation, duration, and tissue specificity could be a key for the beneficial effects of TAT downregulation. Indeed, we observed stronger lifespan extension with nervous-specific TAT downregulation than with ubiquitous ActinGeneSwitch driver. In addition, TAT-mutant flies had shortened lifespan. Moreover, the ubiquitous ActinGeneSwitch driver has a mosaic pattern of expression that would probably still allow degradation of some tyrosine and create a balance between neurotransmitter production and tyrosine degradation. This notion is consistent with the fact that patients heterozygous for TAT do not exhibit any clinical manifestations. In agreement with the beneficial role of downregulation of TAT on lifespan, our metabolomics analysis revealed upregulation of several metabolites previously connected to lifespan extension. These metabolites include GlcN-6-phosphate (Weimer et al., 2014), nicotinamide (Balan et al., 2008; Liu et al., 2018), and methylcysteine (Wassef et al., 2007). Targeting metabolic pathways attributed to these metabolites extend health- and lifespan across different species (Parkhitko et al., 2020). It will be of interest to elucidate the mechanisms leading to the elevation of these metabolites. NTBC/nitisinone/Orfadin (2-(2-nitro-4-trifluoromethylbenzoyl)cyclohexane-1,3-dione) is an FDA-approved drug that inhibits 4-hydroxyphenylpyruvate dioxygenase in the tyrosine degradation pathway and is currently approved for the treatment of hereditary tyrosinemia type 1 (Lock et al., 2014). Given that our current study shows that inhibition of the tyrosine degradation pathway and specifically of CG11796, the fly ortholog of 4-hydroxyphenylpyruvate dioxygenase, extends lifespan, it would be of interest to test the potential of NTBC/nitisinone/Orfadin to promote health- and lifespan in humans.
Tyrosine supplementation in human clinical trials
Tyrosine is a precursor for biogenic amine neurotransmitters: dopamine, tyramine, and octopamine. Tyramine and octopamine in flies are analogous to epinephrine (adrenaline) and norepinephrine (noradrenaline) in humans. In mice, tyrosine supplementation reaches maximum concentration in the brain 1 hr after oral ingestion (Topall and Laborit, 1989) and enhances catecholamine synthesis in particular noradrenergic neurons (Gibson and Wurtman, 1978; Milner and Wurtman, 1986). In rats, tyrosine supplementation increases dopamine concentration in the extracellular fluid of corpus striatum and nucleus accumbens (During et al., 1988; Woods and Meyer, 1991). Due to the beneficial role of catecholamines in coping with stress, a number of clinical trials in healthy subjects have been designed to investigate whether tyrosine reduces cognitive and physiological stress in humans exposed to a combination of mental and physical stressors. In humans, tyrosine has been shown to improve cold-induced decrements in working memory (Shurtleff et al., 1994), improve subject performance on stress-sensitive attention tasks (Deijen and Orlebeke, 1994), improve attentional focus in the presence of a distractor (Deijen and Orlebeke, 1994), and attenuate performance decrements and adverse mood states associated with acute exposure to cold and hypoxia (Deijen and Orlebeke, 1994; Banderet and Lieberman, 1989; Dollins et al., 1995; Shukitt-Hale et al., 1996). Most of these clinical trials in healthy subjects have been performed on young volunteers. Interestingly, the first study investigating the effects of tyrosine on cognitive effects in older adults has demonstrated unfavorable effects of higher doses tyrosine on working memory performance (van de Rest et al., 2017). These findings are consistent with our hypothesis that tyrosine metabolism is reprogrammed in older adults and that at this stage tyrosine supplementation enhances the tyrosine degradation pathway instead of enhancing the production of neurotransmitters. Finally, it would be interesting to test whether Tigecycline, an FDA-approved drug that suppresses the activity of the tyrosine degradation pathway in flies in response to mitochondrial dysfunction could restore normal levels of tyrosine-derived neurotransmitters in elderly people.
Materials and methods
Reagent type (species) or resource | Designation | Source or reference | Identifiers | Additional information |
---|---|---|---|---|
Antibody | Anti-α-Tubulin | Sigma | T5168 | |
Antibody | Anti-GFP | Invitrogen | A-6455 | |
Strain, strain background (Escherichia coli) | One Shot TOP10 Chemically Competent E. coli | Thermo Fisher Scientific | C404003 | |
Commercial assay or kit | iScript Reverse Transcription Supermix | Bio-Rad | 1708896 | |
Commercial assay or kit | iQ SYBR Green Supermix | Bio-Rad | 1708880 | |
Commercial assay or kit | BenchMark Prestained Protein Ladder | Invitrogen | 10748–010 | |
Chemical compound, drug | TRIzol reagent | Invitrogen | 15596–018 | |
Chemical compound, drug | Mifepristone | Cayman Chemical Company | 10006317 | |
Chemical compound, drug | Methyl viologen dichloride hydrate (Paraquat) | Sigma-Aldrich | 856177 | |
Chemical compound, drug | ProSieve EX transfer buffer | Lonza | 00200309 | |
Chemical compound, drug | ProSieve EX running buffer | Lonza | 200307 | |
Chemical compound, drug | L-Glutathione reduced | Sigma-Aldrich | G6013-5G | |
Chemical compound, drug | Laemmli Sample Buffer | Bio-Rad | 1610737 | |
Chemical compound, drug | RIPA | Cell Signaling | 9806 | |
Chemical compound, drug | Protease Inhibitor Cocktail Tablets | Roche | 4693159001 | |
Chemical compound, drug | Idebenone | Cayman Chemical Company | 15475 | |
Chemical compound, drug | Mitoquinol | Cayman Chemical Company | 89950 | |
Chemical compound, drug | Nuclease-Free Water (not DEPC-Treated) | Ambion, Inc | AM9930 | |
Chemical compound, drug | 3,4-Dihydroxy-L-phenylalanine | Millipore Sigma | D9628 | |
Chemical compound, drug | Octopamine hydrochloride | Millipore Sigma | O0250 | |
Chemical compound, drug | RQ1 RNase-Free DNase | Promega | M6101 | |
Chemical compound, drug | Tyramine | Sigma-Aldrich | T90344 | |
Commercial assay or kit | 4–20% Mini-PROTEAN TGX Precast Protein Gels | Bio-Rad | 4561095 | |
Commercial assay or kit | pENTR/D-TOPO Cloning Kit | Life Technologies | K2400-20 | |
Commercial assay or kit | Gateway LR Clonase II Enzyme mix | Invitrogen | 11791–020 | |
Genetic reagent (D. melanogaster) | white RNAi (HMS00017) | Bloomington Drosophila Stock Center | # 33623 | |
Genetic reagent (D. melanogaster) | GFP RNAi (HMS00314) | Perrimon’s lab | ||
Genetic reagent (D. melanogaster) | CG1461 RNAi (HMC03212) | Bloomington Drosophila Stock Center | # 51470 | |
Genetic reagent (D. melanogaster) | CG1461 RNAi (HMS05690) | Bloomington Drosophila Stock Center | # 67830 | |
Genetic reagent (D. melanogaster) | CG1461 RNAi (HMS05877) | Bloomington Drosophila Stock Center | # 76065 | |
Genetic reagent (D. melanogaster) | Hgo RNAi (HMC03775) | Bloomington Drosophila Stock Center | # 55629 | |
Genetic reagent (D. melanogaster) | CG11796 RNAi (HMC03663) | Bloomington Drosophila Stock Center | # 52923 | |
Genetic reagent (D. melanogaster) | CG9762 RNAi (HMC06415) | Bloomington Drosophila Stock Center | # 67311 | |
Genetic reagent (D. melanogaster) | Sdhc RNAi (HMC03497) | Bloomington Drosophila Stock Center | # 53281 | |
Genetic reagent (D. melanogaster) | CG18809 RNAi (HMS04326) | Bloomington Drosophila Stock Center | # 56907 | |
Genetic reagent (D. melanogaster) | CG5548 RNAi (HM05255) | Bloomington Drosophila Stock Center | # 30511 | |
Genetic reagent (D. melanogaster) | NP15.6 RNAi (HMS01560) | Bloomington Drosophila Stock Center | # 36672 | |
Genetic reagent (D. melanogaster) | CG8680 RNAi (HMC03434) | Bloomington Drosophila Stock Center | # 51860 | |
Genetic reagent (D. melanogaster) | CG1970 RNAi (HMC04814) | Bloomington Drosophila Stock Center | # 57499 | |
Genetic reagent (D. melanogaster) | CG3214 RNAi (HMS01584) | Bloomington Drosophila Stock Center | # 36695 | |
Genetic reagent (D. melanogaster) | mtacp1 RNAi (HM05206) | Bloomington Drosophila Stock Center | # 29528 | |
Genetic reagent (D. melanogaster) | B3 | Gift from Dr. Trudy Mackay | ||
Genetic reagent (D. melanogaster) | O1 | Gift from Dr. Trudy Mackay | ||
Genetic reagent (D. melanogaster) | O3 | Gift from Dr. Trudy Mackay | ||
Genetic reagent (D. melanogaster) | OregonR | Perrimon’s lab | ||
Genetic reagent (D. melanogaster) | tubulinGal4; tubulinGal80ts | Perrimon’s lab | ||
Genetic reagent (D. melanogaster) | CG1461-mutant | This paper | ||
Genetic reagent (D. melanogaster) | Actin-GeneSwitch-Gal4 | Gift from Dr. John Tower | ||
Genetic reagent (D. melanogaster) | Whole body fat body – GeneSwitch – Gal4 | Gift from Dr. John Tower | ||
Genetic reagent (D. melanogaster) | TIGS-2 | Gift from Dr. John Tower | ||
Genetic reagent (D. melanogaster) | 3X Elav-GeneSwitch-Gal4 | Gift from Dr. Scott Pletcher | ||
Genetic reagent (D. melanogaster) | ElavGal4; tubulinGal80ts | Perrimon’s lab | ||
Genetic reagent (D. melanogaster) | UAS-dTsc1,Tsc2 | Tapon et al., 2002 | ||
Genetic reagent (D. melanogaster) | UAS-p60 | Bloomington Drosophila Stock Center | # 25899 | |
Genetic reagent (D. melanogaster) | UAS-Spargel | Gift from Dr. David Walker | ||
Genetic reagent (D. melanogaster) | GstD-GFP | Gift from Dr. Dirk Bohmann | ||
Genetic reagent (D. melanogaster) | CG1461-GFP-tagged | VDRC stock center | # 318640 | |
Genetic reagent (D. melanogaster) | GFP-CL1 | Gift from Dr. Udai Pandey | ||
Genetic reagent (D. melanogaster) | hsp22-GFP | Gift from Dr. John Tower | ||
Genetic reagent (D. melanogaster) | STAT92-GFP | Bloomington Drosophila Stock Center | # 26198 | |
Genetic reagent (D. melanogaster) | Drs-GFP | Bloomington Drosophila Stock Center | # 55707 | |
Genetic reagent (D. melanogaster) | Xbp1-GFP | Bloomington Drosophila Stock Center | # 60730 | |
Genetic reagent (D. melanogaster) | Syb-QF2; QUAS- α-synuclein | Gift from Dr. Mel Feany |
Lifespan analysis
Request a detailed protocolFor survival analysis, flies were collected within 24 hr from eclosion, sorted by sex under light CO2 anesthesia, and reared at standard density (20–25 flies per vial) on cornmeal/soy flour/yeast fly food at 25°C and 60% humidity with 12 hr on/off light cycle. Flies were transferred to fresh vials every 2 days and dead flies counted. RU486 dissolved in ethanol was administered in the media at the final concentration of 150 ug/mL. The following RNAi lines were used: white RNAi (HMS00017); GFP RNAi (HMS00314); CG1461/TAT/tyrosine aminotransferase RNAi [TAT-1 HMC03212 (weak), TAT-2 HMS05690 (strong), TAT-3 HMS05877 (strong)], Hgo RNAi (HMC03775), CG11796 RNAi (HMC03663), CG9762 RNAi (HMC06415), Sdhc RNAi (HMC03497), CG18809 RNAi (HMS04326), CG5548 RNAi (HM05255), NP15.6 RNAi (HMS01560), CG8680 RNAi (HMC03434), CG1970 RNAi (HMC04814), CG3214 RNAi (HMS01584), mtacp1 RNAi (HM05206). All lifespan counts are listed in the Supplementary file 1.
qRT-PCR
Request a detailed protocolTotal RNA was extracted with the TRIzol reagent (Life Technologies), followed by DNase digestion using RQ1 RNase-Free DNase (Promega). Total RNA was reverse transcribed with the iScript cDNA synthesis kit (Bio-Rad). qRT-PCR was performed with the iQ SYBR Green Supermix (Bio-Rad) and a CFX96 Real- Time PCR Detection System (Bio-Rad). RpL32 and alpha-Tubulin 84B were used as a normalization reference. Relative quantitation of mRNA levels was determined with the comparative CT method.
Gene | FlyPrimerBank ID | Forward primer | Reverse primer |
---|---|---|---|
TAT | PP16388 | CGCTGTCCATTGGTGATCCC | TGGCATACCCATTGTACTTGC |
TAT | PP28777 | GGCTCCAAGCTATCCCTTAACA | CACCAATGGACAGCGGTATCA |
Faa | PP37488 | GGGATGTGGTAAGAAGCCAGA | CTGTGGCACAATGGAAACCG |
Hgo | PP36016 | CTTCCATTCCAGCCCTTCAAG | TTTCCATCCTTTGGAGGCAAG |
CG11796 | PP24189 | GGATTGCCCTCCACCAAGC | CAGGATTCTCTCGTACCAGGA |
GstZ2 | PP18005 | CCGCGAGGTGAATCCAATG | CTGGGGACGTGTTTCCTCC |
Hn | PP19433 | TGTTTTCGCCCAAGGATTCGT | CACCAGGTTTATGTCATGCTTCT |
aSynuclein | AAGAGGGTGTTCTCTATGTAGGC | GCTCCTCCAACATTTGTCACTT | |
Rp49 | ATCGGTTACGGATCGAACAA | GACAATCTCCTTGCGCTTCT |
Antibodies and immunoblot analyses
A rabbit anti-GFP antibody was obtained from Abcam, and the anti-tubulin antibody from Sigma-Aldrich. For immunoblot analyses, 10 flies or 20 heads were grinded in bead beader in RIPA (Cell Signaling) lysis buffer with phosphatase and protease inhibitors (Roche). Whole-cell lysates were resolved by electrophoresis, and proteins were transferred onto PVDF membranes (Immobilon P; Millipore), blocked in Tris-buffered saline Tween-20 buffer (Cell Signaling Technology) containing 2.5% dry milk, and probed with the indicated antibodies diluted in this buffer.
Statistical analysis
Request a detailed protocolStatistical analyses were performed in either JMP (SAS, Cary, NC, USA) or Excel.
Metabolite profiling
Request a detailed protocolTen to 20 flies per sample (four biological replicates) were collected and intracellular metabolites extracted using 80% (v/v) aqueous methanol. A 5500 QTRAP hybrid triple quadrupole mass spectrometer (AB/SCIEX) coupled to a Prominence UFLC HPLC system (Shimadzu) was used for steady-state analyses of the samples. Selected reaction monitoring (SRM) of 287 polar metabolites using positive/negative switching with HILIC chromatography was performed. Peak areas from the total ion current for each metabolite SRM transition were integrated using MultiQuant v2.1 software (AB/SCIEX). The resulting raw data from the MultiQuant software were analyzed using MetaboAnalyst (http://www.metaboanalyst.ca/MetaboAnalyst/).
Quantification of neurotransmitters from fly heads
Request a detailed protocolFive fly heads per sample (three biological replicates) were homogenized in 190 μL of acidified acetone, 10 μL of 10 mM ascorbic acid and 1 μL of a 5 μg/mL mixture of the corresponding deuterated internal standards (CDN Isotopes, Quebec, Canada). The supernatants were collected, dried, and derivatized using in-house synthesized 6-aminoquinolyl-N-hydroxysuccinimidyl carbamate (AQC). Sample cleanup was done using solid phase extraction (SPE) cartridges (Phenomenex, Inc Hyderabad, India). The eluted samples were completely dried before reconstitution in 2% acetonitrile with 0.5% formic acid and injection into the UHPLC ESI-MS. The liquid chromatography (LC) gradient and electro-spray ionization (ESI) conditions were as described in Ramesh and Brockmann, 2019. The calibration curves were made in neat solvents over a 32-fold concentration range. The highest concentration on column for the different compounds: Tyrosine 64 ng, DOPA 0.16 ng, Dopamine 1.6 ng, Tyramine 0.32 ng, Octopamine 1.6 ng, Histamine 3.2 ng, GABA 160 ng. Quantitation was done using the Xcalibur software (version 2.2 SP1.48).
Neuron counts
Request a detailed protocolNeurons were counted according to the protocol developed by Ordonez et al., 2018; Olsen and Feany, 2019. Flies were fixed in formalin, embedded in paraffin, and 2 μm serial frontal sections were prepared through the entire fly brain. Slides were processed through xylene, ethanols, and into water and stained with hematoxylin. Images of the medulla brain region were taken at 40x magnification using the SPOT software. The neurons were counted and normalized to a pixel aspect ratio of 2.6 pixels/µm in the FIJI (ImageJ) software. For each fly genotype, six brains were imaged and analyzed.
Data availability
All data generated or analyzed during this study are included in the manuscript and supporting files. Additional metabolomics data are available in our previous publication (https://doi.org/10.1101/gad.282277.116).
References
-
Mitochondria, OxPhos, and neurodegeneration: cells are not just running out of gasJournal of Clinical Investigation 129:34–45.https://doi.org/10.1172/JCI120848
-
GFP reporters detect the activation of the Drosophila JAK/STAT pathway in vivoGene Expression Patterns 7:323–331.https://doi.org/10.1016/j.modgep.2006.08.003
-
Life span extension and neuronal cell protection by Drosophila nicotinamidaseJournal of Biological Chemistry 283:27810–27819.https://doi.org/10.1074/jbc.M804681200
-
AMPK at the nexus of energetics and agingCell Metabolism 20:10–25.https://doi.org/10.1016/j.cmet.2014.03.002
-
Effect of tyrosine on cognitive function and blood pressure under stressBrain Research Bulletin 33:319–323.https://doi.org/10.1016/0361-9230(94)90200-3
-
L-tyrosine ameliorates some effects of lower body negative pressure stressPhysiology & Behavior 57:223–230.https://doi.org/10.1016/0031-9384(94)00278-D
-
A Drosophila mitochondrial complex I deficiency phenotype arrayFrontiers in Genetics 10:245.https://doi.org/10.3389/fgene.2019.00245
-
Age-related decline in mitochondrial bioenergetics: does supercomplex destabilization determine lower oxidative capacity and higher superoxide production?Seminars in Cell & Developmental Biology 23:758–767.https://doi.org/10.1016/j.semcdb.2012.04.002
-
Regulation of pyruvate metabolism and human diseaseCellular and Molecular Life Sciences 71:2577–2604.https://doi.org/10.1007/s00018-013-1539-2
-
Overexpression of Glucose-6-phosphate Dehydrogenase Extends the Life Span of Drosophila melanogasterJournal of Biological Chemistry 283:32492–32499.https://doi.org/10.1074/jbc.M805832200
-
Overexpression of nmnat improves the adaption of health span in aging DrosophilaExperimental Gerontology 108:276–283.https://doi.org/10.1016/j.exger.2018.04.026
-
The role of nitisinone in tyrosine pathway disordersCurrent Rheumatology Reports 16:457.https://doi.org/10.1007/s11926-014-0457-0
-
Dissecting the mechanisms underlying unusually successful human health span and life spanCold Spring Harbor Perspectives in Medicine 6:a025098.https://doi.org/10.1101/cshperspect.a025098
-
Catecholamine synthesis: physiological coupling to precursor supplyBiochemical Pharmacology 35:875–881.https://doi.org/10.1016/0006-2952(86)90071-7
-
Characterization of Drosophila tyramine beta-hydroxylase gene and isolation of mutant flies lacking octopamineThe Journal of Neuroscience 16:3900–3911.
-
Histology and survival in age-delayed low-tryptophan-fed ratsMechanisms of Ageing and Development 43:79–98.https://doi.org/10.1016/0047-6374(88)90099-1
-
Minocycline effect on life and health span of Drosophila melanogasterAging and Disease 3:352.
-
Using artificial diets to understand the nutritional physiology of Drosophila melanogasterCurrent Opinion in Insect Science 23:104–111.https://doi.org/10.1016/j.cois.2017.07.014
-
Insights from Drosophila on mitochondrial complex ICellular and Molecular Life Sciences 77:607–618.https://doi.org/10.1007/s00018-019-03293-0
-
The genetic tyrosinemiasAmerican Journal of Medical Genetics Part C: Seminars in Medical Genetics 142C:121–126.https://doi.org/10.1002/ajmg.c.30092
-
Tyrosine administration prevents hypoxia-induced decrements in learning and memoryPhysiology & Behavior 59:867–871.https://doi.org/10.1016/0031-9384(95)02107-8
-
Tyrosine reverses a cold-induced working memory deficit in humansPharmacology Biochemistry and Behavior 47:935–941.https://doi.org/10.1016/0091-3057(94)90299-2
-
Brain tyrosine increases after treating with prodrugs: comparison with tyrosineJournal of Pharmacy and Pharmacology 41:789–791.https://doi.org/10.1111/j.2042-7158.1989.tb06368.x
-
Expression of hsp22 and hsp70 transgenes is partially predictive of Drosophila survival under normal and stress conditionsThe Journals of Gerontology Series A: Biological Sciences and Medical Sciences 64A:828–838.https://doi.org/10.1093/gerona/glp054
-
Enhanced energy metabolism contributes to the extended life span of calorie-restricted Caenorhabditis elegansJournal of Biological Chemistry 287:31414–31426.https://doi.org/10.1074/jbc.M112.377275
Article and author information
Author details
Funding
National Institute on Aging (K99/R00 AG057792)
- Andrey A Parkhitko
National Institutes of Health (5P01CA120964)
- Norbert Perrimon
National Centre for Biological Sciences (12P4167)
- Axel Brockmann
American Foundation for Aging Research
- Norbert Perrimon
The funders had no role in study design, data collection and interpretation, or the decision to submit the work for publication.
Acknowledgements
We thank Trudy Mackay for providing the B3, O1 and O3 flies, John Tower for the GeneSwitch fly stocks and hsp22-GFP line, Scott Pletcher for providing the TIGS-2 and 3XElav Gene-Switch driver lines, David Walker for providing UAS-dPGC-1/Spargel line, Mel Feany for providing Syb-QF2; QUAS- α-synuclein line, Udai Pandey for GFP-CL1 line, Dirk Bohmann for GstD-GFP line. We thank Jay Hirsh for comments on measuring neurotransmitters and Kit Tuen for excellent technical assistance. We thank Stephanie Mohr for critical reading of the manuscript. We thank the TRiP at Harvard Medical School supported by NIH/NIGMS R01-GM084947 for providing transgenic RNAi lines and NCBS institutional fund to AB (12P4167) to support the quantification of neurotransmitters. This work was supported by NIA K99/R00 AG057792 (AP), 5P01CA120964 (NP) and AFAR (NP). NP is an investigator of the Howard Hughes Medical Institute.
Copyright
© 2020, Parkhitko et al.
This article is distributed under the terms of the Creative Commons Attribution License, which permits unrestricted use and redistribution provided that the original author and source are credited.
Metrics
-
- 5,039
- views
-
- 550
- downloads
-
- 40
- citations
Views, downloads and citations are aggregated across all versions of this paper published by eLife.
Citations by DOI
-
- 40
- citations for umbrella DOI https://doi.org/10.7554/eLife.58053