Structural, mechanistic, and physiological insights into phospholipase A-mediated membrane phospholipid degradation in Pseudomonas aeruginosa
Figures
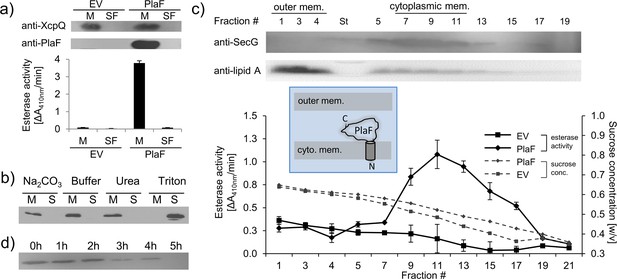
Subcellular localization of PlaF.
(a) PlaF is a membrane protein of Pseudomonas aeruginosa. The membrane (M) and soluble fractions (SFs) of cell extracts from P. aeruginosa p-plaF, and the empty vector control strain (EV) were separated, analyzed by immunodetection with anti-His6-tag antibodies, and by esterase activity assay. The membrane protein marker P. aeruginosa XcpQ was detected with anti-XcpQ antibodies. (b) PlaF is an integral membrane protein of P. aeruginosa. The crude membranes of P. aeruginosa p-plaF were treated with sodium carbonate, urea, Triton X-100, or MES buffer control followed by ultracentrifugation (S, supernatant; M, membrane proteins). PlaF was detected as in (a). (c) PlaF is a cytoplasmic-membrane protein of P. aeruginosa. The membrane fractions of P. aeruginosa p-plaF and the EV strains were isolated and separated by ultracentrifugation in a sucrose density gradient. The esterase activity was assayed as in (a). P. aeruginosa SecG, and outer membrane lipid A were used as markers for cytoplasmic, and outer membranes, and detected by Western blotting using anti-SecG, and anti-Lipid A antibodies, respectively. Inlet: A model of PlaF cellular localization. All values are mean ± standard deviation (S.D.) of three independent experiments measured in triplicates. (d) The catalytic domain of PlaF is exposed to the periplasm. P. aeruginosa p-plaF cells with permeabilized outer membrane were treated with trypsin for the indicated periods, and PlaF was detected as described in (a).
-
Figure 1—source data 1
Uncropped Western blot shown in Figure 1a.
- https://cdn.elifesciences.org/articles/72824/elife-72824-fig1-data1-v2.pdf
-
Figure 1—source data 2
Uncropped Western blot shown in Figure 1b.
- https://cdn.elifesciences.org/articles/72824/elife-72824-fig1-data2-v2.pdf
-
Figure 1—source data 3
Uncropped Western blot shown in Figure 1c.
- https://cdn.elifesciences.org/articles/72824/elife-72824-fig1-data3-v2.pdf
-
Figure 1—source data 4
Uncropped Western blot shown in Figure 1d.
- https://cdn.elifesciences.org/articles/72824/elife-72824-fig1-data4-v2.pdf
-
Figure 1—source data 5
Excel file with data used to make Figure 1a.
- https://cdn.elifesciences.org/articles/72824/elife-72824-fig1-data5-v2.xlsx
-
Figure 1—source data 6
Excel file with data used to make Figure 1c.
- https://cdn.elifesciences.org/articles/72824/elife-72824-fig1-data6-v2.xlsx
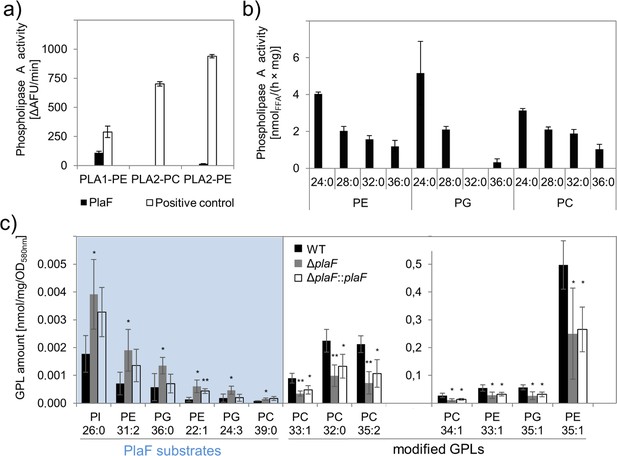
Phospholipolytic activity profiling of PlaF.
(a) PlaF is a phospholipase A1. Enzyme activities of PlaF were measured fluorimetrically using artificial PLA1, and PLA2 substrates containing either ethanolamine (PE) or choline (PC) head groups. The control enzymes were PLA1 of Thermomyces lanuginosus, and PLA2 of Naja mocambique. Results are means±S.D. of three independent measurements performed with at least three samples. (b) PlaF releases FAs from naturally occurring bacterial GPLs. PLA activity of PlaF was measured by quantification of released FAs after incubation of PE, PG, and PC substrates containing FAs with different chain lengths (C12–C18). (c) PlaF changes GPL composition of Pseudomonas aeruginosa cells. Crude lipids extracted from P. aeruginosa wild-type (WT), ΔplaF, and ΔplaF::plaF membranes were quantified by Q-TOF-MS/MS using an internal standard mixture of GPLs. PlaF substrates are elevated in ΔplaF and depleted in ΔplaF::plaF, while modified GPLs show inverse response than GPL substrates. The GPL amount (nmol) was normalized to mg of crude lipids, and optical density (Supplementary file 3). FA composition of GPL is depicted as XX:Y, where XX defines the number of carbon atoms, and Y defines the number of double bonds in FAs bound to GPL. Results are mean ± S.D. of four biological replicates of WT, ΔplaF, and three of the ΔplaF::plaF. T-test of normally distributed values, ** p<0.01, * p<0.05. FA, fatty acid; GPL, glycerophospholipid.
-
Figure 2—source data 1
Excel file with data used to make Figure 2a.
- https://cdn.elifesciences.org/articles/72824/elife-72824-fig2-data1-v2.xlsx
-
Figure 2—source data 2
Excel file with data used to make Figure 2b.
- https://cdn.elifesciences.org/articles/72824/elife-72824-fig2-data2-v2.xlsx
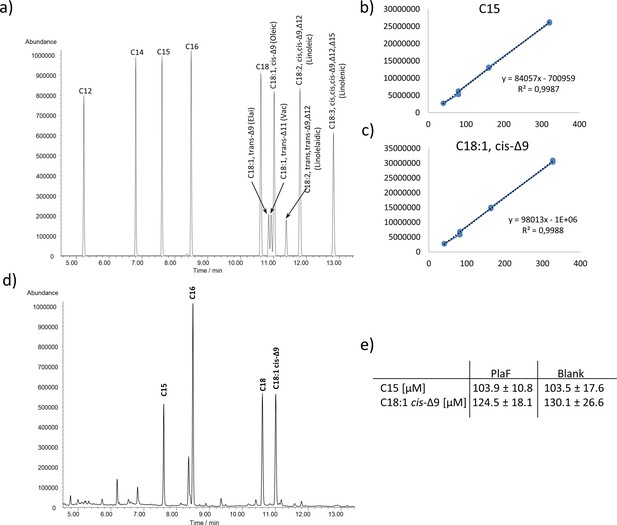
Determination of PLA activity of PlaF by GC-MS.
(a) Total ion current chromatogram of fatty acid (FA) methyl ester standards. Standard curves of C15 (b) and C18:1 cis-Δ9 (c) methyl esters. (d) FAs were extracted with organic solvent from samples in which PG-15:0-18:1 was incubated with PlaF. Silylated FAs were quantified by GC-MS. Palmitic and stearic acids detected in these samples were probably bound to PlaF used in the experiment. (e) FA amounts detected in PlaF and blank samples. Results are means ± standard deviations of two independent experiments. GC-MS, gas chromatography-mass spectrometric.
-
Figure 2—figure supplement 1—source data 1
Excel file with data used to make Figure 2—figure supplement 1.
- https://cdn.elifesciences.org/articles/72824/elife-72824-fig2-figsupp1-data1-v2.xlsx
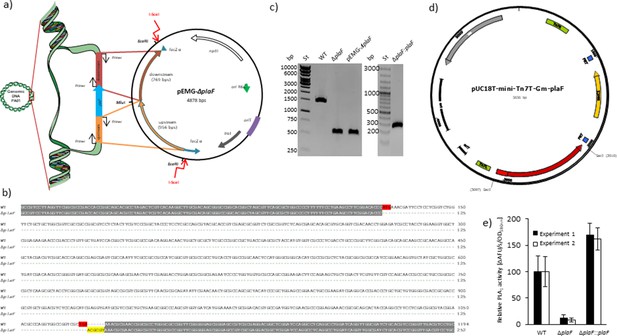
Generation of Pseudomonas aeruginosa ΔplaF deletion mutant and P. aeruginosa ΔplaF::plaF complemented strain.
(a) Generation of the mutagenesis vector pEMG-ΔplaF and homologous recombinant with a chromosome of P. aeruginosa PAO1 (Martínez-García and de Lorenzo, 2011). Upstream and downstream regions of the plaF gene were amplified by standard PCR using Phusion DNA polymerase, a genomic DNA of P. aeruginosa PAO1 as a template, and primer pairs 5′-ATATATGAATTCTCTGCTCGGCGCGAAACGCAGCGP-3′/5′-ATATATACGCGTGGGTGTCCGAAGGCTTCAGGAAAAAAGGGGC-3′ and 5′-ATATATACGCGTAAACGCGAACCGGCGCCTGGG-3′/5′-CTGGATGAATTCTGGCCTGGACACCGACAAGGAAGTGATCAAGG-3′, respectively. DNA fragments upstream and downstream of plaF gene were cloned into the pEMG vector by ligation of DNA fragments hydrolyzed with EcoRI restriction endonuclease. The white arrow indicates the neomycin phosphotransferase II gene (nptII) necessary for plasmid selection in the presence of kanamycin antibiotic. DNA recognition sequence for I-SceI restriction endonuclease is indicated with the red arrows. (b) Sequence alignment of DNA products of P. aeruginosa ΔplaF and the wild-type (WT) strain obtained by PCR as described in Figure 2—figure supplement 2. The start and stop codons of plaF gene are indicated in red, identical nucleotides are indicated in gray, the recognition site for MluI restriction endonuclease inserted on the chromosome using the mutagenesis vector pEMG-ΔplaF in yellow. The company Eurofins Genomics (Ebersberg, Germany) performed DNA sequencing. (c) Verification of P. aeruginosa ΔplaF and ΔplaF::plaF strains by PCR. Standard PCR was performed using Phusion DNA polymerase and the primers (5′-AAGGTCGCCGCCAGGAATTTCCG-3′/5′-CCGCCTGCGTGCCGACTACAAGG-3′) that bind to the up- and downstream regions of plaF gene. As PCR templates were used genomic DNA of P. aeruginosa PAO1 (WT), pEMG-ΔplaF plasmid and DNA obtained from the colony of P. aeruginosa ΔplaF or ΔplaF::ΔplaF scratched from the LB agar plate and suspended in water. The expected sizes of DNA fragments were 1494, 552, 552, and 322 bp for WT, ΔplaF, and pEMG-ΔplaF, and ΔplaF::plaF, respectively. DNA products were analyzed by electrophoresis on agarose gel (1% w/v). The sizes of standard DNA fragments are indicated on the left. (d) Generation of pUC18T-mini-Tn7T-Gm-plaF plasmid for recombination of plaF gene containing 128 bp upstream region of plaF with a chromosome of P. aeruginosa ΔplaF. DNA fragment containing upstream region and plaF gene was amplified using primer pair 5′-AATAGAGCTCACCGCCGTCCTTAGGTTC-3′/5′-AATAGAGCTCCGTTTTCAGCGACCGGC-3′ from the genomic DNA of P. aeruginosa PAO1. Both primers contained the restriction site SacI for cloning into the pUC18T-mini-Tn7T-Gm (gifts from Herbert Schweizer, Addgene plasmids #63121, #64968, and #64946) which consists of β-lactamase resistance gene (bla), ColE1 origin of replication (ori), the origin of conjugative transfer (oriT), left and right ends of Tn7 (Tn7L and Tn7R), Flp recombinase target (FRT) and gentamycin acetyltransferase gene (aacC1). (e) Phospholipase A1 activity of P. aeruginosa ΔplaF and ΔplaF::plaF. P. aeruginosa PAO1, ΔplaF, and ΔplaF::plaF strains were grown overnight in LB medium at 37°C, and cells were harvested at O.D.580nm~1 by centrifugation. Cells were suspended in Tris-HCl buffer (100 mM, pH 8) to equal cell count, and enzyme activities of these samples were measured using PLA1 assays with N-((6-(2,4-DNP)amino)hexanoyl)–1-(BODIPYFL C5)–2-hexyl-sn-glycero-3-phosphoethanolamine substrate. Activities are normalized on the activity of the WT which was set as 100%. The results are mean ± S.D. of two experiments with three biological replicates each measured three times. LB, lysogeny broth.
-
Figure 2—figure supplement 2—source data 1
Excel file with data used to make Figure 2—figure supplement 2.
- https://cdn.elifesciences.org/articles/72824/elife-72824-fig2-figsupp2-data1-v2.xlsx
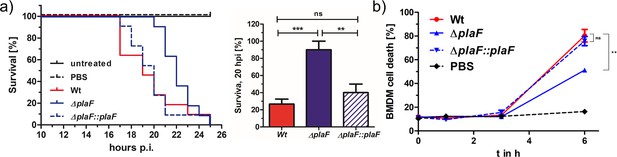
PlaF is a novel virulence factor of Pseudomonas aeruginosa PAO1.
(a) Left: P. aeruginosa ΔplaF strain is less virulent than the respective wild-type (WT) strain in a Galleria mellonella larvae virulence assay. Kaplan-Meier plot of representative data of at least two experiments with 10 larvae per group. PBS treated and untreated larvae served as infection and viability controls, respectively. Right: Statistical analysis of the survival at the 20 hr using three independent experiments with 10 larvae each. (b) P. aeruginosa ΔplaF strain is less cytotoxic to bone marrow-derived macrophages (BMDMs) than the WT strain in cell culture. The BMDM cells (5×105) were infected with 5×105 bacteria in a 24-well plate, and lactate dehydrogenase activity in supernatants was determined as a measure of BMDM death. The ΔplaF phenotype could be complemented with P. aeruginosa ΔplaF::plaF. PBS or Triton-X100 (1% v/v) treated cells served as viability or 100% killing controls, respectively. Results are the representative data of two independent experiments (n=10). One-way ANOVA analysis, *** p<0.001, ** p<0.01, ns, not significant; PBS, phosphate-buffered saline.
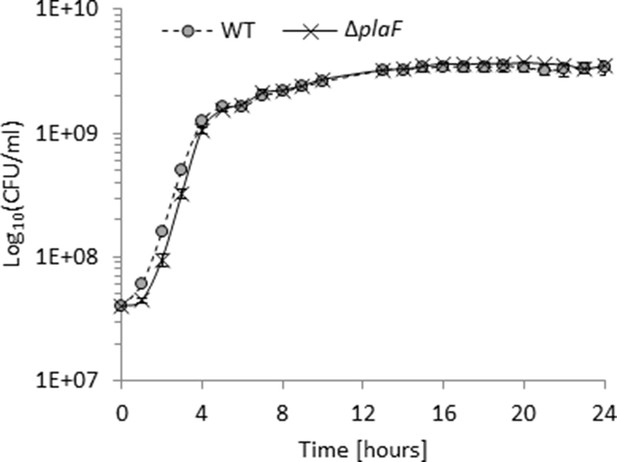
The growth of Pseudomonas aeruginosa PA01 and ΔplaF do not differ.
P. aeruginosa strains (n=3) were grown in LB medium in Erlenmeyer flasks at 37°C. LB, lysogeny broth.
-
Figure 3—figure supplement 1—source data 1
Excel file with data used to make Figure 3—figure supplement 1.
- https://cdn.elifesciences.org/articles/72824/elife-72824-fig3-figsupp1-data1-v2.xlsx
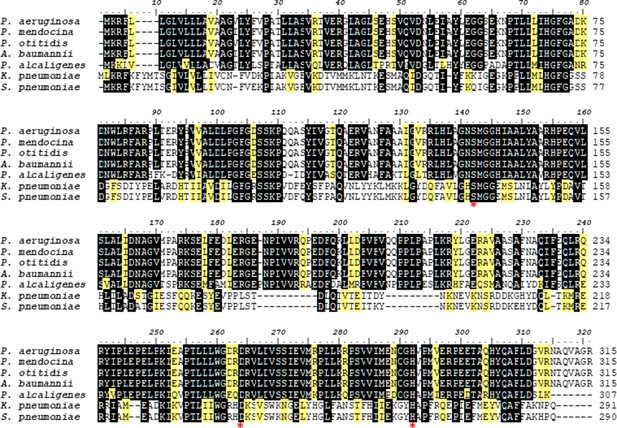
Sequence alignment of PlaF and its homologs.
Pseudomonas alcaligenes (Pal-PlaF; sequence ID: A0A142IQQ0; sequence identity 68%, coverage 97%), Pseudomonas mendocina (sequence ID: A0A291K7Z5; sequence identity 63%, coverage 100%), Pseudomonas otitidis (sequence ID: A0A1I0U5Q8; sequence identity 62%, coverage 100%), Acinetobacter baumannii (sequence ID: A0A1G5KTV2; sequence identity 99.7%, coverage 100%), Klebsiella pneumoniae (sequence ID: OON65700.1; sequence identity 27%, coverage 90%), and Streptococcus pneumoniae (sequence ID: CGG52681.1; sequence identity 28%, coverage 90%). Residues identical and similar in at least five sequences were shaded in black and yellow, respectively. The catalytic triad residues of PlaF are indicated with an asterisk. The figure was prepared using BioEdit software. (Skjevik et al., 2016).
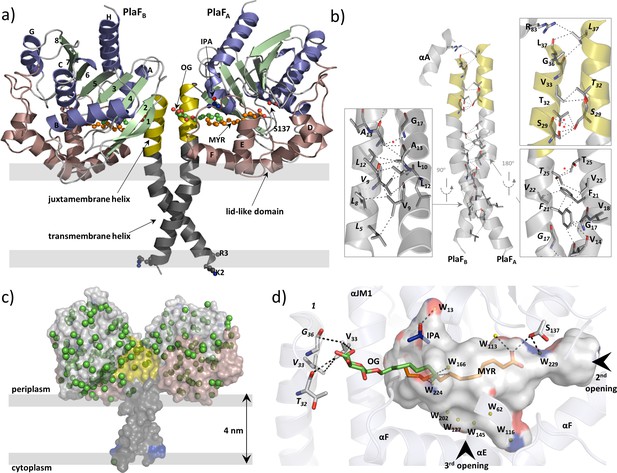
Overall structure of dimeric PlaF with bound endogenous FA ligands.
(a) A unique N-terminal helix comprising a putative transmembrane helix (αTM1, L5–L27, gray) flanked by charged residues (K2, R3) on one side and, on another side, the juxtamembrane helix (αJM1, A28–L37, yellow). αJM1 links the αTM1 with the catalytic domain, which consists of an α/β-hydrolase (blue, α-helices; green, β-strands, and gray, loops), and a lid-like domain (brown). Ligands bound in the active site cleft are shown as ball-and-sticks (oxygen, red; carbon of OG, MYR, and IPA, green, orange, and blue, respectively). Thick gray lines roughly depict the membrane borders. (b) Dimer interface. Interactions involving TM-JM helices are predominantly hydrophobic with four weak H-bonds (indicated by a red asterisk) detected mostly in the αJM1. R83 is the only residue outside of the JM-TM helix involved in interactions. Residues of the PlaFB molecule are indicated in italics. A detailed list of interactions is provided in Supplementary file 6. (c) A model suggesting the orientation of PlaF in the membrane. The water molecules are indicated as green spheres. The transparent surface of PlaF was colored as in (a). PlaF is rotated by 180° along the normal to the membrane compared with Figure 4. (d) Interaction network within the ligand-binding cleft of PlaFA. MYR is linked via H-bond with the catalytic S137, and via hydrophobic interactions with OG. The sugar moiety of OG from PlaFA forms H-bonds with V33 of PlaFA, which is interacting with V33 and G36 of PlaFB. The part of the cleft in the direction of the opening 3 is occupied by several water molecules (W, yellow spheres). The cleft accommodates one IPA molecule bound to the water. Arrows indicate two openings not visible in this orientation. The cleft was calculated using the Pymol software and colored by elements: carbon, gray; oxygen, red; nitrogen, blue. FA, fatty acid.
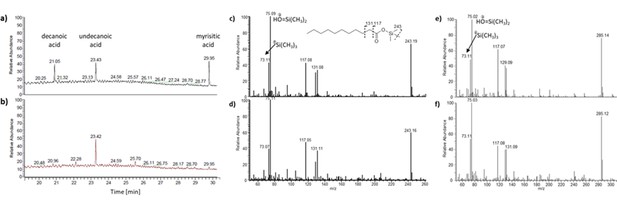
Identification of fatty acid (FA) ligands co-purified with PlaF.
FAs were extracted from purified PlaF samples with organic solvent followed by silylation and gas chromatographic separation with mass spectrometric detection (GC-MS). GC chromatograms of pure C10, C11, and C14 fatty acids as standards (a) were compared to PlaF extracts (b). Mass spectrometric analysis of compounds with retention times 23.43/23.42 min and 29.95 min for FA standards (c, e) and PlaF extracts (d, f) revealed the presence of undecanoic and myristic acid trimethylsilyl esters, respectively. The chemical structure of undecanoic and myristic acid trimethylsilyl ester and characteristic fragments (Diekman et al., 2002) (molecular weights in Da are indicated above) identified in mass spectra are shown.
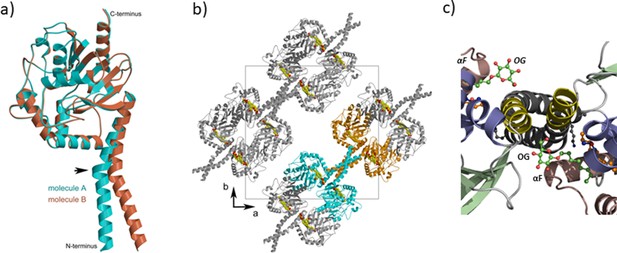
Comparison of PlaF monomers.
(a) Superposition of PlaFA (cyan) and PlaFB (orange). The arrow indicates the kink in TM-JM helix. (b) Crystal packing of PlaF showing a four-helix bundle formed by TM-JM helices. For clarity, two dimers are shown in cyan and orange colors. The ligands present are shown as filled circles and colored according to the element (carbon yellow and oxygen red). The unit cell (black square) and the a-b axes are labeled. (c) Coiled-coil structure of the TM-JM. Enlarged section of PlaF viewed from the periplasmic side showing the coiled-coil organization of the TM-JM helix and the OG ligands located between JM and the loop preceding αF in both PlaF monomers. Elements of the PlaFB molecule are indicated in italics.
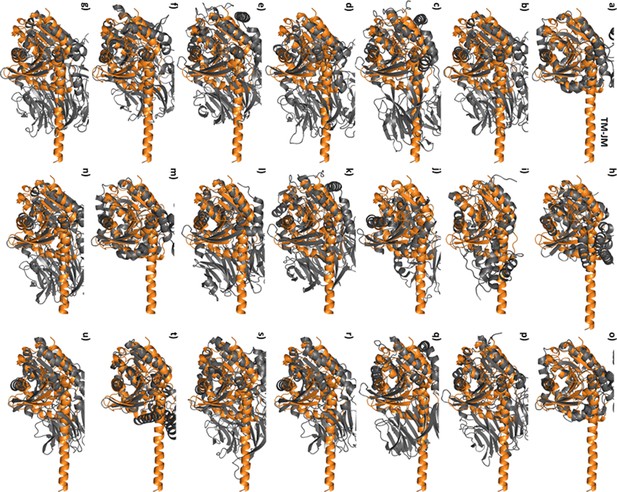
TM-JM helix of PlaF is not detected among PlaF structural homologs.
Pairwise structural alignment of PlaFB (colored orange) with 1cqz (a), 1orw (b), 1yr2 (c), 1z68 (d), 2bkl (e), 2ecf (f), 2bgc (g), 2jbw (h), 2roq (i), 3mga (j), 3mun (k), 3o4j (l), 4hai (m), 4n8e (n), 5alj (o), 5l8s (p), 5t88 (q), 5yzm (r), 6eop (s), 6hxa (t), and 6igp (u). PlaF structural homologs (colored gray) containing conserved sequence in TM-JM helix region of PlaF (yellow highlighted in Supplementary file 6) and in catalytic domain as identified by Dali server.
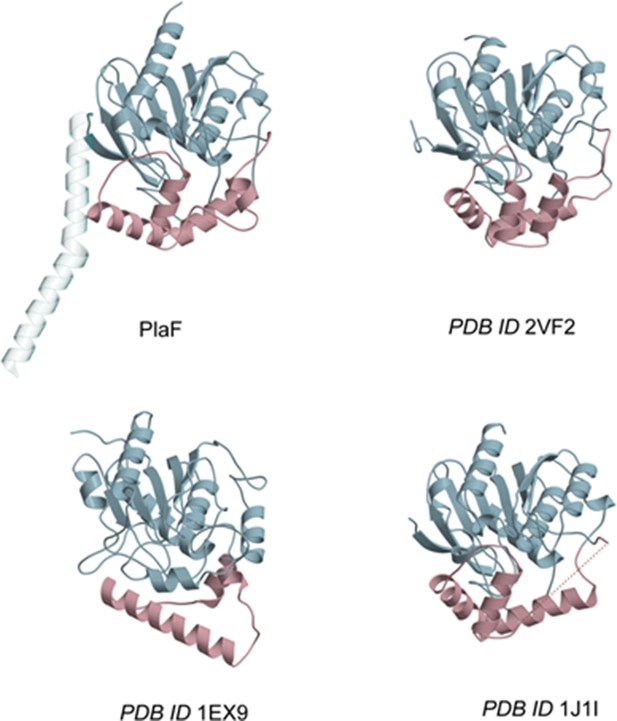
The lid-like domains of PlaF and its homologs.
Lid-like domains, shown in pink, vary considerably among PlaF and hydrolytic enzymes (hydrolase HsaD from Mycobacterium tuberculosis, PDB ID 2VF2 Lack et al., 2010; lipase LipA from Pseudomonas aeruginosa, PDB ID 1E × 9 Nardini et al., 2000; and hydrolase CarC from Janthinobacterium sp. strain J3, PDB ID 1J1I Habe et al., 2003) with structurally conserved α/β-hydrolase domains (light blue).
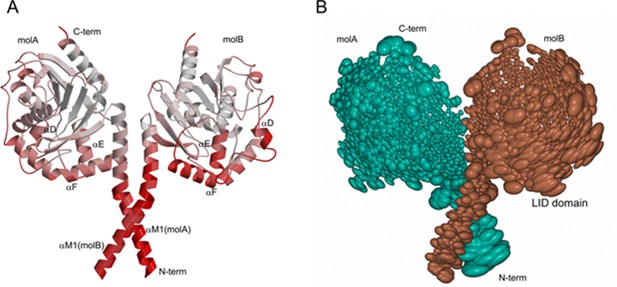
PlaF structure reveals differently ordered subdomains.
(a) Ribbon representation of the dimer structure colored according to B-factor. The lid domain and the N-terminal helices show significantly higher B-factors (color spectrum white—low B-factor, to red—high B-factor). The average B-factors of the αTM1 helix and the lid domain in PlaF dimer are ~74 and ~55 Å2, respectively. (b) Thermal ellipsoid representation of the dimer scaled by the B-factors combined with TLS (translation-libration-screw-rotation model) displays comparatively higher B-factors in the lid domain in molecule B and the αTM1 helix in molecule A. The figure was prepared using CCP4mg (Winn et al., 2011).
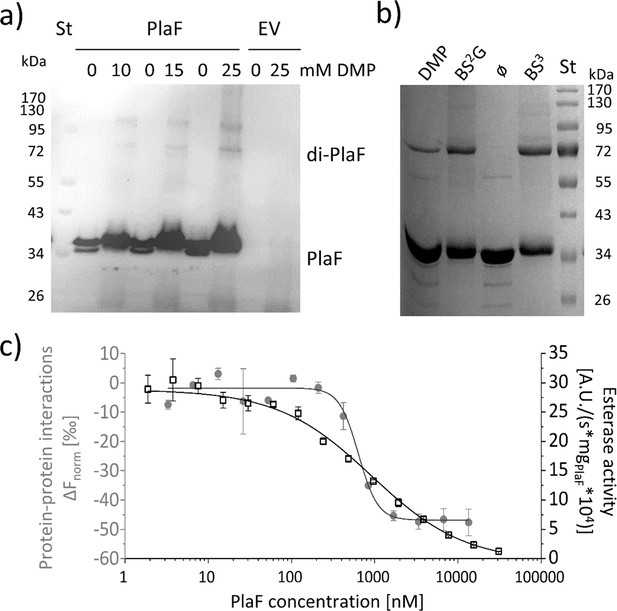
PlaF oligomeric states and their enzymatic activity.
(a) PlaF forms dimers in cell membranes.In vivo cross-linking experiments were performed by incubating Pseudomonas aeruginosa p-plaF or the empty vector control (EV) cells with different concentrations of DMP cross-linker followed by immunodetection of PlaF with anti-PlaF antiserum. (b) In vitro cross-linking of purified PlaF. Purified PlaF was incubated with DMP, BS2G, and BS3 cross-linking reagents or buffer control (ø) for 90 min, and the samples were analyzed by SDS-PAGE. Molecular weights of protein standard in kDa are indicated. (c) PlaF homodimerization, and activity are concentration-dependent. Protein-protein interactions of purified PlaF were monitored by measuring the changes in thermophoresis (ΔFnorm, gray circles) using the MST method. The MST results are mean ± S.D. of two independent experiments with PlaF purified with OG. Esterase activity (black squares) of PlaF was measured in three independent experiments using 4-methylumbelliferyl palmitate substrate. Dissociation (KD) and activation (Kact) constants were calculated using a logistic fit of sigmoidal curves.
-
Figure 5—source data 1
Uncropped Western blot shown in Figure 5a.
- https://cdn.elifesciences.org/articles/72824/elife-72824-fig5-data1-v2.pdf
-
Figure 5—source data 2
Uncropped SDS-PAGE shown in Figure 5b.
- https://cdn.elifesciences.org/articles/72824/elife-72824-fig5-data2-v2.pdf
-
Figure 5—source data 3
Origin file with data used to make Figure 5c.
- https://cdn.elifesciences.org/articles/72824/elife-72824-fig5-data3-v2.zip
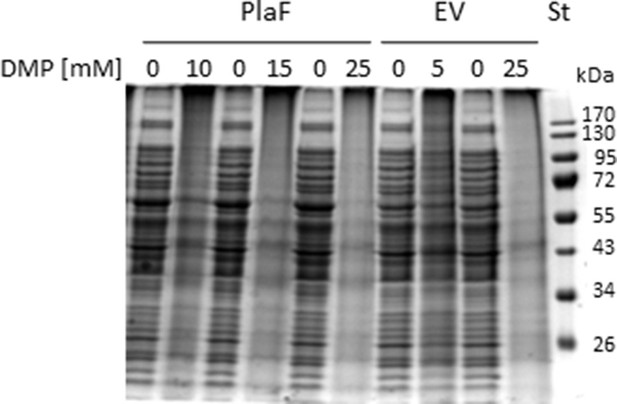
In vivo crosslinking of PlaF.
The SDS-PAGE analysis of in vivo cross-linking samples of Pseudomonas aeruginosa strain carrying pBBR1mcs-3 (empty vector control, EV) or p-plaF obtained in the experiment shown in Figure 5a. Molecular weights of protein standard (St) in kDa are indicated.
-
Figure 5—figure supplement 1—source data 1
Uncropped SDS-PAGE shown in Figure 5—figure supplement 1.
- https://cdn.elifesciences.org/articles/72824/elife-72824-fig5-figsupp1-data1-v2.xlsx
-
Figure 5—figure supplement 1—source data 2
Original file of the SDS-PAGE shown in Figure 5—figure supplement 1.
- https://cdn.elifesciences.org/articles/72824/elife-72824-fig5-figsupp1-data2-v2.pdf
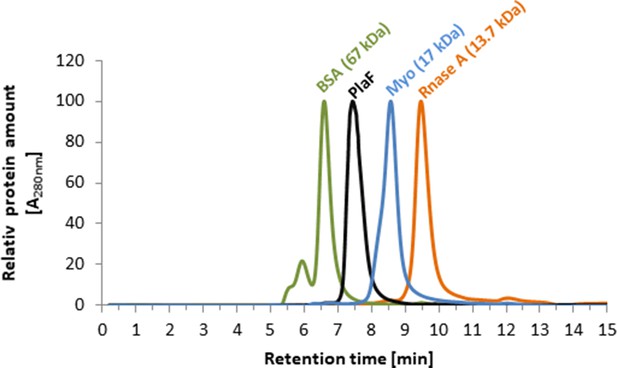
Size exclusion chromatography of PlaF showed a monomer.
PlaF (10 µl, 2 mg/ml) purified with OG and standard proteins, bovine serum albumin (BSA), equine myoglobin (Myo), and bovine ribonuclease A (RNase A) were separately analyzed using Biosep-SEC-S2000 column. Proteins were detected by measuring absorbance at a wavelength of 280 nm, and values were normalized to the maximal A280nm of each protein.
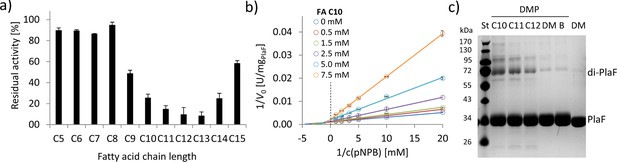
FAs exert an inhibitory effect on PlaF and trigger dimerization.
(a) Inhibition of PlaF with FAs. Esterase activity of PlaF was measured in the presence of 7.5 mM FA (C5–C15); an untreated PlaF sample was set as 100%. The results are mean ± S.D. of three experiments with three samples each. (b) Kinetic studies with FA C10 show evidence of mixed-inhibition. Double-reciprocal plots of initial reaction velocities measured with the p-NPB substrate and FA C10 inhibitor at concentrations in a range of 0–7.5 mM. (c) The effect of FAs on PlaF dimerization. PlaF samples incubated with FAs (C10–C12), dimethyl sulfoxide (DM, DMSO used to dissolve FAs), and purification buffer (B, dilution control) were cross-linked with dimethyl pimelimidate (DMP). FA, fatty acid.
-
Figure 6—source data 1
Excel file with data used to make Figure 6a.
- https://cdn.elifesciences.org/articles/72824/elife-72824-fig6-data1-v2.xlsx
-
Figure 6—source data 2
Uncropped SDS-PAGE shown in Figure 6c.
- https://cdn.elifesciences.org/articles/72824/elife-72824-fig6-data2-v2.xlsx
-
Figure 6—source data 3
Excel file with data used to make Figure 6b.
- https://cdn.elifesciences.org/articles/72824/elife-72824-fig6-data3-v2.pdf
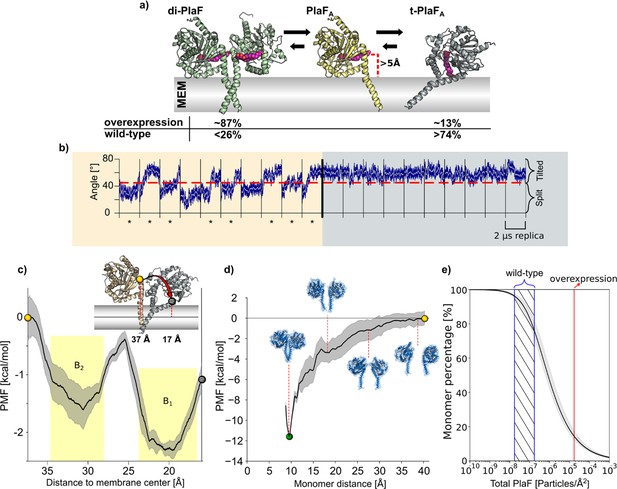
Molecular dynamics (MD) simulations and PMF computations of PlaF in the lipid bilayer.
(a) Structures used for MD simulations. di-PlaF: Crystal structure oriented in the membrane by the PPM method. PlaFA: Chain A from PlaF dimer oriented as in the dimer. The entrance of the active site cleft is more than 5 Å above the membrane bilayer surface. t-PlaFA: Extracted monomer A oriented using the PPM method. Cocrystallized MYR, 11A, and OG (depicted in pink), although not included in the simulations, are shown in the figures to highlight the orientation of the active site cleft. Arrows between the structures reflect the predicted states of equilibria under physiological conditions in Pseudomonas aeruginosa. Percentages of the different states are obtained from the molecular simulations (see main text and (e)). (b) MD simulations of monomeric PlaF. Time course of the orientation of monomeric PlaF with respect to the membrane starting from the PlaFA configuration as observed in the structure (left). In 80% of the trajectories, the monomer ends in a tilted configuration (marked with *). When starting from t-PlaFA (right), in all cases, the structure remains tilted. This shows a significant tendency of the monomer to tilt (McNemar’s Χ2=6.125, p=0.013). (c) Potential of mean force (PMF) of monomer tilting. The distance between the COM of Cα atoms of residues 33–37 (yellow, and gray spheres) and the COM of the C18 of the oleic acid moieties of all lipids in the membrane (continuous horizontal line in the membrane slab) was used as a reaction coordinate. The shaded area shows the standard error of the mean obtained by dividing the data into four independent parts of 50 ns each. The yellow shaded regions are the integration limits used to calculate Ktilting (Equation 5). The spheres in the PMF relate to monomer configurations shown in the inset. (d) PMF of dimer separation. The distance between the COM of Cα atoms of residues 25–38 of each chain was used as the reaction coordinate. The shaded area shows the standard error of the mean obtained by dividing the data into four independent parts of 50 ns each. Insets show representative structures at intermediate reaction coordinate values. (e) Percentage of PlaF monomer as a function of total PlaF concentration in the membrane according to the equilibria shown in (c) and (d). The monomer percentage was computed according to Equations 7–11 (see Materials and methods and SI for details). The red line shows the experimentally determined PlaF concentration under overexpressing conditions in P. aeruginosa p-plaF, while the blue-dashed region shows an estimated span for the PlaF concentration in P. aeruginosa wild-type (see Materials and methods for details). Calculated percentages are shown in (a).
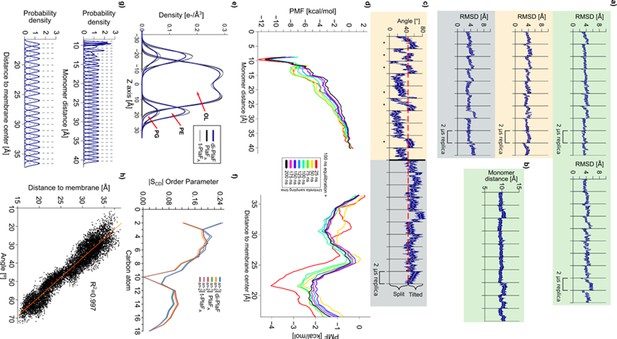
Structural variations, membrane parameters, and tests for PMF convergence.
(a) Root mean square deviations during 10 independent, unbiased molecular dynamics (MD) simulations of (I) the di-PlaF, where each chain was measured independently (green, left and right), (II) monomeric PlaF started in the PlaFA configuration (beige), and (III) monomeric PlaF started in the tilted configuration (t-PlaFA, gray), computed with respect to the respective initial structure. Most simulations reached a plateau at ~4 Å. (b) di-PlaF monomer distance during MD simulations. In 10 independent replicas of 2 µs, the dimer does not show a tendency to separate on the time scale of the MD simulations according to the distance between the COM of Cα atoms of residues 25–38 of each monomer. (c) Time course of the orientation of monomeric PlaF starting from the PlaFB (left) and t-PlaFB (right) configurations. Six out of 10 PlaFB replicas show tilting of the monomer in less than 2 μs, while all 10 replicas that started in the t-PlaFB configuration stayed tilted, similar to simulations started from PlaFA and t-PlaFA. (d) Convergence of the PMFs for dimer separation (left) and monomer tilting (right). The plots show PMFs computed every 25 ns of umbrella simulations for each window; the first 100 ns of umbrella simulations were considered equilibration phase and removed. (e) Electron density profiles of membrane components averaged over 10 independent, unbiased MD simulations of di-PlaF, PlaFA, or t-PlaFA configurations for the phospholipid head groups (PE and PG) and oleic acid tails (OL). The obtained shapes correspond with those generally found by experiment and MD simulations for biomembranes (Dickson et al., 2014; Tristram-Nagle et al., 1998; Liu and Nagle, 2004). (f) SCD order parameter of the lipid phase averaged over 10 independent, unbiased MD simulations of di-PlaF, PlaFA, or t-PlaFA configurations. The carbon atoms are numbered according to their position in the phospholipid tail. A clear dip is seen at the unsaturated position of the oleic acid tail, and the overall shape resembles a structured membrane bilayer, as previously described (Dickson et al., 2014; Vemparala et al., 2011). (g) Distribution of reaction coordinate values obtained by umbrella sampling of dimer separation (top) and monomer tilting (bottom). The dashed lines represent the restrained distance used for each window. In both cases, a force constant of 4 kcal mol–1 Å–2 was used, obtaining distributions with a median overlap of 8.2% and 8.6%, respectively. For details, see the main text. (h) Distance to membrane center versus tilting angle. The scatter plot shows the distance of the COM of residues 33–37 to the membrane center versus the tilting angle during the first microsecond of MD simulations of the tenth replica, starting from the PlaFA configuration (R2=0.997, p<0.001). The red dots represent the structures used as starting conformations for calculating the PMF of the tilting process.
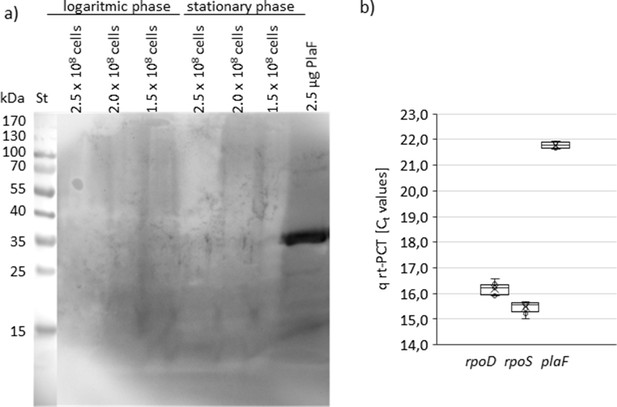
Detection of PlaF by Western blotting and qRT-PCR.
(a) Western blot was performed using anti-PlaF antiserum; Pseudomonas aeruginosa cells were from logarithmic (OD580nm 1) and stationary phase (OD580nm 3). Cell count was determined by counting colonies formed on LB agar plates. Purified PlaF was used as a positive control. Dimensions of P. aeruginosa cells in the exponential phase are 3.14×0.75 µm2 (Deforet et al., 2015), which yields the surface area of 3.12×106 nm2. (b) qRT-PCR analysis of transcription of rpoD, rpoS, and plaF genes in P. aeruginosa PA01 overnight culture. LB, lysogeny broth; qRT-PCR, quantitative real-time-PCR.
-
Figure 7—figure supplement 2—source data 1
Uncropped Western blot shown in Figure 7—figure supplement 2.
- https://cdn.elifesciences.org/articles/72824/elife-72824-fig7-figsupp2-data1-v2.tif
-
Figure 7—figure supplement 2—source data 2
Data used to make Figure 7—figure supplement 2.
- https://cdn.elifesciences.org/articles/72824/elife-72824-fig7-figsupp2-data2-v2.pdf
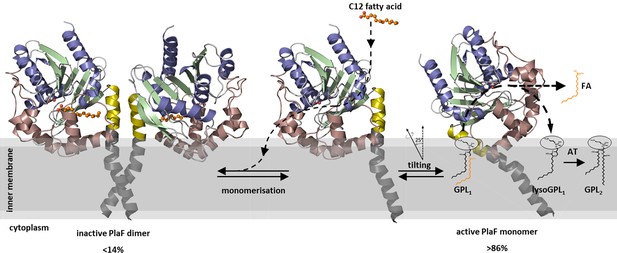
A model of PlaF-mediated membrane GPL remodeling.
PlaF is anchored with the TM helix to the inner membrane of Pseudomonas aeruginosa (Figures 1c and 4c), where it forms an inactive dimer (Figure 5c). Monomerization (Figure 5c) and subsequent spontaneous tilting (Figure 7) lead to activation. Binding of dodecanoic acid (C12) to monomeric PlaF triggers dimerization (Figure 6c) and inhibits enzymatic activity (Figure 6a). Tilting constrains the active site cavity of PlaF to the membrane surface such that GPL substrates can enter (GPL1, Figure 2), which are hydrolyzed to FA and lysoGPL1. A yet unknown acyl transferase possibly acylates lyso-GPL1 to yield modified GPL2 (Figure 2). GPL, glycerophospholipid.
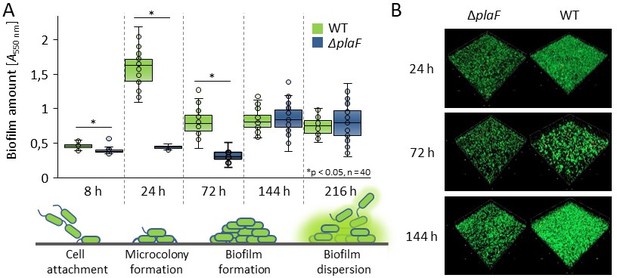
PlaF is a novel P. aeruginosa biofilm effector.
(A) Biofilm amount of P. aeruginosa ΔplaF and the wild-type strain cultivated in 96-well MTP (LB medium, 37°C, without aeration) was quantified by staining the cells attached to MTP with crystal violet. The results are mean ± standard deviation of five biological replicates, each measured eight times. Statistical analysis was performed using the t-test, * p < 0.05. (B) Biofilm architecture was analysed by CLSM after 24, 72, and 144 h growth at 37ºC in a flow cell with a continuous supply of LB medium. Shown are representative figures of two biological replicates analysed by imaging three different sections (100 x 100 µm) of the cover glass.

P. aeruginosa ΔplaF mutant strain is impaired in swimming motility.
The growth of the strains on LB agar swimming plates was quantified using ImageJ and expressed as swimming area, *** p < 0.00005, n = 10.
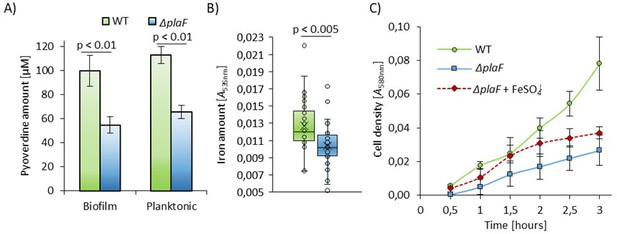
Iron-acquisition is disbalanced in P. aeruginosa ∆plaF.
A) Strains were grown statically at 37°C in 96-well microtiter plates for biofilm formation or in Erlenmeyer flasks under planktonic conditions for 24 h. Pyoverdine concentration was quantified in cell-free supernatant of P. aeruginosa PA01 and ∆plaF grown in plastic 96-well microtiter plates (biofilm) or in Erlenmeyer flasks (planktonic) at 37°C for 24 h. Results are mean ± S.D. B) P. aeruginosa ΔplaF shows a lower intrecellular iron concentration than the wild type. Iron was quantified [Tamarit, J., et al., Anal Biochem, 2006] in cells isolated from the cultures grown 18 h in LB medium at 37 C. C) The growth curves of P. aeruginosa PA01 and ∆plaF (n = 3) in M9 minimal medium under iron-limiting conditions and in the presence of 100 µM FeSO4 were determined by measuring the optical density at 580 nm. Results are mean ± S.D. The growth of P. aeruginosa ∆plaF and PA01, as well as P. aeruginosa ∆plaF and ∆plaF + FeSO4, differ significantly (p < 0.05).
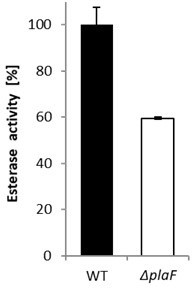
Esterase activity of P. aeruginosa ΔplaF strain.
P. aeruginosa PAO1 and ΔplaF strains were grown overnight in LB-medium at 37°C. Cells were harvested by centrifugation, suspended in Tris‑HCl buffer (100 mM, pH 8) to equal cell count, and enzyme activities were determined with p-nitrophenyl butyrate as the substrate. The results are mean ± standard deviations of three biological replicates, each measured three times.
Videos
MD simulation of monomeric PlaFA in GPL bilayer.
Blue and red spheres indicate head groups of GPLs in two leaflets of the bilayer.
Tables
Data collection and refinement statistics on PlaF.
X-ray data | |
---|---|
Beamline/detector | ID29, ESRF (Grenoble, France)/DECTRIS PILATUS 6M |
Wavelength (Å)/monochromator | λ=0.96863/channel-cut silicon monochromator, Si (111) |
Resolution range (Å) | 47.33–2.0 (2.05–2.0)* |
Space group | I 41 2 2 |
Unit cell (a=b), c (Å); α=β=γ | a=133.87 c=212.36; 90° |
Total reflections | 669,964 (47,385) |
Unique reflections | 65,113 (4527) |
Multiplicity | 10.3 (10.5) |
Completeness (%) | 100.0 (100.0) |
Mean I/sigma (I) | 24.6 (2.5) |
Wilson B-factor (Å2) | 38.3 |
R-merge (%) | 5.3 (91.3) |
R-meas (%) | 5.6 (100.6) |
Refinement | |
R-work (%) | 16.3 (23.15) (2.071–2.0)* |
R-free (%) | 18.57 (27.81) |
Number of atoms | 5187 |
Macromolecules | 4831 |
Ligands | 123 |
Water | 233 |
Protein residues | 620 |
RMS (bonds) | 0.008 |
RMS (angles) | 1.07 |
Ramachandran favored (%) | 99 |
Ramachandran outliers (%) | 0 |
Clashscore | 3.14 |
Average B-factor (Å2) | 49.1 |
Macromolecules (Å2) | 48.8 |
Ligands (Å2) | 79.2 |
Solvent (Å2) | 47.9 |
-
*
Values in parentheses are for the highest resolution shell.
Material used in this work.
Material | Ordering details |
---|---|
Galleria mellonella larvae | Fauna Topics GmbH, order number: 527 |
Trypsin, porcine, MS grade | Merck, order number: 650279 |
Anti-SecG antiserum | gift of R. Voulhoux, CNRS AMU LCB, Marseille |
Anti-lipid A antibodies | Acris Antibodies, Herford, Germany, order number: BP 2235 |
Ni-NTA agarose | Macherey–Nagel, Düren, Germany, order number: 745400 |
n-Octyl-β-D-glucoside | Merck, order number: 850511P |
para-Nitrophenyl butyrate | Sigma-Aldrich, order number: N9876 |
Glycerophospholipids | Avanti Polar Lipids, Alabaster, USA |
NEFA-HR(2) kit | Wako Chemicals, Richmond, USA, order number: 999-34691 |
[N-((6-(2,4-DNP)amino)hexanoyl)-1-(BODIPYFL C5)-2-hexyl-sn-glycero-3-phosphoethanolamine] | Thermo Fisher Scientific Inc, Waltham, MA, order number: A10070 |
1-O-(6-BODIPY558/568-aminohexyl)-2-BODIPYFL C5-Sn-glycero-3-phosphocholine | Thermo Fisher Scientific Inc, Waltham, MA, order number: A10072 |
N-((6-(2,4-dinitrophenyl)amino)hexanoyl)-2-(4,4-difluoro-5,7-dimethyl-4-bora-3a,4a-diaza-s-indacene-3-pentanoyl)–1-hexadecanoyl-sn-glycero-3-phosphoethanolamine triethylammonium salt | Thermo Fisher Scientific Inc, Waltham, MA, order number: D23739 |
Thermomyces lanuginosus PLA1 | Sigma-Aldrich, order number: L3295 |
Naja mocambique mocambique PLA2 | Sigma-Aldrich, order number: P7778 |
Dimethyl pimelimidate | Merck, order number: 80490 |
Bis(sulfosuccinimidyl) glutarate | Thermo Fisher Scientific, order number: 21610 |
Bis(sulfosuccinimidyl) suberate | Thermo Fisher Scientific, order number: 21586 |
PD-10 columns | Merck, order number: GE17-0851-01 |
NHS Labeling Kit | NanoTemper, Munich, Germany, order number: MO-L011 |
4-Methylumbelliferyl palmitate | Sigma-Aldrich; order number: M7259 |
CytoTox 96 non-radioactive cytotoxicity assay | Promega, order number: G1780 |
NucleoSpin RNA Preparation Kit | Macherey–Nagel, Düren, Germany, order number: 740955 |
RNase-Free DNase Kit | Qiagen, Hilden, Germany, order number: 79254 |
Ambion DNA-Free DNase Kit | Thermo Fisher Scientific, Darmstadt, Germany, order number: AM1906 |
Maxima First Strand cDNA Synthesis Kit | Thermo Fisher Scientific, Darmstadt, Germany, Order number: K1641 |
SYBR Green/ROX qPCR Master Mix | Thermo Fisher Scientific, Darmstadt, Germany, order number: K0221 |
N-Methyl-N-(trimethylsilyl) trifluoroacetamide | Sigma-Aldrich; order number: 69479 |
Additional files
-
Supplementary file 1
All phospholipid species identified in P. aeruginosa PA01 and ∆plaF by Q-TOF MS/MS.
- https://cdn.elifesciences.org/articles/72824/elife-72824-supp1-v2.xlsx
-
Supplementary file 2
Phospholipid species significantly differentially abundant in P. aeruginosa wild-type, ∆plaF, and ∆plaF::plaF.
- https://cdn.elifesciences.org/articles/72824/elife-72824-supp2-v2.docx
-
Supplementary file 3
Properties of the cultures used for lipid extraction.
- https://cdn.elifesciences.org/articles/72824/elife-72824-supp3-v2.docx
-
Supplementary file 4
PlaF homologs in P. aeruginosa species with sequenced genomes.
- https://cdn.elifesciences.org/articles/72824/elife-72824-supp4-v2.xls
-
Supplementary file 5
List of interactions involving the ligand molecules.
- https://cdn.elifesciences.org/articles/72824/elife-72824-supp5-v2.docx
-
Supplementary file 6
List of interactions involving the dimer interface.
- https://cdn.elifesciences.org/articles/72824/elife-72824-supp6-v2.docx
-
Supplementary file 7
List of interactions# involving the catalytic triad residues S137, D258 and H286.
- https://cdn.elifesciences.org/articles/72824/elife-72824-supp7-v2.docx
-
Supplementary file 8
Residues lining the active site cavity and their interactions with ligands.
- https://cdn.elifesciences.org/articles/72824/elife-72824-supp8-v2.docx
-
Supplementary file 9
Michaelis-Menten constants for inhibition of PlaF with decanoic acid (FA C10).
- https://cdn.elifesciences.org/articles/72824/elife-72824-supp9-v2.docx
-
Supplementary file 10
Average 2D-RMSDall atom of residues 25 to 315 of the structures sampled along MD trajectories.[a].
- https://cdn.elifesciences.org/articles/72824/elife-72824-supp10-v2.docx
-
Transparent reporting form
- https://cdn.elifesciences.org/articles/72824/elife-72824-transrepform1-v2.docx
-
Source data 1
All supplementary data in editable format.
- https://cdn.elifesciences.org/articles/72824/elife-72824-data1-v2.zip