Glia-neuron coupling via a bipartite sialylation pathway promotes neural transmission and stress tolerance in Drosophila
Figures
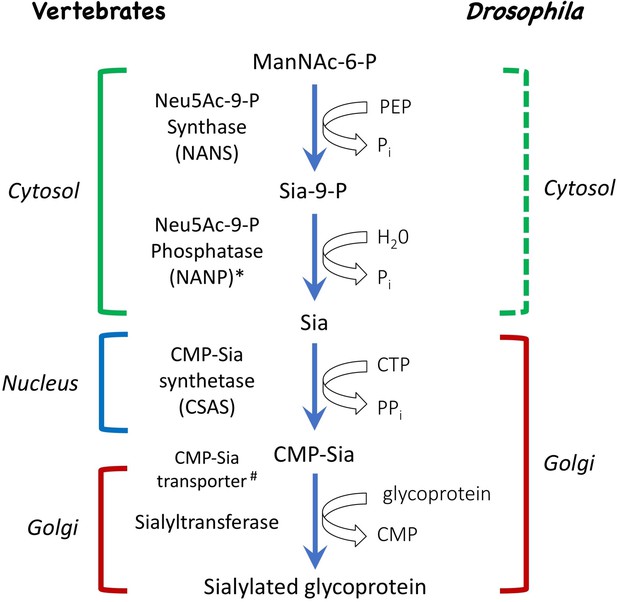
Schematic of the sialylation pathways in vertebrate and Drosophila.
In vertebrates, phosphorylated sialic acid is produced by N-acetylneuraminic acid synthase (Neu5Ac-9-P synthase, or NANS) from N-acetyl-mannosamine 6-phosphate (ManNAc-6-P), converted to sialic acid by N-acylneuraminate-9-phosphatase (Neu5Ac-9-P phosphatase, or NANP), and then activated by CMP-sialic acid synthetase (CSAS, also known as CMAS) to become CMP-Sia, the substrate for sialyltransferase enzymes that work in the Golgi and attach sialic acid to termini of glycan chains. While NANS and NANP work in the cytosol, CSAS enzymes normally localize to the nucleus in vertebrate cells, and the transfer of CMP-Sia to the Golgi requires CMP-Sia transporter. In Drosophila, both CSAS and sialyltransferase are localized in the Golgi, and CMP-Sia transporter is not required for sialylation. Biochemical activities of NANS, CSAS, and the Drosophila sialyltransferase (DSiaT) were confirmed in vitro (Kim et al., 2002; Koles et al., 2004; Viswanathan et al., 2006; Mertsalov et al., 2016). Dashed bracket: subcellular localization was not experimentally confirmed. #CMP-Sia transporter was not identified in invertebrates. *NANP was found to be not essential for sialylation (Willems et al., 2019).
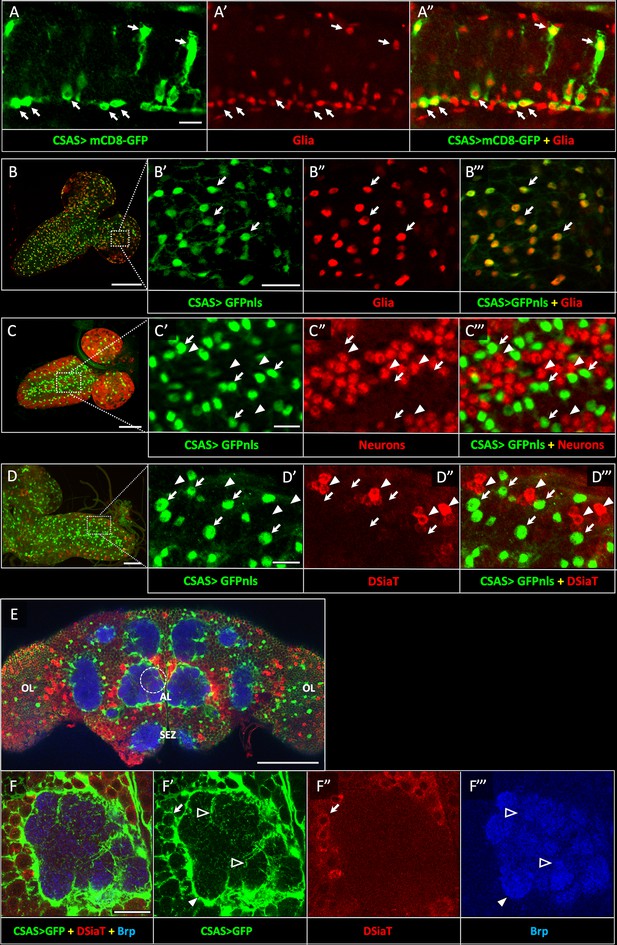
CSAS expression is restricted to glial cells and shows no overlap with the expression of DSiaT during development and in the adult brain.
(A–A”) CSAS expression (green) is detected in glial cells (Repo, red) of the developing ventral ganglion during late embryonic stages. Arrows indicate examples of glial cells with CSAS expression. A” is the overlay of green (A) and red (A’) channels. (B–B’’’) CSAS expression (green) is present in the majority of glial cells (Repo, red) in the CNS at larval stages. (B’–B’’’) are zoomed-in images of a brain region outlined in B, B’’’ is overlay of B’ and B”. (C–C’’’) CSAS expression (green) is not detected in neurons (Elav, red) in the CNS at larval stages. Arrows and arrowheads indicate examples of cells with CSAS and Elav expression, respectively. (C’–C’’’) are zoomed-in images of a ventral ganglion region outlined in C, C’’’ is overlay of C’ and C”. (D–D’’’) CSAS expression (green) is not detected in the CNS cells expressing DSiaT (red) at larval stages. Arrows and arrowheads indicate examples of cells with CSAS and DSiaT expression, respectively. (D’–D’’’) are zoomed-in images of a ventral ganglion region outlined in D, D’’’ is overlay of D’ and D”. (E) CSAS (green) is expressed throughout the adult brain, including glial cells in the optic lobes (OL), around the antennal lobe (AL), and the sub esophageal zone (SEZ), but CSAS expression is not detected in DSiaT-expressing neurons (red). (F–F’’’) Zoomed-in images of the antennal lobe region indicated by a dashed circle in E. CSAS-expressing cells produce processes surrounding the soma of DSiaT-expressing projection neurons (arrow), enveloping the antennal lobe (filled arrowhead), and sending fine projections inside the glomeruli (empty arrowheads). Brp staining (blue) labels neuropil in E–F. (A) Embryonic stage 17, lateral view, anterior is left, ventral is up; (B) third instar larval stage, anterior is top-right; (C) first instar larval stage, anterior is top-right; (D) third instar larval stage, anterior is left; (E–F) adult brain, frontal view. Scale bars: 10 μm (A, C’, D’), 100 μm (B, E), 20 μm (B’, F), 50 μm (C–D). CSAS expression was visualized using CSAS-LexA driver-induced expression of GFP with membrane (mCD8-GFP) or nuclear localization (GFPnls) tags. Images were acquired using confocal microscopy.
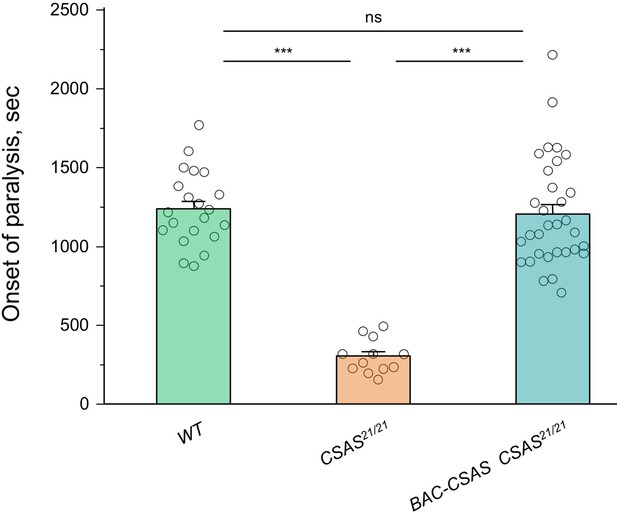
Rescue of the temperature-sensitive (TS) paralysis phenotype of CSAS mutants by BAC-CSAS.
The BAC-CSAS transgenic construct that was used to generate the CSAS-LexA driver can fully rescue the TS paralysis phenotype of CSAS mutants, suggesting that BAC-CSAS-mediated expression of CSAS recapitulates the endogenous CSAS expression. See Supplementary file 1 for detailed genotypes. In all experiments, for each genotype, 5-day-old females were assayed for TS paralyses at 38°C. Sample size per genotype: WT, 21; CSAS21/21, 12; BAC-CSAS CSAS21/21, 32. Error bars are SEM. One-way ANOVA with post hoc Tukey test was used for statistical analyses. ns, no significant difference (p>0.05); ***p<0.001.
-
Figure 2—figure supplement 1—source data 1
Source data for Figure 2—figure supplement 1.
- https://cdn.elifesciences.org/articles/78280/elife-78280-fig2-figsupp1-data1-v2.xlsx
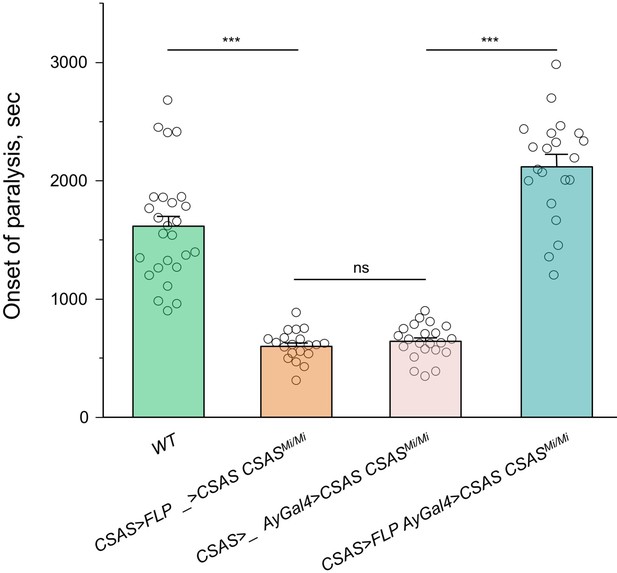
Rescue of the temperature-sensitive (TS) paralysis phenotype of CSAS mutants by CSAS-LexA-induced transgenic expression of CSAS cDNA.
CSAS-LexA-mediated expression of CSAS cDNA in CSAS mutants results in a full rescue of the TS paralysis phenotype, which supports the conclusion that CSAS-LexA expression recapitulates the endogenous pattern of CSAS expression. UAS-CSAS was induced via activation of AyGal4 using LexAop-FLP driven by CSAS-LexA. The control genotypes (without AyGal4 or without LexAop-FLP) showed no rescue, indicating that the rescue was specifically induced by CSAS-LexA and not associated with possible ‘leaking’ of Lex-Aop-FLP or AyGal4 constructs. In all experiments, at least twenty 5-day-old females were assayed for TS paralyses at 38°C for each genotype. Error bars are SEM. One-way ANOVA with post hoc Tukey test was used for statistical analyses. ns, no significant difference (p>0.05); *** p<0.001. See Supplementary file 1 for detailed genotypes.
-
Figure 2—figure supplement 2—source data 1
Source data for Figure 2—figure supplement 2.
- https://cdn.elifesciences.org/articles/78280/elife-78280-fig2-figsupp2-data1-v2.xlsx
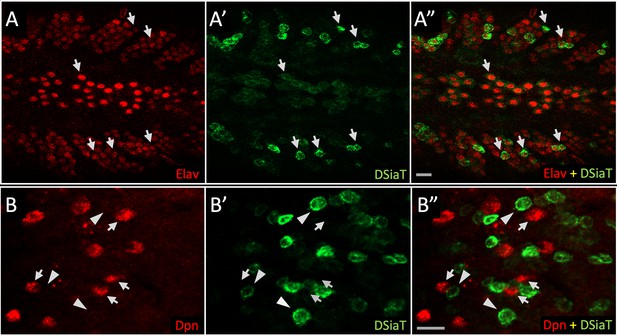
DSiaT-HA expression is detected in neurons but not in neuroblasts.
Double immunostainings were performed using antibodies for Elav and Deadpan (Dpn), pan-neuronal, and neuroblast markers, respectively (Yao and White, 1994; Lee et al., 2006). (A) Expression of Elav (red); (A’) expression of DSiaT-HA (green); (A”) overlay of red (A) and green (A’) channels shows that DSiaT-HA is expressed only in Elav-positive cells. Arrows indicate examples of cells co-expressing DSiat-HA and Elav. (B) Expression of Dpn (red); (B’) expression of DSiaT-HA (green); (B”) overlay of red (B) and green (B’) channels shows that Dpn and DSiaT are expressed in different cells. Examples of cells expressing Dpn or DSiaT-HA are indicated by arrows or arrowheads, respectively. The images show regions of the third instar larval ventral ganglion. Scale bars, 10 μm.
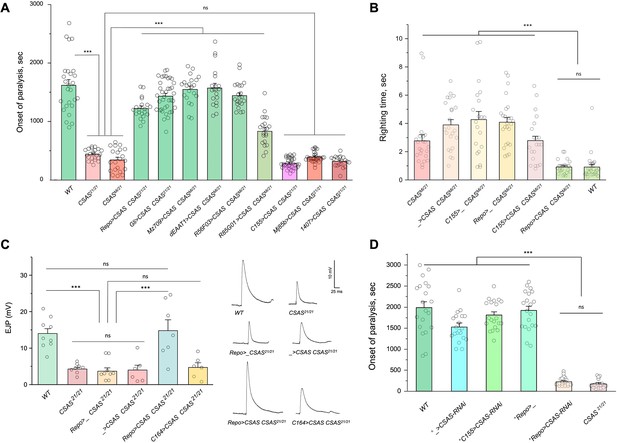
CMP-sialic acid synthetase (CSAS) is required in glial cells, but not in neurons, for normal neural functions.
(A) Rescue of TS paralysis phenotype of CSAS mutants using UAS-Gal4 system. CSAS21 (null) and CSASMi (strong loss-of-function) mutant alleles were used in homozygous and heteroallelic combinations. The expression of transgenic UAS-CSAS construct was induced using a panel of cell-specific Gal4 drivers. A pan-neuronal Gal4 driver (C155) or drivers broadly expressed in the CNS neurons (1407 and Mj85b) did not induce rescue, while the expression of UAS-CSAS by glial-specific drivers, including Repo, Gli, and Mz709 (expressed in nearly all glial cells, ensheathing glial cells, and subperineural glia, respectively), rescued the phenotype. Analyses of control mutant genotypes (UAS-CSAS without driver, and driver-only mutant genotypes) confirmed the specificity of the rescue results (Figure 3—figure supplement 1). The expression of CSAS induced by C155 was confirmed using immunostaining (Figure 3—figure supplement 2). At least 19 files (5-day-old females) were assayed for each genotype. (B) Locomotor phenotype of CSAS mutants rescued using UAS-Gal4 system. The expression of UAS-CSAS induced in neurons by C155 driver did not rescue the phenotype, while the glial-specific expression driven by Repo-Gal4 resulted in full rescue. Mutant genotypes with UAS-CSAS alone or drivers alone were used as controls, and they did not show rescue. At least 20 females were assayed for each genotype. (C) Rescue of neuromuscular excitatory junction potential (EJP) defect of CSAS mutants using UAS-Gal4 system. The reduced EJP phenotype was rescued by glial-specific expression of UAS-CSAS induced by Repo-Gal4. The expression of UAS-CSAS in motoneurons using C164-Gal4 did not result in rescue. Representative EJP traces are shown on the right. EJPs were evoked in 0.5 mM Ca2 and analyzed at muscle 6/7 neuromuscular junctions (NMJs) of third instar larvae (see Materials and methods for details). 6-9 larvae were assyed for each genotype. (D) Cell-specific RNAi-mediated knockdown reveals that CSAS is required in glial cells. UAS-CSAS-RNAi was induced in glial cells by Repo-Gal4, which resulted in TS paralyses phenotype. The expression of UAS-CSAS-RNAi in neurons induced by C155-Gal4 did not cause the phenotype. To potentiate the effect of CSAS-RNAi, the knockdown experiments were performed in the genetic background with co-expression of UAS-dcr2 and heterozygous for CSAS21 mutant allele (^, genotypes with matching genetic background including UAS-dcr2 and CSAS21/+). At least 20 females were assayed for each genotype (all data points represent different flies). In all panels: error bars are SEM; one-way ANOVA with post hoc Tukey test was used for statistical analyses; *** p<0.001; ns, no significant difference (p>0.05). See Supplementary file 1 for detailed genotype information.
-
Figure 3—source data 1
Source data for Figure 3A.
- https://cdn.elifesciences.org/articles/78280/elife-78280-fig3-data1-v2.xlsx
-
Figure 3—source data 2
Source data for Figure 3B.
- https://cdn.elifesciences.org/articles/78280/elife-78280-fig3-data2-v2.xlsx
-
Figure 3—source data 3
Source data for Figure 3C.
- https://cdn.elifesciences.org/articles/78280/elife-78280-fig3-data3-v2.xlsx
-
Figure 3—source data 4
Source data for Figure 3D.
- https://cdn.elifesciences.org/articles/78280/elife-78280-fig3-data4-v2.xlsx
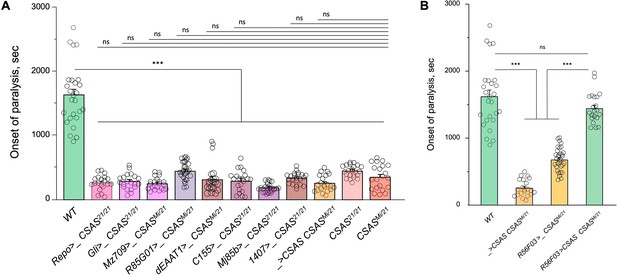
Temperature-sensitive (TS) paralysis assays of control genotypes for cell type-specific rescue of CSAS mutants (Figure 3A).
The assays were carried out and analyzed the same way as described for the experiments shown in Figure 3A. (A) The results show that the transgenes alone (Gal4 drivers or UAS-CSAS) do not affect the paralysis phenotype of CSAS mutants. (B) Controls for rescue using R56F03-Gal4, a neuropile ensheathing glia-specific driver. R56F03-driven expression of UAS-CSAS can fully rescue the paralysis phenotype of CSAS mutants, while neither of the transgenes alone can induce the rescue. (A-B) For each genotype, 20-32 five-day-old female flies were assayed for TS paralyses at 38°C. Error bars are SEM; one-way ANOVA with post hoc Tukey test was used for statistical analyses; *** p<0.001; ns, no significant difference (p>0.05).See Supplementary file 1 for detailed genotypes.
-
Figure 3—figure supplement 1—source data 1
Source data for Figure 3—figure supplement 1.
- https://cdn.elifesciences.org/articles/78280/elife-78280-fig3-figsupp1-data1-v2.xlsx
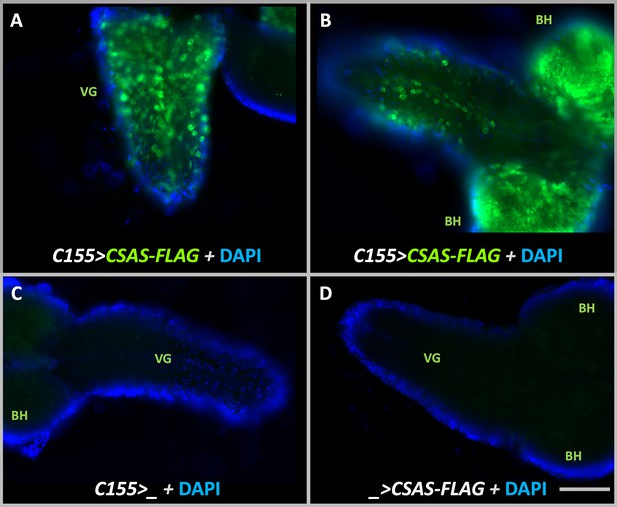
Transgenic expression of UAS-CSAS induced by C155-Gal4 driver in larval brains assayed by immunostaining.
(A–B) Examples of third instar larval brains with the transgenic expression of UAS-CSAS-FLAG. Prominent expression of CSAS-FLAG was induced in the ventral ganglion (VG) and brain hemispheres (BH). (C–D) Control brains without UAS-CSAS-FLAG or C155-Gal4 did not show CSAS-FLAG expression. (A–D) All brains were stained using a master mix of primary and secondary antibodies. DAPI staining was added to outline brain morphology. The images were acquired using the same exposure time. Scale bar is 100 μm.
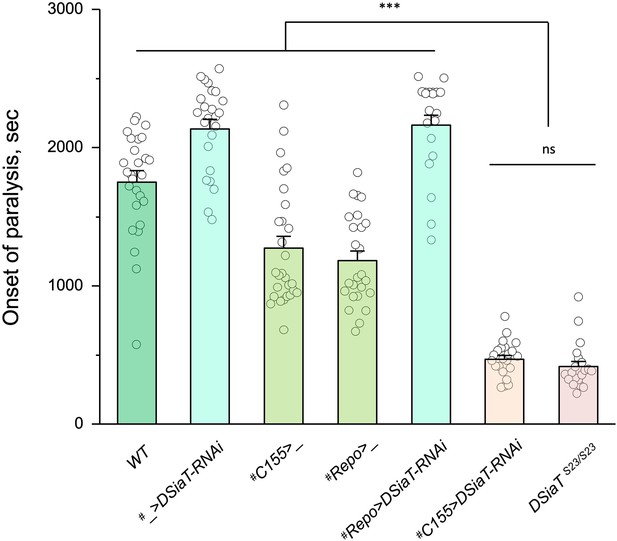
Cell-specific knockdown revealed that DSiaT is required in neurons.
UAS-DSiaT-RNAi was induced in neurons by C155-Gal4, which resulted in temperature-sensitive (TS) paralyses phenotype. The expression of UAS-DSiaT-RNAi in glial cells by Repo-Gal4 did not produce the phenotype. To potentiate the effect of RNAi, knockdown was carried out in the genetic background heterozygous for DSiaT mutant allele (DSiaTS23/+) and flies were reared at 29°C. 20-28 five-day-old female flies were assayed for each genotype. #, genotypes with matching genetic background heterozygous for DSiaTS23. Error bars are SEM; one-way ANOVA with post hoc Tukey test was used for statistical analyses; *** p<0.001; ns, no significant difference (p>0.05).See Supplementary file 1 for detailed genotypes.
-
Figure 4—source data 1
Source data for Figure 4.
- https://cdn.elifesciences.org/articles/78280/elife-78280-fig4-data1-v2.xlsx
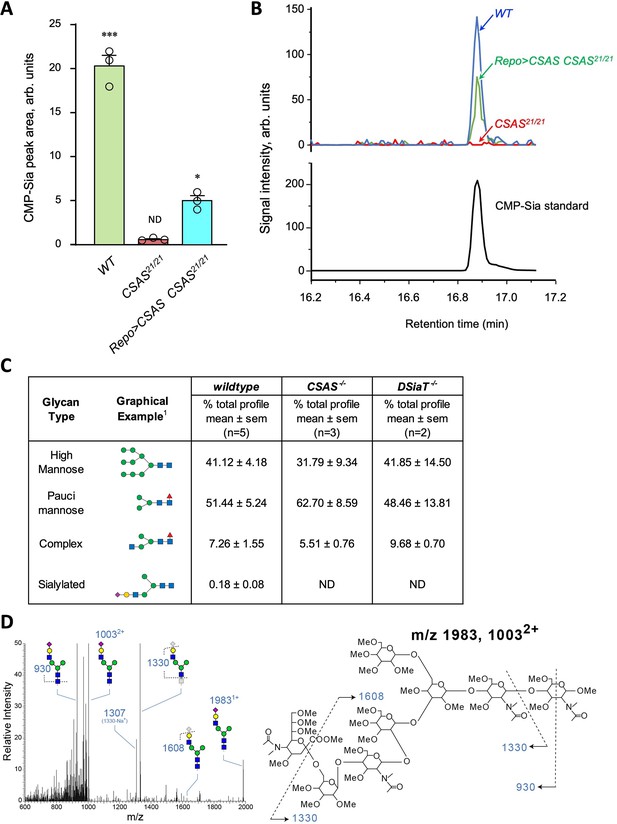
Analysis of CMP-Sia and sialylated glycans in Drosophila.
(A) Quantification of CMP-Sia using LC-MS/MS by normalized peak area (see Materials and methods). CMP-Sia was detected in wild-type flies (WT) but not in CSAS mutants (CSAS21/21). Transgenic expression of UAS-CSAS in glial cells of CSAS mutants by Repo-Gal4 (Repo>CSAS CSAS21/21, a rescue genotype) could significantly restore the level of CMP-Sia. ND, not detected (signal/noise ratio <1). Data were obtained from three biological repliacates per genotype, each including 100 adult flies (50 males plus 50 females) analyzed in three technical repeats. Error bars are SEM; one-way ANOVA with post hoc Tukey test was used for statistical analyses; ***, *, differences with p<0.001 and p<0.05, respectively. (B) Typical examples of normalized CMP-Sia signal intensity traces for wild-type, CSAS mutant, and rescue genotypes, as well as CMP-Sia standard. (C) Summary of glycomic analyses of N-linked glycans in wild-type Drosophila, CSAS, and DSiaT mutants. The N-glycome of third instar larval brains was analyzed. No sialylated N-glycans were detected in the mutants. Samples from wild-type and mutant genotypes were analyzed in parallel using the glycomic protocol described in Materials and methods. n, number of replicates. 1Most abundant glycan detected in wild-type is shown as representative. ND, not detected. Graphical representation and description of structures are according to the accepted glycan nomenclature (Aoki et al., 2007; Varki et al., 2015b). See extended table of N-glycan species identified by glycomic analyses in Figure 5—source data 3. (D) Example of fragmentation of a sialylated N-glycan extracted from wild-type larvae. MS/MS fragmentation of the doubly charged, permethylated ion at m/z=1003 (m+Na)2+ reveals signature ions consistent with loss of charge (m/z=1983), loss of sialic acid (m/z=608, 1330, 1307), as well as cross-ring fragmentation and loss of reducing terminal residues. The fragmentation pattern confirms the presence of the depicted sialylated structure. Similar fragmentation was not detected in DSiaT or CSAS mutants.
-
Figure 5—source data 1
Source data for Figure 5A.
- https://cdn.elifesciences.org/articles/78280/elife-78280-fig5-data1-v2.xlsx
-
Figure 5—source data 2
Source data for Figure 5B.
- https://cdn.elifesciences.org/articles/78280/elife-78280-fig5-data2-v2.xlsx
-
Figure 5—source data 3
Extended table of N-glycan species identified by glycomic analyses in wild-type, DSiaT mutant, and CSAS mutant larval brains.
n, number of replicates. ND, not detected. Graphical representation and description of structures are according to the glycan nomenclature (Aoki et al., 2007; Varki et al., 2015b). Accession numbers are from GlyTouCan, the International Glycan Structure Repository (https://glytoucan.org/). All raw mass spectrometric data were deposited at GlycoPost (Watanabe et al., 2021), accession # GPST000260.
- https://cdn.elifesciences.org/articles/78280/elife-78280-fig5-data3-v2.docx
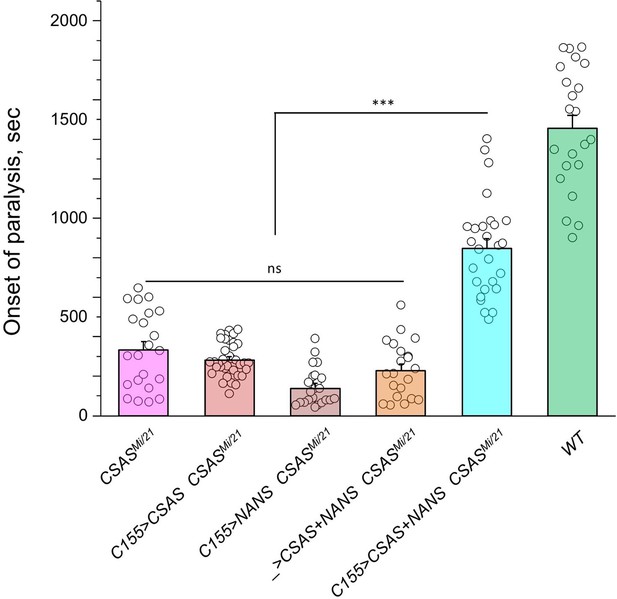
Transgenic co-expression of N-acetylneuraminic acid synthase (NANS) and CMP-sialic acid synthetase (CSAS) in neurons rescued the phenotype of CSAS mutants.
UAS-CSAS and UAS-NANS were expressed individually or together in neurons of CSASMi/21 mutants using C155-Gal4. The co-expression of CSAS and NANS could rescue the temperature-sensitive (TS) paralysis phenotype, while their individual expression did not result in rescue. 22-37 five-day-old female flies were assayed for each genotype. Error bars are SEM; one-way ANOVA with post hoc Tukey test was used for statistical analyses; *** p<0.001; ns, no significant difference (p>0.05). See Supplementary file 1 for detailed genotypes.
-
Figure 6—source data 1
Source data for Figure 6.
- https://cdn.elifesciences.org/articles/78280/elife-78280-fig6-data1-v2.xlsx
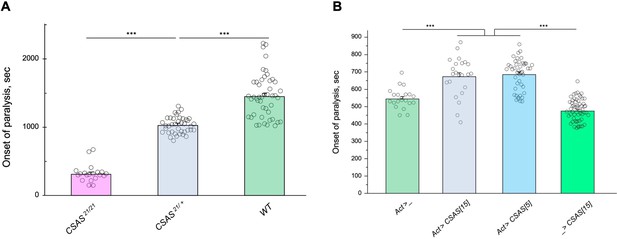
Tolerance to heat shock is very sensitive to the level of CMP-sialic acid synthetase (CSAS) activity.
(A) Comparing phenotypes of CSAS homozygous null mutants (CSAS21/21), CSAS mutant heterozygotes (CSAS21/+), and wild-type flies. Temperature-sensitive (TS) paralyses assays were performed at 38°C. All genotypes had matching genetic backgrounds: the CSAS21/21 mutants were outcrossed to wild-type flies (WT) at least seven times; the heterozygotes were obtained from the cross between WT and CSAS21/21. (B) Overexpression of CSAS increases tolerance to heat shock. Driver-only genotype (Act>_) was used as a ‘wild-type’ control with a matching genetic background. Two independent UAS-CSAS transgenic insertions on different chromosomes (designated as CSAS[5] and CSAS[15]) were tested to confirm the specificity of the effect. No-driver control (_>CSAS[15]) confirmed that the effect is indeed due to CSAS overexpression. TS paralyses assays were performed at 40°C to decrease the time to paralysis. All genotypes were multiply outcrossed to the same WT genetic background (w1118 Canton S). (A–B) At least 25 five-day-old females were assayed for each genotype. Error bars are SEM; one-way ANOVA with post hoc Tukey test was used for statistical analyses; *** p<0.001. See Supplementary file 1 for detailed genotypes.
-
Figure 7—source data 1
Source data for Figure 7A.
- https://cdn.elifesciences.org/articles/78280/elife-78280-fig7-data1-v2.xlsx
-
Figure 7—source data 2
Source data for Figure 7B.
- https://cdn.elifesciences.org/articles/78280/elife-78280-fig7-data2-v2.xlsx
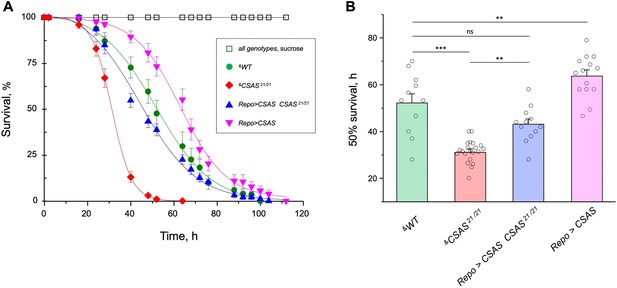
CMP-sialic acid synthetase (CSAS) affects tolerance to oxidative stress.
(A) Survival in oxidative stress conditions was assayed using a paraquat exposure paradigm. CSAS homozygous mutants (CSAS21/21), CSAS mutant rescue (Repo>CSAS CSAS21/21), wild-type (WT), and transgenic overexpression (Repo>CSAS) genotypes were exposed to 40 mM paraquat or sucrose as a control. Every genotype was assayed on paraquat using >10 independent biological replicates, each including a group of 8–12 flies; at least 50 flies were assayed for sucrose control. &, all genotypes had matching genetic backgrounds that included Repo-Gal4 driver. All mutant alleles and transgenic insertions were outcrossed to the same WT background multiple times. Statistical significance was analyzed by (i) log-rank test of cumulative survival data for each genotype (Figure 8—figure supplement 1) and (ii) one-way ANOVA with post hoc Tukey test applied to survival at 40, 48, and 52 hr. These analyses revealed significant differences between all genotypes (p<0.05), except for WT and rescue that were not always significantly different from each other. Error bars are SEM. (B) Comparison of 50% survival time on paraquat estimated from the data shown in A. Error bars are SEM. One-way ANOVA with post hoc Tukey test was used for statistical analyses; *** p<0.001; ** p<0.01; ns, no significant difference (p>0.05). See Supplementary file 1 for detailed genotypes.
-
Figure 8—source data 1
Source data for Figure 8A.
- https://cdn.elifesciences.org/articles/78280/elife-78280-fig8-data1-v2.xlsx
-
Figure 8—source data 2
Source data for Figure 8.
- https://cdn.elifesciences.org/articles/78280/elife-78280-fig8-data2-v2.xlsx
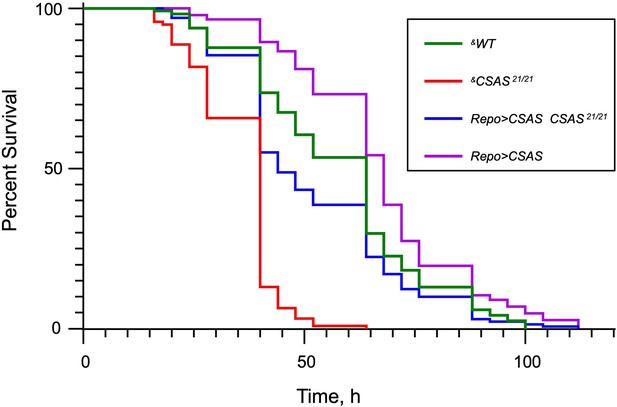
The Kaplan-Meier survival curves from paraquat exposure experiments.
The log-rank analysis revealed statistically significant differences (p<0.001) between all curves except for the pair of WT and Repo>CSAS CSAS 21/21 (rescue) genotypes. Number of flies analyzed per genotype: WT (‘wild-type’ control), 114; CSAS 21/21, 213; Repo>CSAS CSAS 21/21, 129; Repo>CSAS, 142. The statistical analysis was performed using GraphPad Prism software. &, all genotypes had matching genetic background including repo-Gal4. See Supplementary file 1 for detailed genotypes.
-
Figure 8—figure supplement 1—source data 1
Source data for Figure 8—figure supplement 1.
- https://cdn.elifesciences.org/articles/78280/elife-78280-fig8-figsupp1-data1-v2.xlsx
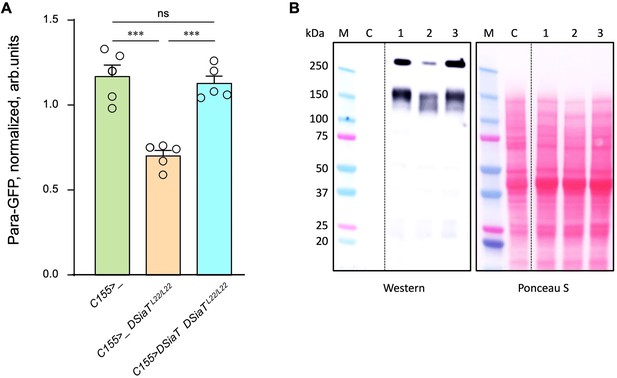
The level of Para is decreased in DSiaT mutants.
(A) Quantification of Para-GFP by western blots revealed that DSiaT mutants (C155>_ DSiaTL22/L22) have a lower level of Para-GFP as compared to a control ‘wild-type’ genotype (C155>_). The level of Para-GFP is restored in the mutants by transgenic expression of UAS-DSiaT using C155-Gal4 driver (C155>DSiaT DSiaTL22/L22, rescue genotype), which confirmed the specificity of the phenotype. Para-GFP signal was normalized to total protein amount analyzed by Ponceau S staining. The analysis is based on five biological replicates (data points shown), each including up to three technical repeats (see Materials and methods for details). Error bars are SEM. One-way ANOVA with post hoc Tukey test was used for statistical analyses; *** p<0.001; ns, no statistical difference (p>0.05). (B) A representative example of Para-GFP western blot and corresponding Ponceau S protein staining. M, molecular weight marker; C, control flies without Para-GFP; 1, C155>_; 2, C155>_ DSiaTL22/L22; 3, C155>DSiaT DSiaTL22/L22, rescue genotype. §, genotypes had matching genetic backgrounds that included C155-Gal4 driver and were heterozygous for para-GFP. Ten to fifteen 7-day-old females were used per genotype in each experiment. For complete genotype information, see Supplementary file 1.
-
Figure 9—source data 1
Source data for Figure 9A.
- https://cdn.elifesciences.org/articles/78280/elife-78280-fig9-data1-v2.xlsx
-
Figure 9—source data 2
Source data for Figure 9B.
- https://cdn.elifesciences.org/articles/78280/elife-78280-fig9-data2-v2.zip
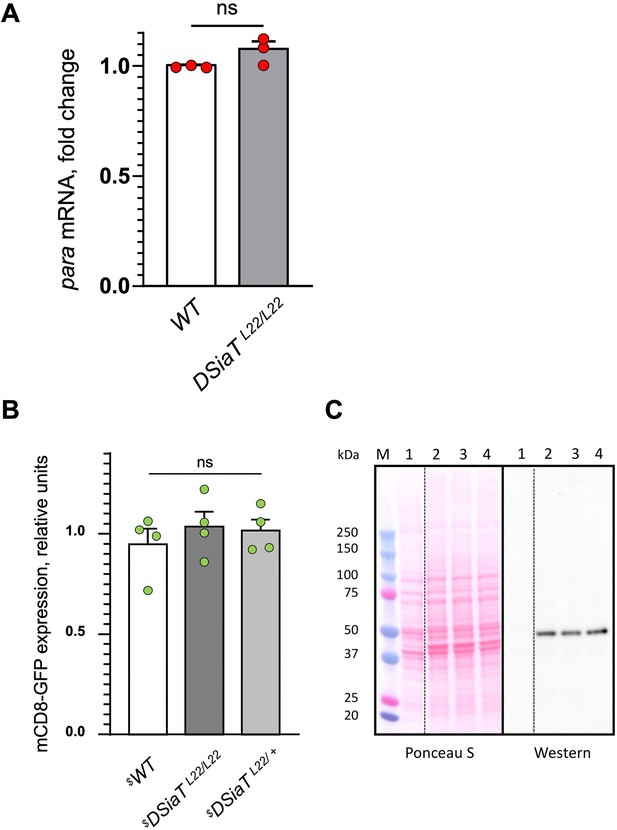
Analyses of para mRNA and mCD8-GFP expression in DSiaT mutants, as controls for the specificity of the posttranscriptional effect of DSiaT on the level of Para-GFP.
(A) Para qRT-PCR analyses revealed no significant difference between wild-type (WT) and DSiaT mutant flies based on three biological replicates. Error bars are SEM; two-tailed unequal variance t-test was used for statistical analysis; ns, no statistical difference (p>0.05) (B–C) Transgenic mCD8-GFP expression (Pfeiffer et al., 2010) induced by C155-Gal4 was analyzed as a control for a non-specific effect on membrane proteins expressed in neurons of DSiaT mutants. (B) No significant difference was detected in mCD8-GFP expression by western blots in four biological replicates between control (WT) and DSiaTL22/L22 mutants (heterozygous DSiaTL22/+ siblings we used as an additional control). One-way ANOVA with post hoc Tukey test was used for statistical analyses; ns, no statistical difference (p>0.05). (C) A representative example of SDS-PAGE analysis of mCD8-GFP expression, showing a Ponceau S protein staining and the corresponding mCD8-GFP western blot. M, molecular weight marker; 1, negative control, flies without mCD8-GFP expression; 2, mCD8-GFP expression in wild-type background; 3, mCD8-GFP expression in DSiaTL22/L22 mutants; 4, mCD8-GFP expression in DSiaTL22/+ heterozygotes. $, all genotypes had matching genetic background including C155-Gal4 driver. Ten to fifteen 7-day-old female flies were used in each experiment. See Supplementary file 1 for detailed genotypes.
-
Figure 9—figure supplement 1—source data 1
Source data for Figure 9—figure supplement 1A.
- https://cdn.elifesciences.org/articles/78280/elife-78280-fig9-figsupp1-data1-v2.xlsx
-
Figure 9—figure supplement 1—source data 2
Source data for Figure 9—figure supplement 1B.
- https://cdn.elifesciences.org/articles/78280/elife-78280-fig9-figsupp1-data2-v2.xlsx
-
Figure 9—figure supplement 1—source data 3
Source data for Figure 9—figure supplement 1C.
- https://cdn.elifesciences.org/articles/78280/elife-78280-fig9-figsupp1-data3-v2.zip
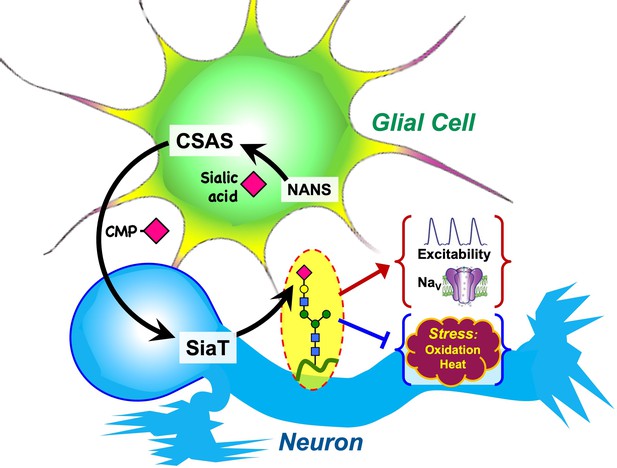
Graphical summary of the proposed mechanism of glia-neuron coupling via a bipartite sialylation pathway.
In Drosophila brain, the last two steps of the sialylation pathway that are mediated by CMP-sialic acid synthetase (CSAS) and Drosophila sialyltransferase (DSiaT) are separated between glia and neurons. As a result, glia provides CMP-Sia to neurons that carry out sialylation of glycoproteins. This new mode of glia-neuron coupling promotes neural excitability, maintains the normal level of voltage-gated Na+ channels (NaV), and counteracts the effects of heat and oxidative stress.

DSiaT expression in the adult brain is regulated via a posttranscriptional mechanism.
(A-B) The expression of DSiaT in the brain is significantly decreased in 10-day-old flies (B) as compared to newly eclosed flies (A, 0-day-old). Arrows indicate the regions of elevated expression in the olfactory system (projection neurons) and optic lobes. Asterisks indicate the increased background signal in the older brain (B). Images were obtained using identical experimental conditions (i.e. dissection, immunostaining, and imaging). (C) Quantitative RTPCR analysis of DSiaT mRNA expression in adult brains using primers for the two known splicoforms (short and long). Absence of correlation with the level of DSiaT protein expression (A-B) indicates that the expression of DSiaT undergoes a posttranscriptional regulation. Unpublished data obtained by Kate Koles.
Additional files
-
Supplementary file 1
Supplementary table of genetic strains and transgenic constructs used in the study.
- https://cdn.elifesciences.org/articles/78280/elife-78280-supp1-v2.docx
-
MDAR checklist
- https://cdn.elifesciences.org/articles/78280/elife-78280-mdarchecklist1-v2.docx