Delilah, prospero, and D-Pax2 constitute a gene regulatory network essential for the development of functional proprioceptors
Abstract
Coordinated animal locomotion depends on the development of functional proprioceptors. While early cell-fate determination processes are well characterized, little is known about the terminal differentiation of cells within the proprioceptive lineage and the genetic networks that control them. In this work we describe a gene regulatory network consisting of three transcription factors–Prospero (Pros), D-Pax2, and Delilah (Dei)–that dictates two alternative differentiation programs within the proprioceptive lineage in Drosophila. We show that D-Pax2 and Pros control the differentiation of cap versus scolopale cells in the chordotonal organ lineage by, respectively, activating and repressing the transcription of dei. Normally, D-Pax2 activates the expression of dei in the cap cell but is unable to do so in the scolopale cell where Pros is co-expressed. We further show that D-Pax2 and Pros exert their effects on dei transcription via a 262 bp chordotonal-specific enhancer in which two D-Pax2- and three Pros-binding sites were identified experimentally. When this enhancer was removed from the fly genome, the cap- and ligament-specific expression of dei was lost, resulting in loss of chordotonal organ functionality and defective larval locomotion. Thus, coordinated larval locomotion depends on the activity of a dei enhancer that integrates both activating and repressive inputs for the generation of a functional proprioceptive organ.
Editor's evaluation
The study provides compelling evidence for a gene regulatory network involved in generating different sensory cell types from a common progenitor. The careful work shows how an enhancer can integrate the antagonistic relationship between two transcription factors for Drosophila sensory system development.
https://doi.org/10.7554/eLife.70833.sa0Introduction
A central question in developmental biology is how different cells that originate in the same lineage and develop within the same organ, acquire unique identities, properties and specialized morphologies. One of the common mechanisms involved in cell fate diversification within a cell lineage is asymmetric cell division in which cytoplasmic determinants of the mother cell differentially segregate into one of the two daughter cells. This asymmetry is then translated into differential gene expression and the activation of cell-type-specific gene regulatory networks (GRN) that dictate the differentiation programs of cells with unique properties. The transition from a primary cell fate to the characteristic phenotype of a fully differentiated cell involves complex GRNs in which numerous genes regulate each other’s expression. Despite this complexity, genetic analyses in well-characterized developmental systems can often reveal elementary interactions in small GRNs which dictate a specific cell fate, or a specific feature of the differentiating cell.
Many of the core components and the central processes underlying asymmetric cell divisions and primary cell fate decisions have been uncovered in studies performed on the central and peripheral nervous system (PNS) of Drosophila (e.g. Knoblich, 2008; Schweisguth, 2015). The PNS of Drosophila contains two classes of multicellular sensory organs, external sensory organs and chordotonal organs (ChOs), whose lineages share a similar pattern of asymmetric cell divisions (Lai and Orgogozo, 2004). In both types of organs, the neuron and support cells, which collectively comprise the sensory organ, arise from a single sensory organ precursor cell (SOP) through a sequence of precisely choreographed asymmetric cell divisions. Antagonistic interactions involving Notch and Numb are key regulators of the asymmetry generated between each two sibling cells within these lineages (Gönczy, 2008; Rebeiz et al., 2011; Reeves and Posakony, 2005). Unlike the primary cell-fate specification, which has been extensively investigated, the process of terminal differentiation of the post-mitotic progeny is poorly understood. We are using the larval lateral pentascolopidial ChO (LCh5) as a model system to study cell fate diversification within a sensory lineage.
The LCh5 organ is composed of five mechano-sensory units (scolopidia) that are attached to the cuticle via specialized epidermal attachment cells. Each of the five scolopidia originates in a single precursor cell that divides asymmetrically to generate five of the six cell types that construct the mature organ: the neuron, scolopale, ligament, cap, and cap-attachment cell (Brewster and Bodmer, 1995; Figure 1A–C). Three of the five cap-attachment cells are rapidly removed by apoptosis, leaving two cap-attachment cells that anchor the five cap cells to the epidermis (Avetisyan and Salzberg, 2019). Later in development, following the migration of the LCh5 organ from the dorsal to the lateral region of the segment, a single ligament-attachment cell is recruited from the epidermis to anchor the five ligament cells to the cuticle (Inbal et al., 2004). The mature LCh5 organ responds to mechanical stimuli generated by muscle contractions that lead to relative displacement of the attachment cells and the consequent shortening of the organ (Hassan et al., 2019).
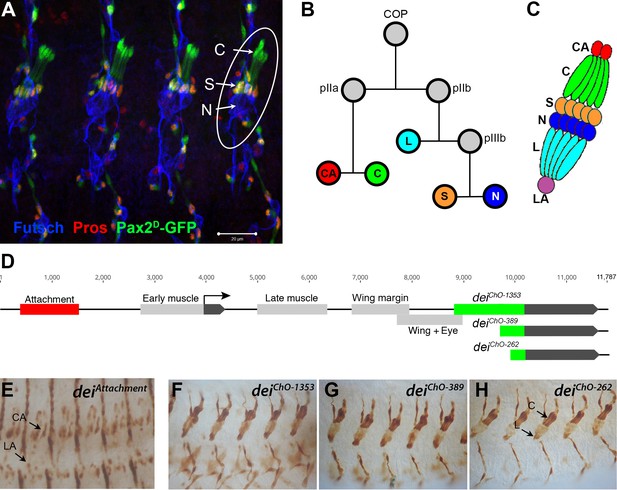
The LCh5 organ and the dei gene.
(A) Four abdominal segments of a stage 16 embryo carrying the sv/Pax2D1-GFP reporter (green) which labels the cap and scolopale cells, stained with anti-Pros (red) which labels the nuclei of scolopale cells, and anti-Futsch (blue), which labels the neurons. One LCh5 organ is circled and the cap cells (C), scolopale cells (S) and neurons (N) are indicated. The cap-attachment, ligament-attachment and ligament cells are not stained. Scale bar = 20 μm. (B–C) the ChO lineage (B) and schematic illustration of an LCh5 organ (C). The neurons are depicted in blue, the scolopale cells in orange, the ligament cells in cerulean, the cap cells in green, the cap-attachment cells in red and the ligament-attachment cell in purple. (D) Schematic representation of the dei locus showing the two exons (black boxes) and the cis regulatory modules (CRM) that drive gene expression within the ChO lineage: the deiattachment CRM which drives expression in the attachment cells (red box) and the deiChO CRM which drives expression in the cap and ligament cells (green box). The deiChO CRM was originally mapped to a 1353 bp fragment (deiChO-1353) and was then delimited to a smaller 389 bp fragment located immediately upstream to the second exon (deiChO-389). As part of this work, the ChO-specific CRM was further delimited to a 262 bp fragment (deiChO-262). (E–H) The embryonic expression patterns driven by the deiattachment (E), deiChO-1353 (F), deiChO-389 (G), and deiChO-262 (H) enhancers are shown. C, cap cell; L, ligament cell; CA, cap-attachment cell, LA, ligament-attachment cell.
Very little is known about the unique cell-type-specific differentiation programs that characterize each of the ChO cells, whose morphologies and mechanical properties differ dramatically from each other. To address this issue, we focus on the transcription factor Taxi wings/Delilah (Dei), an important regulator of cell adhesion (Egoz-Matia et al., 2011), which is expressed in the four accessory cell types (cap, ligament, cap-attachment and ligament-attachment) but is excluded from the neuron and the scolopale cell. Even though dei is expressed in all four accessory cells, its expression in these cells is differentially regulated. The transcription of dei in the ChO is controlled by two cis-regulatory modules (CRMs): The deiattachment enhancer, located ~2.5 Kb upstream of the dei transcription start site, drives expression in the cap-attachment and ligament-attachment cells (as well as tendon cells), whereas the deiChO-1353 enhancer, an intronic 1353 bp DNA fragment, drives dei expression specifically in the cap and ligament cells (Nachman et al., 2015; Figure 1D–F). The deiattachment enhancer was shown to be activated by the transcription factor Stripe, which is considered a key regulator of tendon cell development (Becker et al., 1997) and a known determinant of attachment cell identity (Klein et al., 2010). Here, we provide a high-resolution dissection of the deiChO-1353 enhancer and show that it integrates both activating and repressive cues to drive dei expression in cap and ligament cells while suppressing it in scolopale cells. We find that D-Pax2/Shaven (Sv), which is expressed in both branches of the cell lineage, is a positive regulator of dei and that Prospero (Pros) inhibits dei expression specifically in the scolopale cell. This small GRN is required for the realization of differentiation programs characterizing cap versus scolopale cell fates and is therefore essential for ChO functionality and coordinated larval locomotion.
Results
Identifying D-Pax2/Sv as a putative direct activator of dei expression
To reveal the gene network that regulates dei expression in the ChO cells, we used the previously identified ChO-specific deiChO-1353 enhancer or the shorter version of it - deiChO-389, both described in Nachman et al., 2015; Figure 1D and F–G, as an entry point. As part of this work, we have further narrowed down the critical regulatory region to an evolutionarily conserved 262 bp fragment, deiChO-262, which drives ChO-specific expression in a pattern indistinguishable from the expression pattern driven by the larger fragments deiChO-1353 and deiChO-389 (Figure 1D, F and H). These regulatory fragments were used in two types of screens aimed at identifying genes that regulate the expression of dei in the ChO lineage. The first screen was an RNAi-based phenotypic screen conducted in larvae (described in Hassan et al., 2018). It capitalized on a reporter fly strain in which the cap and ligament cells expressed green fluorescent protein (GFP) under the regulation of the deiChO-1353 enhancer, while the attachment cells expressed red fluorescent protein (RFP) under the regulation of the deiattachment enhancer (Halachmi et al., 2016). One of the 31 genes identified in that screen as being required for normal morphogenesis of the larval LCh5 organ was shaven (sv), which encodes for the Drosophila Pax2 homologue D-Pax2. The knockdown of sv within the ChO lineage led to loss of expression of the deiChO-1353 reporter from the cap cells, suggesting that D-Pax2 is a positive regulator of dei transcription in this cell type (Hassan et al., 2018).
Here, we describe the second screen, a yeast one hybrid (Y1H) screen, aimed at identifying proteins that bind directly to the deiChO-389 enhancer. The screen was performed by Hybergenics Services on a Drosophila whole embryo library (ULTImate Y1H screen vs Drosophila Whole Embryo RP2 0–12 + 12-24 hr), using the deiChO-389 sequence as a bait. Screening was performed using the Aureobasidin A selection system (described in detail in the Materials and methods section). Out of 124 million clones screened, 146 clones that were found to grow on the selective medium containing 400 ng/ml of the yeast antibiotic agent Aureobasidin-A were sequenced (Supplementary file 1). Two proteins were identified to bind the bait with very high confidence in the interaction: D-Pax2/Sv (eight independent clones) and LamC (21 independent clones). Three additional candidates were identified as binders with moderate confidence in the interaction: Fax (one clone), Lola (two independent clones), and Toy (three independent clones) (Figure 1—figure supplement 1 and Supplementary file 1). Together, the results of the two independent screens identified D-Pax2/Sv as a putative direct transcriptional activator of dei and an important player in ChO development.
D-Pax2/Sv activates dei expression in the cap cell
To validate the sv RNAi-induced phenotype, we characterized the LCh5 organs of sv mutant embryos (a null allele - sv6). In accordance with the knockdown phenotypes, the expression of dei in the cap cell was abolished in sv-deficient embryos (Figure 2A–B). The expression of additional genes associated with proper differentiation of the cap cell, such as αTub85E, was reduced as well (Figure 2C–D). The loss of sv did not eliminate the expression of either scolopale-specific proteins (Crumbs, Pros) or neuronal markers (Futsch, Elav, Nrg), suggesting that it did not affect primary cell fate decisions, however, its loss prevented normal morphogenesis of the sensory unit (Figure 2E–H).
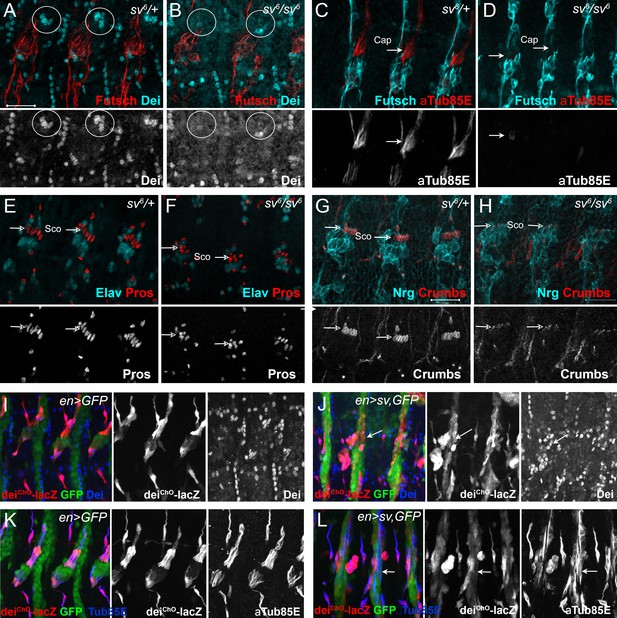
Sv/D-Pax2 activates dei expression in the cap cell.
(A–B) Representative abdominal segments of stage 16 embryos stained for Dei (cyan) and the neuronal marker Futcsh (red). The anti-Dei staining is shown separately in the lower panel. (A) A sv6 heterozygous embryo demonstrating the expression of Dei in the cap cell nuclei (circled). (B) The expression of Dei is lost in homozygous sv6 embryos. (C–D) Heterozygous (C) and homozygous (D) sv6 embryos stained for αTub85E (red) and Futsch (cyan). The arrows mark the cap cells. The anti-αTub85E staining is shown separately in the lower panel. (E–F) Heterozygous (E) and homozygous (F) sv6 embryos stained for Elav (cyan) and Pros (red). The arrows mark the nuclei of scolopale cells. The anti-Pros staining is shown separately in the lower panel. (G–H) Heterozygous (G) and homozygous (G) sv6 embryos stained for Nrg (cyan) and Crumbs (red). The arrows mark the nuclei of scolopale (Sco) cells. The anti-Crumbs staining is shown separately in the lower panel. (I–L) Representative abdominal segments of stage 16 embryos that express GFP (I, K) or GFP and sv (J, L) under the regulation of en-Gal4. The embryos carry the deiChO-262-lacZ marker (anti bGal staining is shown in red) and are stained with anti-Dei (blue in I-J) or anti-αTub85E (blue in K-L). The staining patterns of deiChO-262-lacZ, Dei and αTub85E are shown separately on the right. Note the ectopic expression of Dei, deiChO-262-lacZ and αTub85E in epidermal cells within the en domain (arrows in J, L).
To further test the ability of D-Pax2/Sv to activate dei expression in vivo, we ectopically expressed sv under the regulation of en-Gal4 and examined the resulting changes in gene expression patterns. The ectopic expression of sv led to ectopic expression of both the endogenous dei gene and the deiChO-262 transcriptional reporter, as well as the cap cell marker αTub85E (Figure 2I–L). The ectopic expression of D-Pax2 in the en domain had detrimental effects on the pattern of ChO migration and possibly on other morphogenetic processes in the embryo. Thus, the identification of cells based on their position was not feasible in these embryos. However, this experiment clearly showed that ectopic expression of D-Pax2 could activate expression of the dei gene and the deiChO-262-lacZ reporter in epidermal cells within the en domain. Thus, D-Pax2/Sv ability to activate dei is not restricted to the ChO lineage, or the peripheral nervous system in general. Altogether, these observations corroborate the notion that D-Pax2/Sv is an activator of dei that plays a critical role in ChO morphogenesis.
Pros represses dei in the scolopale cell
The sv gene is expressed broadly within the ChO lineage during early stages of organ development and is then gradually restricted to the cap and scolopale cells (Czerny et al., 1997; Fu et al., 1998; Fu and Noll, 1997), where its expression level remains high during late embryogenesis and larval stages. Yet, the expression of its downstream target gene dei is normally activated in the cap cell but is excluded from the scolopale cell. This discrepancy in the expression pattern of D-Pax2/Sv and dei may point to the presence of a scolopale-specific repressor that prevents the transcription of dei in this D-Pax2/Sv-expressing cell. The results of the abovementioned RNAi screen identified the transcription factor Pros as a good candidate for being that repressor. pros and sv RNAi had opposite effects on the expression of the deiChO-1353-GFP reporter. Although the knockdown of sv led to a loss of the reporter expression from the cap cell, the knockdown of pros led to expansion of its expression into the scolopale cell suggesting that, normally, Pros represses dei in this cell (Hassan et al., 2018).
To validate the pros knockdown phenotypes and to further test the idea that Pros acts as a repressor of dei in the scolopale cell, we characterized the ChOs of pros17 embryos. The loss of pros led to ectopic expression of both the endogenous dei gene and the deiChO-262 reporter, as well as the cap cell marker αTub85E in the scolopale cells of the LCh5 organs (Figure 3A–B). Similar effect was evident in the scolopale cells of the LCh1 and VChA/B organs (Figure 3—figure supplement 1). The pros-deficient scolopale cells developed into cap-like cells rather than ligament-like or attachment cell-like cells. This was indicated by the upregulation of dei which was not accompanied by upregulation of the transcription factor Sr that is normally co-expressed with Dei in the ligament and attachment cells but is excluded from the cap cells (Figure 3C–D). The observed alterations in gene expression pattern do not reflect a full scolopale-to-cap cell fate transformation, as the Pros-deficient scolopale cells still maintain some of their scolopale-specific characteristics, such as Eyes Shut and α—Catenin expression (Figure 3E–H). As the number of neurons, ligament, cap, and cap-attachment cells remained normal in pros mutant embryos (Figure 3—figure supplement 2), we conclude that Pros does not influence primary cell-fate decisions within the LCh5 lineage.
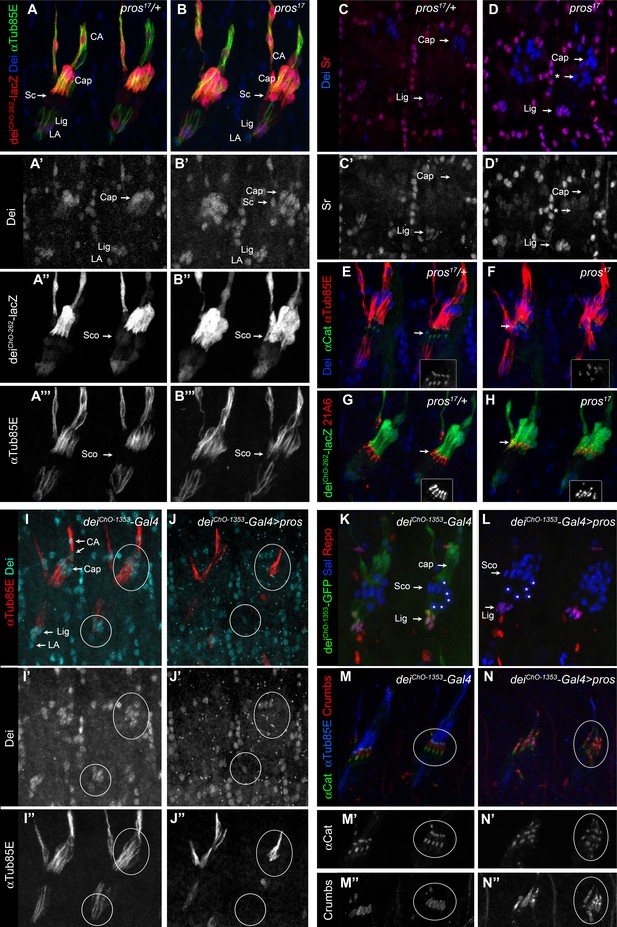
Pros represses dei in the scolopale cell.
(A–B) Representative abdominal segments of stage 16 pros17 heterozygous (A) and homozygous (B) embryos carrying the deiChO-262-lacZ marker (shown in red) and stained for Dei (blue) and αTub85E (green). Each of the three channels is shown separately below. Note the expansion of Dei and the deiChO-262-lacZ marker into the scolopale cells in pros17 mutant embryos. The arrows point to the scolopale cells. (C–D) pros17 heterozygous (C) and homozygous (D) embryos stained for Dei (blue) and Sr (red). Note that the ectopic expression of Dei in the scolopale cells of pros mutant embryo is not accompanied by ectopic expression of Sr (the arrow labeled with asterisks in D). (E–F) pros17 heterozygous (E) and homozygous (F) embryos carrying an α-Catenin-GFP reporter and stained for Dei (blue, shown separately in the inset) and αTub85E (red). (G–H) pros17 heterozygous (G) and homozygous (H) embryos carrying the deiChO-262-lacZ reporter (shown in green) and stained with the scolopale marker anti-Eyes Shut (MAb21A6, red, shown separately in the inset). Note that the expression of both Eyes Shut/21A6 and α—Catenin is maintained in the pros-deficient scolopale cells. (I–J) deiChO-1353-Gal4 (I) and deiChO-1353> UAS pros (J) embryos stained for Dei (cyan, shown separately in I’-J’) and αTub85E (red, shown separately in I’’-J’’). The cap and ligament cells are circled. Note the loss of Dei and αTub85E expression upon Pros expression. (K–L) deiChO-1353 (K) and deiChO-1353> UAS pros (L) embryos carrying the deiChO-1353-lacZ reporter (shown in green) stained for Sal (blue) and Repo (red). The cap, scolopale (Sco) and ligament (Lig) cells are indicated; the asterisks mark oenocyte cell nuclei. (M–N) deiChO-1353-Gal4 (M) and deiChO-1353> UAS pros (N) embryos carrying an α—Catenin-GFP reporter (green, shown separately in M’-N’) and stained for Crumbs (red, shown separately in M’’-N’’) and αTub85E (blue). Note the duplication of scolopale-specific structures in the cap cell expressing Pros ectopically (circled).
The ability of Pros to repress dei was not restricted to the scolopale cell. Ectopic expression of pros in the LCh5 lineage, using a deiChO-1353-Gal4 driver, abolished the expression of both the endogenous dei gene and a deiChO-1353-GFP reporter in the cap and ligament cells as well (Figure 3I–L). In parallel to the repression of dei, ectopic expression of pros was sufficient for upregulating scolopale-specific genes and, moreover, for driving the formation of ectopic scolopale-specific structures within the affected cap cells. Most notably, the Pros-expressing cap cells manifested scolopale rods and ectopically expressed Crumbs and α-Catenin in a scolopale-characteristic pattern (Figure 3M–N).
D-Pax2/Sv and Pros regulate the transcription of dei via deiChO-262
The fact that the expression of the deiChO transcriptional reporters was affected similarly to the endogenous dei gene by both sv and pros loss- and gain-of-function, suggested that both D-Pax2/Sv and Pros regulate dei’s transcription in the ChOs through this regulatory module. To test this hypothesis, we deleted the deiChO-262 region from the fly genome by CRISPR/Cas9-mediated genome editing, resulting in a new regulatory allele of dei (dei∆ChO) (Figure 4—figure supplement 1). To verify that the deletion of this intronic enhancer does not affect splicing of the transcript, cDNA was synthesized from homozygous deiΔChO and control flies. A 416 bp fragment was amplified by PCR from the cDNA samples using primers located on both sides of the intron (in the 1st and 2nd exons) (Figure 4—figure supplement 1B and Materials and methods). Sequencing of the PCR products verified the presence of normally structured dei transcript in deiΔChO mutants (Figure 4—figure supplement 1C).
As expected, in homozygous dei∆ChO embryos the expression of dei was lost from the cap and ligament cells but remained intact in the rest of the dei-expressing cells: CA, LA and tendon cells (Figure 4A–B). This observation strongly suggests that the deiChO-262 enhancer constitutes the sole regulatory module driving dei expression in the cap and ligament cells.
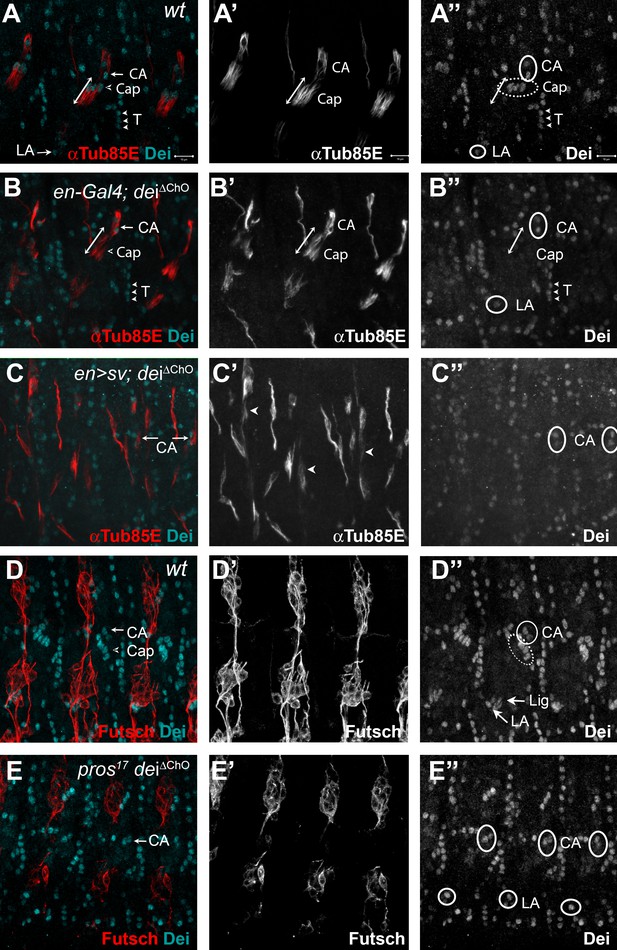
D-Pax2/Sv and Pros regulate the transcription of dei via the deiChO-262 regulatory module.
(A–B) Stage 16 wt (A) and dei∆ChO (B) embryos stained for Dei (cyan) and αTub85E (red). Note that in (B) Dei is still evident in the cap-attachment and ligament-attachment cells (CA and LA, arrows in A, circled in A’’), as well as in tendon cells (T; arrowheads), but is lost from the cap and ligament cells. The double edge arrows in A and B demarcate the length of the cap cells. (C) An embryo in which sv was expressed under the regulation of en-Gal4 in a dei∆ChO background. Sv was unable to induce Dei expression in the cap and ligament cells in the absence of the deiChO-262 enhancer. Ectopic expression of αTub85E is evident within the en domain (arrowheads in C’). (D–E) wt (D) and A pros17 dei∆ChO homozygous embryo (E) stained for Dei (cyan) and anti-Futsch (red). The pros17 dei∆ChO embryo (E) presents a dei∆ChO-like and not pros17-like expression pattern of the Dei protein, indicating that the dei∆ChO deletion is epistatic to pros loss-of-function. The neurons present the typical pros axonal pathfinding defects.
To further test the notion that D-Pax2/Sv regulates dei expression via the deiChO-262 enhancer we examined the ability of D-Pax2/Sv to activate dei expression in the dei∆ChO background. We found that ectopic expression of D-Pax2/Sv failed to induce ectopic dei expression in the dei∆ChO background (Compare Figures 4C and 2L), indicating that the deiChO-262 enhancer is indispensable for the ability of D-Pax2/Sv to activate dei transcription. In a complementary experiment, we tested the effect of pros loss-of-function on the expression of dei in the dei∆ChO background. We found that the deiΔChO regulatory mutation was epistatic to pros loss-of-function, so that no ectopic expression of dei was observed in the pros-deficient scolopale cells in embryos homozygous for the dei∆ChO mutation (Compare Figure 4D–E and Figure 3B and D). Based on these results, we concluded that the expression of dei in ChOs depends on the presence of the deiChO-262 regulatory region and that this enhancer integrates the positive and negative inputs of D-Pax2/Sv and Pros, respectively, to drive a lineage-specific dei expression.
D-Pax2/Sv and Pros are direct transcriptional regulators of dei
Our genetic analyses revealed that D-Pax2/Sv and Pros regulate the expression of dei in opposing manners through the function of the deiChO-262 enhancer. In addition, the Y1H screen identified a direct interaction between D-Pax2/Sv and deiChO-389 (which includes the deiChO-262 module). We therefore hypothesized that the deiChO-262 enhancer contains binding sites for D-Pax2/Sv and Pros. Motif search analysis predicted that the deiChO-262 sequence encodes one canonical binding site for D-Pax2/Sv (Figure 5B, D-Pax2/Sv Site 2). On the other hand, we were unable to predict binding sites for Pros in the deiChO-262 sequence.
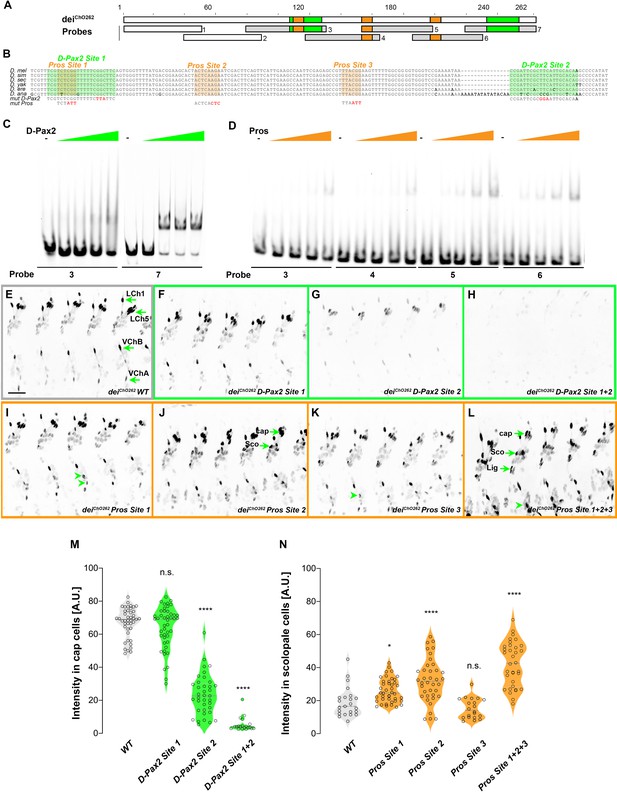
deiChO-262 contains two binding sites for the activator D-Pax2/Sv and three binding sites for the repressor Pros.
(A) Schematic representation of the deiChO-262 enhancer. Green and orange boxes represent the location of the D-Pax2/Sv and Pros binding sites identified by systematic EMSAs using oligos corresponding to the regions represented by Boxes 1–7. (B) Sequence alignment between six Drosophila species for the region of the deiChO-262 enhancer containing the three D-Pax2/Sv and Pros sites (labeled and highlighted in green and orange, respectively). Dashes indicate gaps in the aligned sequence. Mutations of the D-Pax2/Sv and Pros sites used for the in vivo assays are shown at the bottom. (C) D-Pax2/Sv binds to two sites in deiChO-262, one low affinity site in fragment 3 (D-Pax2 site 1) and one high affinity site in fragment 7 (D-Pax2 site 2), as demonstrated with EMSA. The full screen is shown in Figure 5—figure supplement 1. (D) Pros binds to three sites in deiChO-262: Pros site 1 in fragment 3, Pros site 2 in an overlapping sequence in fragments 4 and 5, Pros site 3 in fragment 6, as demonstrated with EMSA. The full screen is shown in Figure 5—figure supplement 2. (E–L) Expression of wild-type (E) and mutated (F–L) deiChO-262-lacZ reporter constructs in abdominal segments A2-A6 of representative stage 16 embryos. The name of the construct is indicated in the bottom of each panel. The green arrows in E point to the cap cells of the various ChO of one abdominal segment: LCh1-lateral ChO1, LCh5-pentascolopidial organ, VChB and VChA are two ventral ChOs. The green arrows in J and L point to cap, scolopale (Sco) and ligament (Lig) cells of LCh5 organs where elevated level of reporter expression is evident. The arrowheads in I and K point to ligament cells of the ventral ChOs. in (M–N) Quantification of reporter activity in nuclei of cap (M) and scolopale cells (N) from LCh5 of segment A2 in embryos carrying the indicated constructs (n = 10 embryos for each genotype). In violin plots, each point represents an individual nucleus, median is shown as dark gray dashed line. Asterisks denote significant difference from wild-type activities, (*) - p < 0.05, (****) – p < 0.0001, n.s. – not significant (Dunnett’s multiple comparison test).
To test whether D-Pax2/Sv actually binds to the predicted site, and to search for additional D-Pax2/Sv binding sites and for Pros binding sites, we systematically screened the deiChO-262 sequence with electrophoretic mobility shift assays (EMSAs, Figure 5 and Figure 5—figure supplements 1 and 2). As shown in Figure 5C, purified D-Pax2/Sv DNA-binding domain bound strongly to the region containing the predicted binding site (probe 7). In addition, we identified another region (in probe 3), that bound D-Pax-2/Sv at a lower affinity (Figure 5C and Figure 5—figure supplement 1). Using purified Pros-S DNA-binding domain we found that four fragments–probes 3, 4, 5, and 6–bound Pros in vitro (Figure 5D). Comprehensive mutagenesis and competition assays revealed three Pros conserved binding sites within these fragments (Figure 5—figure supplement 2), one of them partially overlapping the low-affinity binding site of D-Pax2/Sv (Figure 5B and Figure 5—figure supplement 3). Interestingly, none of these identified binding sites resemble the known binding sites for Pros identified by target detection assay (TDA) (Hassan et al., 1997), by functional studies (Cook et al., 2003), or by single-cell omics analyses (Bravo González-Blas et al., 2020; Figure 5—figure supplement 4).
To test the in vivo role of the D-Pax2/Sv sites identified in vitro, we mutated them either individually, or in combinations, in the context of a deiChO-262 reporter transgene encoding for nuclear β-galactosidase (the mutations are shown in Figure 5—figure supplement 3). Mutation of the canonical D-Pax2/Sv site 2 reduced the expression level driven by deiChO-262 in cap cells (Figure 5G and M). Mutation of D-Pax2/Sv site 1 had no significant effect on its own (Figure 5F and M) but led to a complete suppression of deiChO-262 function when combined with a mutation in D-Pax2/Sv site 2 (Figure 5H and M). This effect was evident in all types of larval ChOs (Figure 5H). These results suggest that D-Pax2/Sv regulates the expression of dei in cap cells by binding to two D-Pax2/Sv binding sites within the deiChO-262 enhancer.
We next tested whether the Pros sites identified by EMSA function in vivo to suppress deiChO-262 activity in scolopale cells. Mutating the Pros site 2 resulted in ectopic expression of the reporter gene in the scolopale cells of LCh5 (Figure 5J and N). In contrast, disruption of Pros site 1 or site 3 had very small or no detectable effects, respectively, on the expression of deiChO-262 in the scolopale cells of LCh5 (Figure 5I, K and N) but induced ectopic expression in scolopale cells of the LCh1 and VChA/B organs (Figure 5I and K, arrowheads). In addition, the disruption of Pros site 3 led to elevation in the reporter’s level in the LCh5 ligament cells (Figure 5K). Simultaneous mutation of all three Pros binding sites in deiChO-262 resulted in intensified ectopic expression in the scolopale cells of all types of larval ChOs (LCh5, LCh1, VChA/B; Figure 5L and N). Based on these results we conclude that Pros represses dei expression in the scolopale cell by binding to three low-affinity binding sites in deiChO-262 that function additively. It is important to note that the mutations introduced in Pros site 1 also disrupted the low-affinity site for D-Pax2/Sv (site 1, Figure 5B) and affected D-Pax2/Sv binding in vitro (Figure 5—figure supplement 1D). While the D-Pax2/Sv site 1 is dispensable for dei expression in cap cells at the presence of the high-affinity site, it is possible that mutations in Pros site 1 would cause a greater effect on the reporter expression in scolopale cells if the D-Pax2/Sv site would not be disrupted simultaneously.
To test whether the identified regulatory interactions apply to additional types of ChOs, we examined the expression pattern driven by the wild-type and mutated deiChO-262 enhancers in developing femoral ChOs (Figure 5—figure supplement 5). This analysis suggests that the Dei/Pros/D-Pax2 GRN plays a similar role in larval and adult ChOs and that the deiChO-262 enhancer encodes pleiotropic transcription factor binding sites (Preger-Ben Noon et al., 2018) that integrate the activating and repressing inputs of D-Pax2/Sv and Pros, respectively.
The deiChO-262 enhancer is essential for normal ChO function and larval locomotion
We have previously shown that the cap cell plays a crucial role in propagating muscle-generated mechanicals signals to the sensory neuron (Hassan et al., 2019). To test whether dei expression in the cap and ligament cells, mediated solely by the deiChO-262 enhancer, is essential for the proprioceptive function of the ChO, we analyzed the pattern of locomotion of freely moving dei∆ChO larvae and compared it to wild-type larvae and larvae homozygous for the deiKO-mCherry null allele (Hassan et al., 2018). As shown in Figure 6, wild-type larvae crawled persistently 94.5% ± 12.4% (n = 27) of the time with a very few changes of direction or head swipes (Figure 6A, E and H, and Videos 1–2). In contrast, the deiKO-mCherry larvae exhibited frequent changes of moving direction and longer pauses, walking on average only 38.9% ± 19.4% (n = 25) of the time. While pausing, the deiKO-mCherry larvae swiped their heads extensively (Figure 6B, F and H, and Videos 3–4). The dei∆ChO larvae exhibited locomotion phenotypes that were similar to, though slightly less severe and more variable than, those of the deiKO-mCherry larvae (Figure 6C, G and H, Videos 5–6). On average, the dei∆ChO larvae crawled 51.9% ± 24.9% (n = 24) of the time and swiped their heads often; in 15.8% of the time their body angle was higher than 250° or lower than 110°, compared to 9.9% in deiKO-mCherry and 1.2% of the time in wt larvae (Figure 6H). These results demonstrate that the deiChO-262 regulatory element is crucial for proper function of the ChO as its deletion resulted in sensory dysfunction and uncoordinated movement.
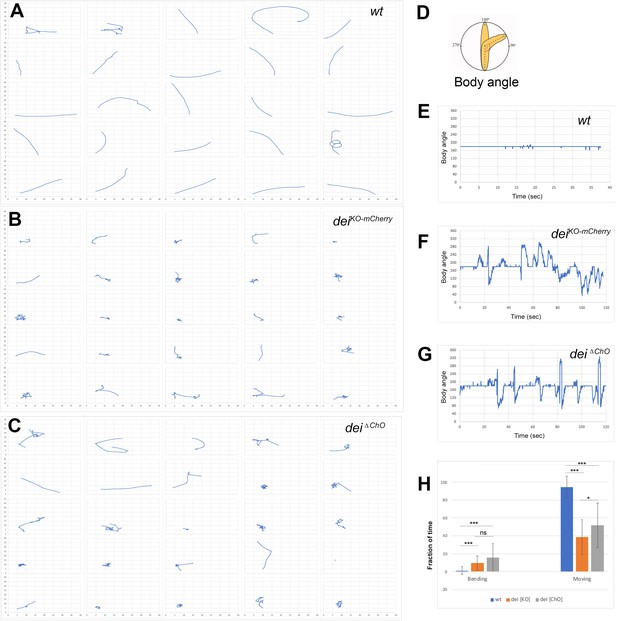
The deiChO-262 enhancer is essential for normal larval locomotion.
(A–C) Crawling trajectories of 25 wild-type (A) deiKO-mCherry (B) and deiΔChO (C) larvae. Each trajectory is shown in a square that represents 40 × 40 mm area. (D) Schematic representation of the body angle, γ, defined as the angle between the head and the body axis. (E–G) Representative time evolutions of the body angle of a wild-type (E), deiKO-mCherry (F) and deiΔChO (G) larvae. The wild-type larva walks persistently, and the body angle stays 180 degrees most of the time (a 40 s interval is shown, after which the larva exited the filmed arena). The deiKO-mCherry and deiΔChO mutant larvae display frequent changes in the direction of motion and long pauses accompanied by extensive head swiping (120 s intervals are shown). (H) A graph showing the average fraction of the time the larvae were crawling (GoPhase) and the fraction of time in which the body was bended more than 70 degrees (the measured angle γ was higher than 250 degrees or lower than 110 degrees). n = 24–27 for all genotypes; error bars represent the standard deviation. *** p < 0.0001, * p < 0.05, ns = non-significant. p Values were calculated using the unpaired two-tailed Mann-Whitney test.
A video showing the locomotion of a wt (Canton-S) larva.
A video showing the locomotion of a wt (Canton-S) larva.
A video showing the locomotion of a deiKO-mCherry larva.
A video showing the locomotion of a deiKO-mCherry larva.
A video showing the locomotion of a deiΔChO larva.
A video showing the locomotion of a deiΔChO larva.
Discussion
Opposing activities of D-Pax2/Sv and Pros dictate cap versus scolopale differentiation programs by regulating the dei gene
In this work, we identify a small GRN that governs the alternative differentiation programs of two cousins once removed cells within the ChO lineage - the cap cell and the scolopale cell. We show that Pros and D-Pax2/Sv are direct regulators of dei that together dictate its expression in the cap cell and its repression in the scolopale cell. Both D-Pax2/Sv and Pros exert their effects on dei transcription via a 262 bp chordotonal-specific enhancer (deiChO-262) in which two D-Pax2/Sv and three Pros binding sites were identified.
Following primary cell fate decisions within the ChO lineage, Pros expression becomes restricted to the scolopale cell (Doe et al., 1991; Vaessin et al., 1991), whereas D-Pax2/Sv expression becomes restricted to the scolopale and cap cells (Figure 1A), similar to its behavior in the external sensory lineages (Johnson et al., 2011; Kavaler et al., 1999). D-Pax2/Sv activates the expression of dei in the cap cell but is unable to do so in the scolopale cell where Pros is co-expressed. If D-Pax2/Sv activity is compromised, the cap cell fails to express dei and loses some of its differentiation markers, such as the expression of αTub85E. In contrast, if Pros activity is lost, dei is ectopically expressed in the scolopale cell that, as a consequence, acquires some cap cell features including the expression of αTub85E (Figure 7). The observed D-Pax2/Sv- and Pros-associated phenotypes do not reflect genuine cell fate transformations, suggesting that D-Pax2/Sv and Pros do not affect primary cell fate decisions within the ChO lineage. Rather, the observed phenotypes reflect a failure of the cap and scolopale cells to follow the cell type-specific differentiation programs responsible for bringing about their characteristic cellular phenotypes. The D-Pax2/Sv-deficient cap cells fail to express unique differentiation markers (such as αTub85E) and are therefore hardly detectable. It is also possible that the Sv/Pax2-deficient cap cells fail to survive. Thus, we cannot exclude the possibility that some of the findings reflect more upstream roles of Sv/D-Pax2 in the specification of cap-cell identity.
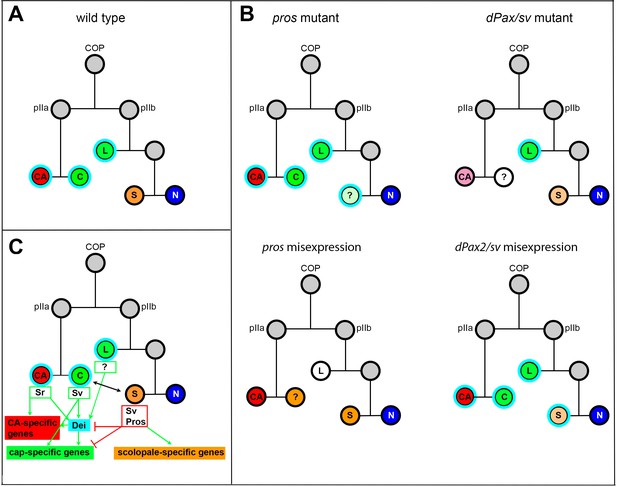
Summary of the relations between Sv, Pros and Dei in the ChO lineage and their effect on ChO development.
(A) A wt ChO lineage. The cap (C) and ligament (L) cells are depicted in green, the scolopale cell (S) is depicted in orange and the neuron (N) is depicted in blue. Cells that expressed Dei are circled in light blue. (B) The loss of pros leads to upregulation of Dei in the scolopale cell and to failure of scolopale cell differentiation. In contrast, the loss of Sv leads to loss of Dei expression from the cap cell and failure of cap cell differentiation. The Sv-deficient scolopale cells are also abnormal. The CA cells which depend on the cap cell for their development/maintenence also appear abnormal is sv mutants. Misexpression of Pros leads to repression of Dei in the cap and ligament cells, preventing their normal differentiation. The Pros-expressing cap cells adopt some scolopale-specific features. In contrast, over-expression of Sv leads to ectopic expression of Dei. Due to the presence of Pros, the level of expression of dei in the scolopale cell is restricted. (C) A schematic summary showing the relations between Pros, Sv and Dei and their relations to cell-type-specific differentiation programs. In the CA cells, dei is activated by Sr via the deiattachment enhancer. Both Sr and Dei are required there for the activation of CA-specific genes. In the cap cell dei is activated by Sv via the deiChO-262 enhancer. Sv is required for activating cap-specific genes in both Dei-dependent and independent ways. In the scolopale cells, dei is repressed by Pros via the deiChO-262 enhancer. Pros is required in addition for activating scolopale-specific genes. Dei is also expressed in the ligament cells and is required for their correct differentiation. The regulators of dei in the ligament cell are yet to be identified.
The switch between the differentiation programs of cap and scolopale identities cannot be simply explained by the nature of asymmetric cell divisions within the ChO lineage. The effects on the ChO lineage of major regulators of asymmetric cell division, such as Notch and Numb, and the expression pattern of cell differentiation determinants such as Pros and D-Pax2/Sv, were mainly postulated based on knowledge gained by analyzing external sensory lineages (Kavaler et al., 1999; Lai and Orgogozo, 2004; Manning and Doe, 1999; Reddy and Rodrigues, 1999). According to the similarity between the lineages, the cap cell parallels the Notch-non-responsive hair (trichogen) cell, whereas the scolopale parallels the Notch- responder sheath (thecogen) cell (based on Rebeiz et al., 2011). Thus, D-Pax2/Sv is expressed in one Notch responder and one non-responder cells in the lineage. The presence of Pros in the Notch-responder cell represses the cap-promoting activity of D-Pax2/Sv. Somewhat similar cousin-cousin cell transformation was found in external sensory organs in the adult where mutations in hamlet transform the sheath cell into a hair cell (parallel to scolopale-to-cap transformation) (Moore et al., 2004). Ectopic expression of hamlet induced pros expression and repressed the hair shaft-promoting activity of D-Pax2/Sv.
In the adult external sensory lineage, Pros was shown to be important for the specification of the pIIb precursor, which gives rise to the neuron and sheath cell (the scolopale counterpart). However, the absence of Pros from the pIIa precursor, which gives rise to the hair and socket cells (the cap and cap-attachment cells counterparts) was even more critical for proper development of this branch of the lineage (Manning and Doe, 1999). This phenomenon is somewhat conserved in the larval ChO. While Pros is required for proper differentiation of the scolopale cell, its absence from the cap cell is critical for adopting the correct differentiation programs within the lineage.
Opposing effects of D-Pax2/Sv and Pros activities on cell differentiation have been also identified in the regulation of neuronal versus non-neuronal cell fate decisions in the developing eye, where they play a role in modulating the Notch and Ras signaling pathway (Charlton-Perkins et al., 2011). Interestingly, in the R7 equivalence group Pros and D-Pax2/Sv can only alter the cell-type-specific differentiation program of cells that already express the other gene (Charlton-Perkins et al., 2011). Similarly, in the ChO lineage, ectopic expression of Pros in the cap and ligament cells transforms the D-Pax2/Sv-positive cap cell toward a scolopale cell identity but does not affect the D-Pax2/Sv-negative ligament cell in a similar fashion, even though the ectopic expression of Pros does repress the transcription of dei in both cell types. Additionally, a loss of Pros activity in the scolopale cell can transform the identity of this cell toward a cap cell identity only in the presence of D-Pax2/Sv.
We have shown that the opposing influences of Pros and D-Pax2/Sv on dei expression is integrated by the deiChO-262 enhancer in both larval and adult ChO lineages. To the best of our knowledge, this is the first example of an enhancer that responds to these opposing signals to dictate cell-specific differentiation programs in a sensory lineage. While the identified enhancer is ChO-specific, it is plausible that other enhancers of sensory organ lineage-specific genes encode coupled Pros and D-Pax2/Sv binding sites. The expression of the dei gene in other (non-ChO) organs is regulated via different enhancers (as described in Nachman et al., 2015). Some of these enhancers are responsible for regulating dei expression in tissues where Pros and Pax2 play opposing roles, such as the eye and wing margin ES organs (the deiwing+eye enhancer; Nachman et al., 2015). It is beyond the scope of this work, but in the future, it will be interesting to decipher whether these enhancers also serve as molecular platforms for integrating opposing effects of Pax2 and Pros.
The deiChO-262 enhancer encodes non-canonical binding sites for Pros and D-Pax2/Sv
We have identified two D-Pax2/Sv and three Pros binding sites in the deiChO-262 enhancer. Apart from D-Pax2/Sv site 2, none of these sites match the published binding motifs for D-Pax2/Sv or Pros. These results agree with recent studies that showed that many transcription factors function in vivo through low-affinity (Crocker et al., 2016; Crocker et al., 2015) or suboptimal (Farley et al., 2015) binding sites that differ from their predicted binding motifs. It was suggested that low-affinity binding sites provide specificity for individual transcription factors belonging to large paralogous families, such as the homeodomain family of transcription factors, that share similar DNA-binding preferences (Crocker et al., 2016; Crocker et al., 2015; Kribelbauer et al., 2019). To compensate for their weak binding capabilities, low-affinity binding sites are often organized in homotypic clusters that can increase the cumulative binding affinity of an enhancer (Crocker et al., 2016; Crocker et al., 2015; Kribelbauer et al., 2019). Our findings, that the homeodomain transcription factor Pros functions through a cluster of low-affinity binding sites in deiChO-262, may represent another example for the suggested tradeoff between transcription factor binding affinity and specificity (Crocker et al., 2016; Crocker et al., 2015).
We do not know how Pros opposes the effect of D-Pax2/Sv in the context of deiChO-262 to inhibit the expression of dei in scolopale cells. Our results suggest that the inhibitory effect of Pros is not mediated through binding competition with D-Pax2/Sv at the D-Pax2/Sv high-affinity site (site 2), since this site does not overlap with a Pros-binding site (Figure 5B). The D-Pax2/Sv low-affinity site does overlap with a Pros binding site and mutations in the Pros binding site affect D-Pax2/Sv binding in vitro (see Figure 5—figure supplement 1D), however, while being important for robust dei expression, this site is dispensable in the presence of the high-affinity site. It is possible that binding of Pros to the deiChO-262 enhancer targets this sequence to a repressed heterochromatin domain as was recently shown for other Pros target genes in differentiating neurons (Liu et al., 2020).
How is dei regulated in other ChO cell types? dei is expressed in four out of six cell types comprising the ChO: the cap-attachment and ligament-attachment cells, in which dei transcription is activated by Sr via the deiattachment regulatory module (Nachman et al., 2015), and the cap and ligament cells in which the expression of dei is regulated via the deiChO-262 enhancer. We now show that D-Pax2/Sv activates dei transcription in the cap cell, and that Pros inhibits its expression in the scolopale cell. The identity of the positive regulator/s of dei in the ligament cell, whose cell-fate is determined by the glial identity genes gcm and repo (Campbell et al., 1994; Halter et al., 1995; Jones et al., 1995), and the identity of the negative regulator/s of dei in the neuron remains unknown. Interestingly, the expression of dei was found to be altered in response to ectopic expression of gcm in the embryonic nervous system; its expression was upregulated at embryonic stage 11, but was repressed in embryonic stages 15–16 (Egger et al., 2002). This observation points to GCM as a potential regulator of dei expression in the ligament cells. Another interesting candidate for repressing dei in the sensory neuron is the transcriptional repressor Lola. Lola has been identified as a putative direct regulator of dei in the Y1H screen and was shown to be required in post-mitotic neurons in the brain for preserving a fully differentiated state of the cells (Southall et al., 2014). The possible involvement of Gcm and Lola in the regulation of dei awaits further studies. The observed upregulation of the deiChO-262 reporter in the ligament cells of embryos with mutated Pros-binding sites may reflect an early role of Pros in the pIIb precursor before its restriction to the scolopale cell, which prevents dei expression in the ligament cell.
The deiChO-262-driven dei expression is critical for organ functionality
Although the loss of dei in the genetic/cellular milieu of the ligament cell (unlike the cap cell), even when accompanied by ectopic expression of Pros, is not sufficient for transforming ligament cell properties towards those of scolopale cells, we know that the expression of dei in the ligament cell is critical for its proper development. Ligament-specific knockdown of dei leads to failure of the ligament cells to acquire the right mechanical properties and leads to their dramatic over-elongation (Hassan et al., 2018). By analysing the locomotion phenotypes of larvae homozygous for a dei null allele and the newly generated cap and ligament-specific dei∆ChO allele, we could show that the expression of dei in the cap and ligament cells is crucial for normal locomotion. Thus, we conclude that the correct expression of dei within the ChO is critical for organ functionality. Surprisingly, the gross morphology of LCh5 of dei∆ChO larvae appears normal (Data not shown). Yet, in a way that remains to be elucidated, the Dei-deficient cap and ligament cells fail to correctly transmit the cuticle deformations to the sensory neuron, most likely due to changes in their mechanical properties.
Materials and methods
Reagent type (species) or resource | Designation | Source or reference | Identifiers | Additional information |
---|---|---|---|---|
Gene (Drosophila melanogaster) | dei/tx | FlyBase | CG5441, FBgn0263118 | |
Gene (Drosophila melanogaster) | pros | FlyBase | CG17228, FBgn0004595 | |
Gene (D. melanogaster) | sv/D-Pax2 | FlyBase | CG11049, FBgn0005561 | |
Genetic reagent (D. melanogaster) | sv6/act-GFP | Kavaler et al., 1999 | N/A | |
Genetic reagent (D. melanogaster) | Dpax2D1-GFP | Johnson et al., 2011 | N/A | |
Genetic reagent (D. melanogaster) | pros17/TM6B, Tb1 | Bloomington Drosophila Stock Center | BDSC:5458 | |
Genetic reagent (D. melanogaster) | deiChO-1353-GFP,deiattachment-RFP;en-Gal4 | Halachmi et al., 2016 | N/A | |
Genetic reagent (D. melanogaster) | deiKO-mCherry | Hassan et al., 2018 | N/A | |
Genetic reagent (D. melanogaster) | en-Gal4 | Brand and Perrimon, 1993 | ||
Genetic reagent (D. melanogaster) | P{UAS-3xFLAG-pros.S}14 c, y1 w*; Pin1/CyO | Bloomington Drosophila Stock Center | BDSC:32245 | |
Genetic reagent (D. melanogaster) | UAS-sv-RNAi | Vienna Drosophila Resource Center | VDRC:107343 | |
Genetic reagent (D. melanogaster) | UAS-sv | Kavaler et al., 1999 | N/A | |
Genetic reagent (D. melanogaster) | UAS-D-α-Catenin-GFP | Oda and Tsukita, 1999 | N/A | |
Genetic reagent (D. melanogaster) | deiattachment-lacZ | Nachman et al., 2015 | N/A | |
Genetic reagent (D. melanogaster) | deiChO-1353-lacZ | Nachman et al., 2015 | N/A | |
Genetic reagent (D. melanogaster) | deiChO-1353 -GFP | Halachmi et al., 2016 | N/A | |
Genetic reagent (D. melanogaster) | deiChO-389 -lacZ | Nachman et al., 2015 | N/A | |
Genetic reagent (D. melanogaster) | deiChO-Gal4 | This study | N/A | |
Genetic reagent (D. melanogaster) | deiChO-262- lacZ(in pH-Pelican) | This study | N/A | P element transgenesis. Available on 1st, 2nd, and 3rd chromosomes |
Genetic reagent (D. melanogaster) | deiChO-262-placZ-wildtype(in placZattB) | This study | N/A | Inserted at attP2 |
Genetic reagent (D. melanogaster) | deiChO-262-placZ-Pros-site 1(in placZattB) | This study | N/A | Inserted at attP2 |
Genetic reagent (D. melanogaster) | deiChO-262-placZ-Pros-site 2(in placZattB) | This study | N/A | Inserted at attP2 |
Genetic reagent (D. melanogaster) | deiChO-262-placZ-Pros-site 3(in placZattB) | This study | N/A | Inserted at attP2 |
Genetic reagent (D. melanogaster) | deiChO-262-placZ-Pros-site 1 + 2 + 3(in placZattB) | This study | N/A | Inserted at attP2 |
Genetic reagent (D. melanogaster) | deiChO-262-placZ-Pax2-site 1(in placZattB) | This study | N/A | Inserted at attP2 |
Genetic reagent (D. melanogaster) | deiChO-262-placZattB Pax2-site 2 | This study | N/A | Inserted at attP2 |
Genetic reagent (D. melanogaster) | deiChO-262-placZ-Pax2-site 1 + 2(in placZattB) | This study | N/A | Inserted at attP2 |
Genetic reagent (D. melanogaster) | deiΔChO/(TM6) | This study | N/A | |
Genetic reagent (D. melanogaster) | M{nos-Cas9.P}ZH-2A | Bloomington Drosophila Stock Center | BDSC:54591 | |
Antibody | Anti Sv/D-Pax2 (rabbit polyclonal) | Johnson et al., 2011 | N/A | (1:10,000) |
Antibody | Anti-α85E-Tubulin (rabbit polyclonal) | Klein et al., 2010 | N/A | (1:200) |
Antibody | Anti α85E-Tubulin (mouse monoclonal) | Nachman et al., 2015 | N/A | (1:20) |
Antibody | Anti-Dei (rabbit polyclonal) | Egoz-Matia et al., 2011 | N/A | (1:50) |
Antibody | Anti-Spalt (rabbit polyclonal) | Halachmi et al., 2007 | N/A | (1:500) |
Antibody | Anti-NRG (rat polyclonal) | Banerjee et al., 2006 | N/A | (1:1000) |
Antibody | Anti-βGal (mouse monoclonal) | Promega | Z3781 | (1:1000) |
Antibody | Anti-Pros (mouse monoclonal) | The Developmental Studies Hybridoma Bank | MR1A | (1:20) |
Antibody | Anti Futsch (mouse monoclonal) | The Developmental Studies Hybridoma Bank | 22C10 | (1:20) |
Antibody | Anti-ELAV (rat monoclonal) | The Developmental Studies Hybridoma Bank | 7E8A10 | (1:50) |
Antibody | Anti-Eys (mouse monoclonal) | The Developmental Studies Hybridoma Bank | 21A6 | (1:20) |
Antibody | Anti-Repo (mouse monoclonal) | The Developmental Studies Hybridoma Bank | 8D12 | (1:10) |
Antibody | Anti Crb (mouse monoclonal) | The Developmental Studies Hybridoma Bank | Cq4 | (1:10) |
Antibody | Anti-Cpo (rabbit polyclonal) | Bellen et al., 1992 | N/A | (1:5000) |
Antibody | Anti-Sr (Chicken polyclonal) | This study | N/A | (1:20) |
Antibody | Cy3-conjugated goat anti-mouse | Jackson Laboratories, Bar-Harbor, Maine, USA | 115-165-166 | (1:100) |
Antibody | Cy3-conjugated goat anti-rabbit | Jackson Laboratories, Bar-Harbor, Maine, USA | 115-165-144 | (1:100) |
Antibody | Cy2-conjugated goat anti-rabbit | Jackson Laboratories, Bar-Harbor, Maine, USA | 111-225-144 | (1:100) |
Antibody | Alexa Fluor-647-conjugated goat anti-mouse | Jackson Laboratories, Bar-Harbor, Maine, USA | 115-605-166 | (1:100) |
Antibody | Alexa Fluor-647-conjugated goat anti-rabbit | Jackson Laboratories, Bar-Harbor, Maine, USA | 115-605-144 | (1:100) |
Antibody | Cy3-conjugated donkey anti-chicken IgY | Jackson Laboratories, Bar-Harbor, Maine, USA | 703-165-155 | (1:100) |
Antibody | Alexa Fluor-647-conjugated Donkey anti-Rat | Jackson Laboratories, Bar-Harbor, Maine, USA | 712-605-153 | (1:100) |
Commercial assay or kit | qScript cDNA Synthesis kit | Quanta BIOSCIENCES | 95047–100 | |
Commercial assay or kit | PrimeSTAR Max DNA polymerase | TAKARA | R045A | |
Chemical compound, drug | DAKO fluorescence mounting medium | Agilent Technologies, Santa Clara, CA, USA | S3023 | |
Chemical compound, drug | TRI Reagent | Sigma | T9424 | |
Software, algorithm | ImajeJ | National Institute of Health | SCR_003070 | |
Software, algorithm | FIMTrack tracking software | Risse et al., 2017 | https://www.uni-muenster.de/FRIA/en/FIM/ | |
Software, algorithm | MATLAB | Mathworks | SCR_001622 | |
Software, algorithm | Imaris | Bitplane | SCR_007370 |
Fly strains
Request a detailed protocolThe following mutant and reporter alleles of sv and pros were used: sv6/act-GFP (Kavaler et al., 1999), Dpax2D1-GFP (Johnson et al., 2011), pros17/TM6B, Tb1 (BDSC:5458). The following Gal4 drivers and UAS strains were used: deiChO-1353-GFP,deiattachment-RFP;en-Gal4 (Halachmi et al., 2016), deiKO-mCherry (Hassan et al., 2018), en-Gal4 (Brand and Perrimon, 1993), P{UAS-3xFLAG-pros.S}14 c, y1 w*; Pin1/CyO (BDSC:32245). UAS-sv-RNAi (VDRC:107343), UAS-sv (Kavaler et al., 1999), UAS-D-α-Catenin-GFP (Oda and Tsukita, 1999).
The deiChO-Gal4 driver was constructed by cloning the deiChO-1353 regulatory module described in Nachman et al., 2015 into the pChs-gal4 vector which was then used for the generation of transgenic fly strains (insertions are available on the X, 2nd and 3rd chromosome). The deiChO–262-lacZ strain was generated as previously described in Nachman et al., 2015. For mutational analysis of the putative binding sites, wild-type and mutated deiChO-262 fragments were synthesized by GenScript (USA) and cloned into the reporter constructs placZattB. Plasmids were integrated into the attP2 landing site by BestGene Inc (Chino Hills CA, USA). (Supplementary file 2 lists all the transgenes used in this study, vectors used, landing sites and the sequence of the inserted mutations). The deiΔChO allele was generated by GenetiVision (Houston TX, USA) via multiplex targeting with two sgRNAs: 5’GCACTTGTTTGCGTTTACATTAC3’ and 5’GGCGAGAAGTATTCCCTGCG3’; creating a defined deletion of 307 bps spanning the deiChO-262 fragment. The presence of the desired deletion was verified by sequencing. To verify that this deletion does not affect splicing or other structural features of the transcript, cDNA was synthesized and sequenced from the homozygous deiΔChO flies and control flies (the M{nos-Cas9.P}ZH-2A strain to which the injection was done). Total RNA was isolated from 10 adult flies using TRI Reagent (Sigma #T9424) according to the protocol described by Green et al. (Cold Spring Harb Protoc; doi:10.1101/pdb.prot101675). One mg of total RNA was used to generate cDNA using qScript cDNA Synthesis kit, according to the manufacturer protocol (Quanta BIOSCIENCE). PCR amplification was performed on 100 ng of cDNA using PrimeSTAR Max DNA polymerase (TAKARA #R045A), a forward primer from exon 1: TGCCAAATTTATGCATGAGC and reverse primer from exon 2: GCTTCTGTCGCAGGGAATAC.
Embryo staining and image analysis
Request a detailed protocolImmunostaining of whole-mount embryos was performed using standard techniques. The following primary antibodies used in this study were: Rabbit anti Sv/D-Pax2 (1:10,000) (Johnson et al., 2011), Rabbit anti α85E-Tubulin (1:200) (Klein et al., 2010), Mouse anti α85E-Tubulin (1:20) (Nachman et al., 2015), Rabbit anti Dei (1:50) (Egoz-Matia et al., 2011), Rabbit anti Spalt (1:500) (Halachmi et al., 2007), Rat anti NRG (1:1000) (Banerjee et al., 2006), anti-Cpo (1:5000) (Bellen et al., 1992), and Mouse anti-βGal (1:1000, Promega). The following antibodies were obtained from the Developmental Studies Hybridoma Bank: Mouse anti Pros (MR1A, 1:20), Mouse anti Futsch (22C10, 1:20), Rat anti-ELAV (7E8A10, 1:50), Mouse anti-Eys (21A6, 1:20), Mouse anti-Repo (8D12, 1:10), Mouse anti Crb (Cq4, 1:10). Chicken anti-Sr (1:20) was made against amino acids 707–1180 of the Sr protein fused to GST in the pGEX-KG expression vector. The ~80 kDa fusion protein was purified on Glutathione-agarose beads followed by elution with reduced glutathione. Antibodies against the GST-Sr fusion protein were produced in Chickens by Dr. Enav Bar-Shira (Department of Animal Sciences, Robert H. Smith Faculty of Agriculture Food and Environment, The Hebrew University of Jerusalem, Rehovot, Israel). The IgY antibody fraction was isolated from the egg yolk and cleaned on Glutathione-agarose beads to reduce background of anti-GST antibodies. Secondary antibodies for fluorescent staining were Cy3, Cy2, Cy5 or Alexa Fluor-647-conjugated anti-Mouse/Rabbit/Rat/Chicken/Guinea pig (1:100, Jackson Laboratories, Bar-Harbor, Maine, USA). Stained embryos were mounted in DAKO fluorescence mounting medium (Agilent Technologies, Santa Clara, CA, USA) and viewed using confocal microscopy (Axioskop and LSM 510, Zeiss).
For the analysis of reporter gene expression, images were analyzed using ImageJ software (http://rsb.info.nih.gov/ij/) as previously described (Preger-Ben Noon et al., 2018). Briefly, maximum projections of confocal stacks were assembled, and background was subtracted using a 50-pixel rolling-ball radius. Then, we manually segmented visible nuclei of cap and scolopale cells from LCh5 of abdominal segments A2 and measured the fluorescence mean intensities of each nucleus. Statistical analyses and graphing were performed using GraphPad Prism version 8, GraphPad Software, La Jolla California USA, https://www.graphpad.com/.
X-Gal staining
Request a detailed protocolStaining was done on staged pupae collected 40 hr after pupal formation. Pupae were removed from the pupal case and fixed at room temprature for 15 min in 4% formaldehyde in PBS. Following two washes in PBT (PBS + 0.1% Triton X-100), the pupae were incubated for five minutes in X-Gal staining buffer (without X-Gal) (5 mM K4[Fe+2(CN)6], 5 mM K3[Fe+2(CN)6]) in PBT and then incubated in staining buffer containing 1 mg/ml X-Gal for 4 hr at 37°C.
Yeast one-hybrid analysis
Request a detailed protocolYeast one-hybrid screening was performed by Hybrigenics Services, S.A.S., Evry, France (http://www.hybrigenics-services.com). The sequence of deiChO-389 was PCR-amplified and cloned into the integrative vector pB301 (pAbAi, Clontech Laboratories, Inc). The construct was checked by sequencing the entire insert and transformed into the YM955 yeast strain to integrate the DNA bait into the yeast genome. Screening was performed against a random-primed Drosophila Whole Embryo cDNA library constructed into pP6 that derives from the original pGADGH (Bartel, 1993) plasmid. 124 million clones (12-fold the complexity of the library) were screened using a mating approach with YHGX13 (Y187 ade2-101::loxP-kanMX-loxP, matα) and the dei-containing yeast (mata) strain as previously described (Fromont-Racine et al., 1997). 146 His + colonies were selected on a medium lacking uracil, leucine and supplemented with 400 ng/ml Aureobasidin A. The prey fragments of the positive clones were amplified by PCR and sequenced at their 5’ and 3’ junctions. The resulting sequences were used to identify the corresponding interacting proteins in the GenBank database (NCBI) using a fully automated procedure. A confidence score (PBS, for Predicted Biological Score) was attributed to each interaction as previously described (Formstecher et al., 2005; Rain et al., 2001; Wojcik et al., 2002).
Motif search analysis
Request a detailed protocolThe D-Pax2/Sv site 2 was predicted by JASPAR (Mathelier et al., 2016). The PWM for Pros was generated using the sequences selected in TDA by Hassan et al., 1997 and the MEME suite (Bailey et al., 2015). The MEME suite was used to search for the Pros motif in deiChO-262 and to compare the experimentally identified binding sites to the D-Pax2/Sv and Pros PWMs.
Protein purification
Request a detailed protocolD-Pax2-HD and Pros-S-HD expression plasmids were a kind gift from Brian Gebelein and Tiffany Cook (Cook et al., 2003; Li-Kroeger et al., 2012). Proteins were purified from E. coli (BL21) as described previously (Uhl et al., 2010) with the following modifications. Protein expression was induced at 37 °C using 0.1 mM IPTG for 4 hr (Pros-S/L-HD) or 0.4 mM IPTG for 4 hr (D-Pax2-HD).
Pros-S-HD: After induction, bacterial pellet was resuspended in PBS supplemented with complete protease inhibitor mix (Roche) and lysed on ice using sonication (10 cycles of 30 s on/off). GST-tagged Pros-S-HD in soluble fraction was purified using Glutathione-Agarose beads (Sigma). The bound proteins were eluted in elution buffer (50 mM Tris, pH 8, 10 mM reduced Glutathione).
D-Pax2-HD: The bacterial pellet was resuspended in 20 mM Tris, pH 7.5 supplemented with protease inhibitor. His-tagged proteins in soluble fraction were purified using cOmplete His-Tag Purification Columns (Roche). The columns were washed with 10 column volumes of wash buffer 1 (20 mM Tris, pH7.5, 300 mM NaCl, 50 mM NaH2PO4 pH 8.0), 2 column volumes of wash buffer 2 (20 mM Tris, pH7.5, 300 mM NaCl, 5 mM DTT, 10 mM Imidazole), and bound proteins were eluted in the same buffer supplemented with 250 mM Imidazole.
All samples were dialyzed against 500 ml of dialysis buffer (20 mM HEPES, pH 7.9, 200 mM NaCl, 10% Glycerol, 2 mM MgCl) for 18 hr at 4 °C. Protein concentrations were measured with NanoDrop and confirmed by SDS-PAGE and Coomassie blue analysis.
Electromobility shift assay (EMSA)
Request a detailed protocolDNA probes were generated by annealing 5’ IRDye700 labeled forward oligonucleotides with unlabeled reverse oligonucleotides (Integrated DNA Technologies) to a final concentration of 5 µM in PNK buffer (New England Biolabs). One hundred femtomoles of labeled IRDye700 probes were used in a 20 µl binding reaction containing 10 mM Tris, pH 7.5; 50 mM NaCl; 1 mM MgCl2; 4% glycerol; 0.5 mM DTT; 0.5 mM EDTA; 50 µg/ml poly(dI–dC); 200 µg/ml of BSA and purified proteins (see Supplementary file 3 for amount of each protein used). The binding reactions were incubated at room temperature for 30 min, and run on a native 4% polyacrylamide gel for 1.5 hr at 180 V. For competition assays, the appropriate amount of cold competitor was added with the IRDye700-labeled probe prior to the incubation. The polyacrylamide gel cassettes were imaged using an Odyssey Infrared Imaging System and image analysis was performed using ImageQuant 5.1 software. All experiments were performed at least three times.
Locomotion assays
Request a detailed protocolLarvae used in the locomotion assays were collected from 8 to 12 hr egg collections that aged at 24°C until reaching the wandering 3rd instar stage (115–140 hr). 25–30 larvae of each genotype were individually transferred to a fresh 2% agar 10 cm plate, prewarmed to 24 °C. Larvae were let to adjust for 30 s prior to 2-min recording at a rate of 30 frames per second. The wild-type larvae often exited the filmed arena before the completion of the full 2 min recording time. Larval locomotion was recorded using a Dino-Lite digital microscope placed above the plate. We used VideoPad software to convert Dino-Lite files into Tiff files. ImageJ and FIMTrack (Risse et al., 2017) tracking software were used for following larval (center of mass) movements and body angle.
Data availability
All data generated or analysed during this study are included in the manuscript and supporting files. Source data files have been provided.
References
-
Axonal ensheathment and septate junction formation in the peripheral nervous system of DrosophilaThe Journal of Neuroscience 26:3319–3329.https://doi.org/10.1523/JNEUROSCI.5383-05.2006
-
BookUsing the two-hybrid system to detect protein-protein interactionsIn: Hartley DA, editors. Cellular Interactions in Development: A Practical Approach. Oxford: Oxford University Press. pp. 153–179.
-
The Soft Touch: Low-Affinity Transcription Factor Binding Sites in Development and EvolutionCurrent Topics in Developmental Biology 117:455–469.https://doi.org/10.1016/bs.ctdb.2015.11.018
-
Protein interaction mapping: a Drosophila case studyGenome Research 15:376–384.https://doi.org/10.1101/gr.2659105
-
Mechanisms of asymmetric cell division: flies and worms pave the wayNature Reviews. Molecular Cell Biology 9:355–366.https://doi.org/10.1038/nrm2388
-
Additional sex combs affects antennal development by means of spatially restricted repression of Antp and wgDevelopmental Dynamics 236:2118–2130.https://doi.org/10.1002/dvdy.21239
-
An RNAi Screen Identifies New Genes Required for Normal Morphogenesis of Larval Chordotonal OrgansG3: Genes, Genomes, Genetics 8:1871–1884.https://doi.org/10.1534/g3.118.200218
-
Low-Affinity Binding Sites and the Transcription Factor Specificity Paradox in EukaryotesAnnual Review of Cell and Developmental Biology 35:357–379.https://doi.org/10.1146/annurev-cellbio-100617-062719
-
Conference2017 IEEE International Conference on Computer Vision WorkshopVisual Tracking of Small Animals in Cluttered Natural Environments Using a Freely Moving Camera.https://doi.org/10.1109/ICCVW.2017.335
-
Asymmetric cell division in the Drosophila bristle lineage: from the polarization of sensory organ precursor cells to Notch-mediated binary fate decisionWiley Interdisciplinary Reviews. Developmental Biology 4:299–309.https://doi.org/10.1002/wdev.175
-
Dedifferentiation of neurons precedes tumor formation in Lola mutantsDevelopmental Cell 28:685–696.https://doi.org/10.1016/j.devcel.2014.01.030
-
Prediction, assessment and validation of protein interaction maps in bacteriaJournal of Molecular Biology 323:763–770.https://doi.org/10.1016/s0022-2836(02)01009-4
Article and author information
Author details
Funding
Israel Science Foundation (Adi Salzberg 674/17)
- Adi Salzberg
The funders had no role in study design, data collection and interpretation, or the decision to submit the work for publication.
Acknowledgements
We wish to thank Joshua Kavaler, Tiffany Cook, Markus Noll, Anna Jazwinska, Manzur Bhat, the Bloomington Stock Center and the Developmental Studies Hybridoma Bank at the University of Iowa for sending us antibodies and fly strains. We are very grateful to Enav Bar-Shira for her kind help in generating the chicken anti-Sr antibodies and to Abeer Hassan for helping us with the analysis of the locomotion assays’ data. This work was supported by a grant (No. 674/17) to AS from The Israel Science Foundation.
Copyright
© 2021, Avetisyan et al.
This article is distributed under the terms of the Creative Commons Attribution License, which permits unrestricted use and redistribution provided that the original author and source are credited.
Metrics
-
- 644
- views
-
- 59
- downloads
-
- 1
- citation
Views, downloads and citations are aggregated across all versions of this paper published by eLife.
Citations by DOI
-
- 1
- citation for umbrella DOI https://doi.org/10.7554/eLife.70833