Structural and regulatory insights into the glideosome-associated connector from Toxoplasma gondii
Figures
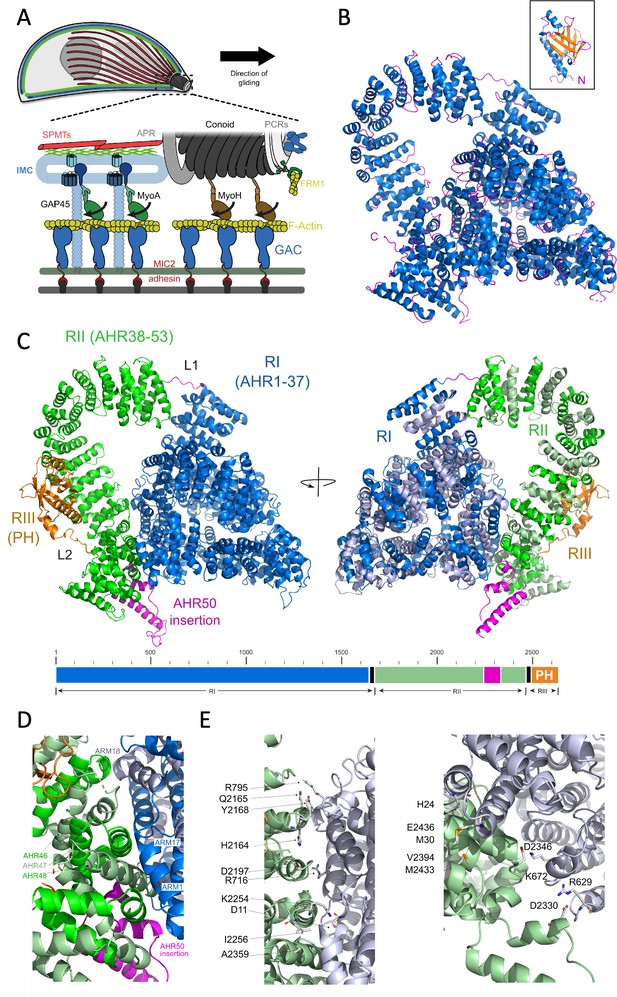
The organisation and structure of TgGAC.
(A) Schematic diagram of the apicomplexan glideosome. Key molecular components and structures are indicated, including subpellicular microtubules (SPMTs), inner membrane complex (IMC), preconoidal rings (PCRs), apical polar ring (APR), Formin-1 (FRM1), filamentous actin (F-actin), glideosome-associated protein 45 (GAP45), myosin motors (MyoA and MyoA), microneme protein 2 (MIC2), and glideosome-associated connector (GAC) protein. (B) Crystal structure of TgGAC7–2504 and representative nuclear magnetic resonance (NMR) model of TgGAC2505–2639 (inset). Helices shown in blue, β-strands in orange and loops in magenta. (C) Full-length TgGAC model comprising the combined crystal structure and NMR-validated AlphaFold2 structures in (B). Region I (R1: residues 1–1665) comprising the first 37 consecutive armadillo (ARM)/HEAT-like repeats (AHRs) is supercoiled into a three-layer pyramid structure (blue). AHR region II comprising 16 ARM repeats (RII: AHR38-53 residues 1670–2489) forms the superhelical arch (green). The C-terminal PH domain encompassing 2511–2639 of RIII is shown in orange (left). Second orientation related by a 180° rotation with even numbered AHRs shown in grey and odd number shown in blue for RI and green for RII. The R1–RII linker and AHR50 which has a helix-loop-helix insertion are shown in magenta. (D) The N/C interface between RI and RII showing key interacting AHRs. (E) Key residues specific interactions across the N/C interface. Cartoon representations coloured in light blue for RI and light green for RII.
-
Figure 1—source data 1
Maps and coordinates.
wwPDB X-ray structure validation; MTZ file for TgGAC X-ray diffraction data and final refined PDB file for TgGAC from X-ray diffraction.
- https://cdn.elifesciences.org/articles/86049/elife-86049-fig1-data1-v2.zip
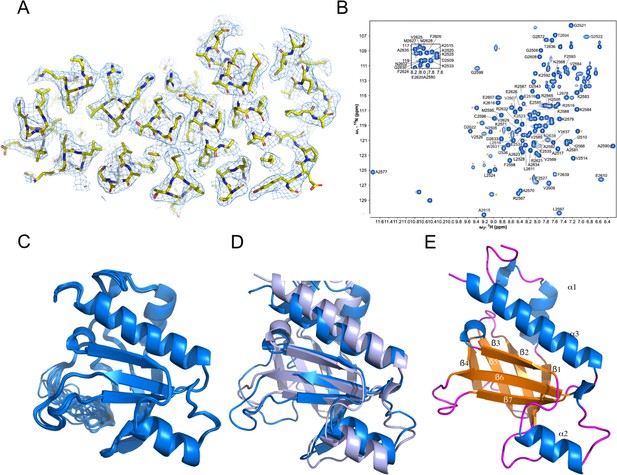
Assessing the quality of structural data for glideosome-associated connector (GAC).
(A) Representative X-ray crystallographic electron density map showing the fit to residues 1203–1453 from the final model. (B) Assigned 1H-15N HSQC nuclear magnetic resonance (NMR) spectrum for TgGACPH (TgGAC2505–2639). (C) Superposition of the the 20 low energy structure of TgGACPH from the NMR ensemble. (D) Superposition of the Alphafold2 structure of TgGACPH with a representative NMR structure. (E) Structural TgGACPH coloured according to annotated secondary structure.
-
Figure 1—figure supplement 1—source data 1
Nuclear Overhauser effect (NOE) assignment, nuclear magnetic resonance (NMR) structure calculation data, PDB file for TgGAC PH domain.
- https://cdn.elifesciences.org/articles/86049/elife-86049-fig1-figsupp1-data1-v2.zip
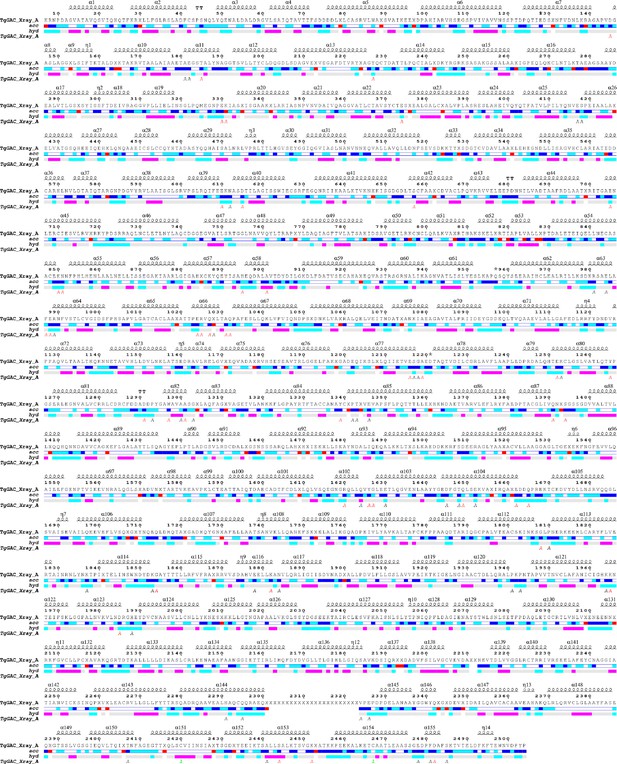
Secondary structure for the crystal structure of TgGAC.
Representation generated using ENDscript (Robert and Gouet, 2014). α-helices labelled α1, α2…, and α156, other helix types η1, η2…, and β-strands by their sheets β1... β7 and turn with TT.
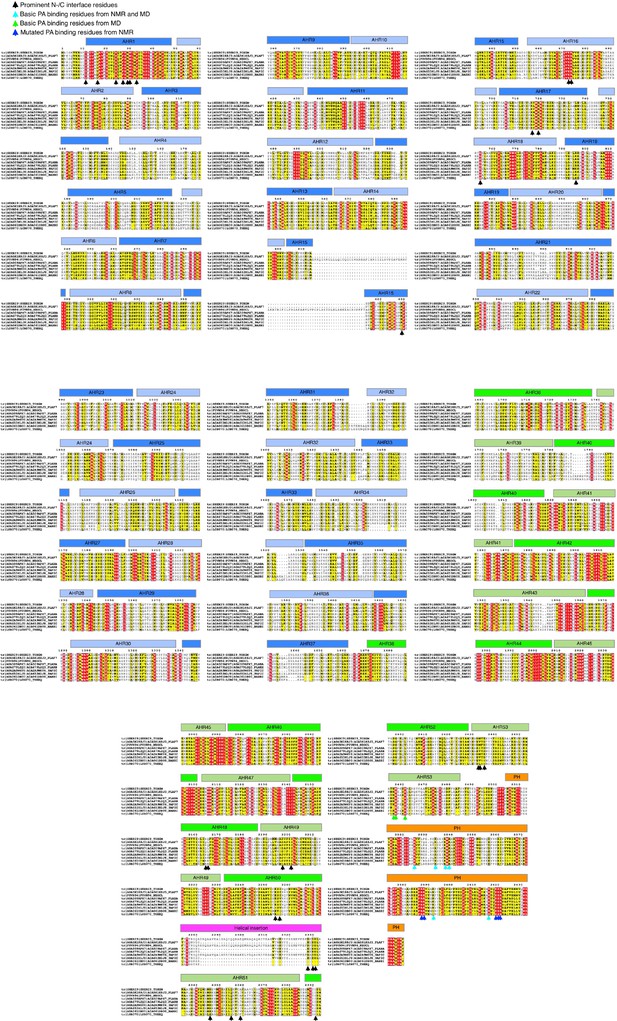
Structural homology with TgGAC.
Comparisons of the structure of the C-terminal armadillo (ARM)-rich arch of TgGAC with human p120 catenin/E-cadherin (PDB 3L6X), β-catenin//E-cadherin from Caenorhabditis elegans (PDB 4R10), human plakoglobin/E-cadherin (PDB 3IFQ) and UNC45 myosin chaperone Caenorhabditis elegans (PDB 6QDL). Orientations are chosen by superposing over equivalent ARMs.
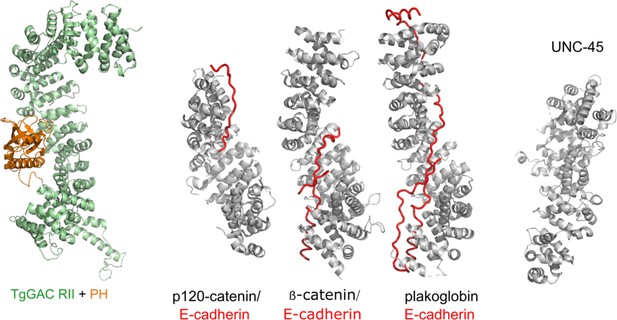
Sequence alignment for the crystal structure of TgGAC.
Sequence alignment of selected glideosome-associated connector (GAC) homologues with domain architecture and key interface, phosphatidic acid (PA) binding residues indicated.
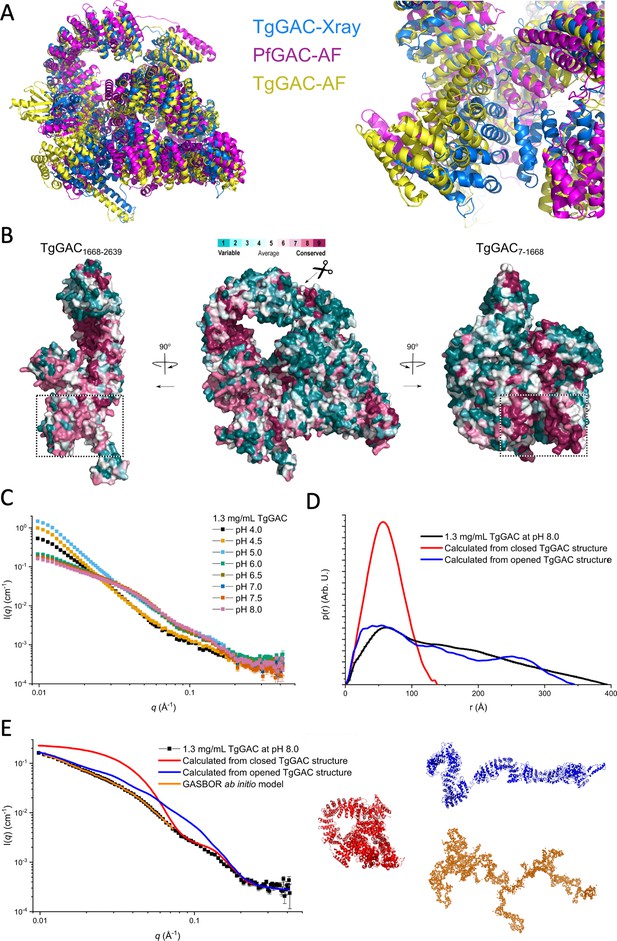
Glideosome-associated connector (GAC) adopts both open and closed structures.
(A) Superimposition of the experimentally determined composite structure of TgGAC with those generated from AlphaFold2 for TgGAC and PfGAC, showing that the closed structure is conserved (left). N/C-terminal interface is highly similar but partially opened in the predicted structures of TgGAC and PfGAC. The PH domain is not displayed for clarity (right). (B) Conservation profile on a surface representation for TgGAC in three different orientation generated using ConSurf (Landau et al., 2005). Centre – full-length TgGAC in the same conformation as (A). TgGAC is split in two and each half rotated 90° to display the N/C interface. C-terminal regions RII and RIII (left) and N-terminal region RI (right). The surface is coloured according to its ConSurf conservation score, which varies from cyan which are highly variable to maroon for highly conserved. (C) Small-angle X-ray scattering (SAXS) data for GAC at pH 4.0–8.0. (D) Pair distance distribution functions (p(r)) for GAC SAXS data at pH 8.0, calculated from full-length closed TgGAC structure and calculated for the opened structure generated by molecular dynamic (MD) simulations. (E) Modelling of TgGAC solution structure at pH 8.0, calculated theoretical scattering curves for the full-length closed TgGAC structure (red), and calculated for the opened full-length TgGAC structure (blue), and a GASBOR ab initio model (orange). Structures are shown in colours corresponding to those of the scattering curves.
-
Figure 2—source data 1
Raw small-angle X-ray scattering (SAXS) data for TgGAC.
- https://cdn.elifesciences.org/articles/86049/elife-86049-fig2-data1-v2.zip
-
Figure 2—source data 2
Movie of steered molecular dynamic (MD) trajectory generating an extend TgGAC conformation.
- https://cdn.elifesciences.org/articles/86049/elife-86049-fig2-data2-v2.zip
-
Figure 2—source data 3
Final extended full-length TgGAC after steered molecular dynamic (MD).
- https://cdn.elifesciences.org/articles/86049/elife-86049-fig2-data3-v2.zip
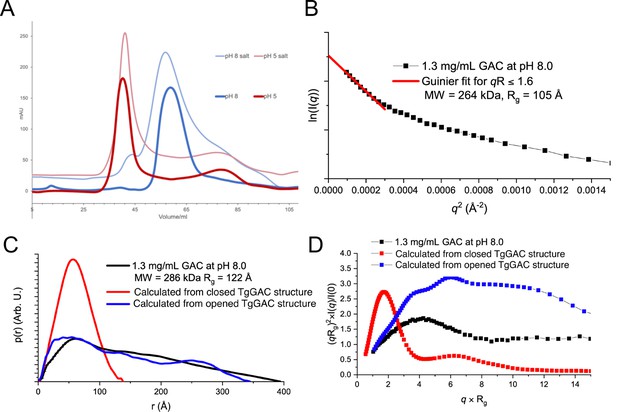
Size-exclusion chromatography of glideosome-associated connector (GAC) and analysis of small-angle X-ray scattering (SAXS) data at pH 8.0 with comparison to crystal structure.
(A) Size exclusion chromatography (SEC) profiles for 4 mg/ml TgGAC at pH 5 (25 mM sodium acetate) and pH 8 (25 mM Tris) in the presence and absence of 100 mM NaCl. (B) Guinier fit of TgGAC SAXS data using the first eight points. The molecular mass (MW) and radius of gyration (Rg) calculated from the Guinier fit are noted in the legend. (C) p(r) functions for TgGAC SAXS data (black), the full length closed TgGAC structure (red), and the molecular dynamics generated opened TgGAC structure (blue). The MW and Rg calculated from indirect Fourier transform (IFT) routine for SAXS data are noted in the legend. (D) Dimensionless Kratky plot for TgGAC SAXS data (black), the full length closed TgGAC structure (red), and the molecular dynamics generated opened TgGAC structure (blue).
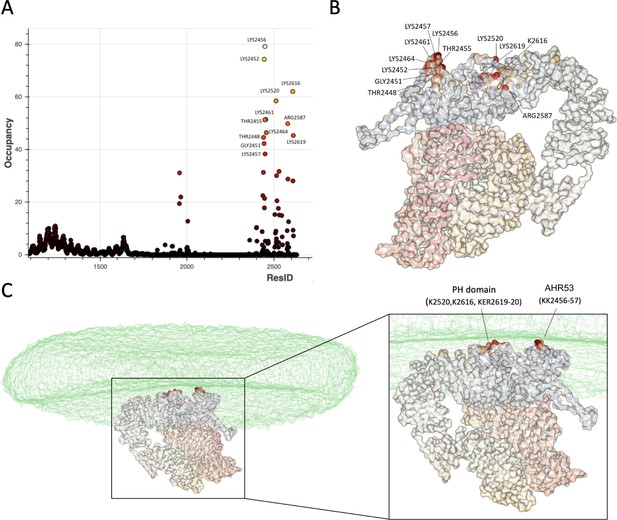
Simulation of TgGAC binding to phosphatidic acid (PA)-containing membranes.
(A) Analysis of residues that interact with 1-palmitoyl-2-oleoyl-sn-glycero-3-phosphate (POPA) as a percentage of contact during the simulation time. Analysis was performed for the final 5 µs of the simulation. (B) Key residues from mapped onto the TgGAC structure. (C) As in (B) in the contact of the membrane which is displayed as a transparent surface.
-
Figure 3—source data 1
Molecular dynamics trajectory analysis data for TgGAC.
- https://cdn.elifesciences.org/articles/86049/elife-86049-fig3-data1-v2.zip
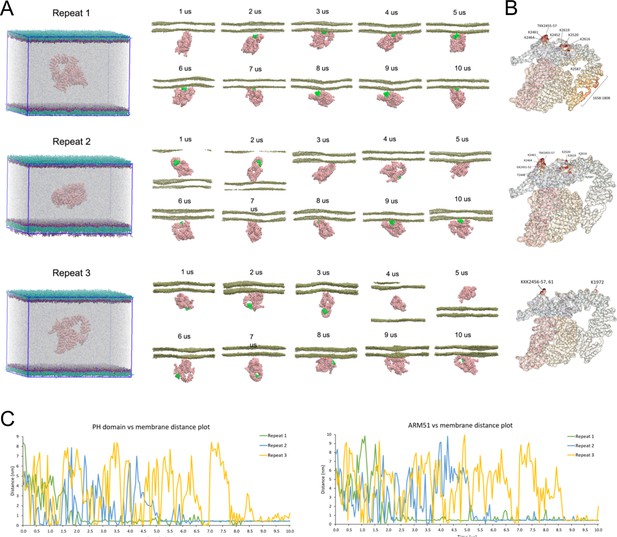
Molecular simulations of glideosome-associated connector (GAC) and phosphatidic acid (PA)-containing membranes.
(A) Binding series and initial orientations for each of three independently generated repeats. The initial configurations show GAC randomly orientated relative to the membrane patch, and the corresponding time series with snapshots at each microsecond are shown. Water is omitted for clarity. (B) Lipid contact analysis for the final microsecond of each trajectory. The heatmap and labelled residues show key regions in contact with POPA lipid. (C) Centre of mass distance over time for each simulation repeat for the PH domain (left hand panel) and the ARM51 region (right hand panel).
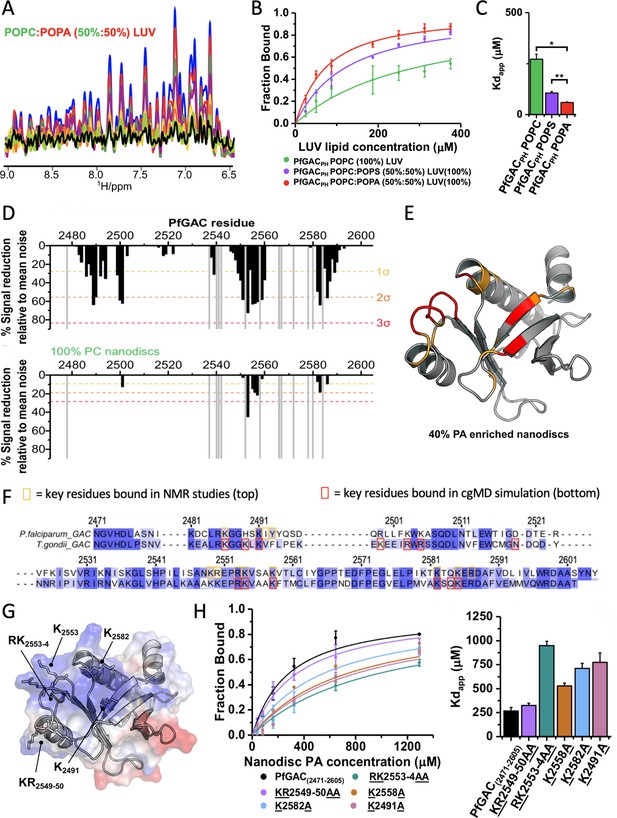
Nuclear magnetic resonance (NMR)-based binding assays for PfGAC2471–2605 (PfGACPH) to phosphatidic acid (PA)-enriched unilamellar liposomes.
(A) 1D 1H-NMR spectrum (9.4–6.5 ppm) of PfGACPH upon titration with increasing concentrations of large unilamellar vesicles (LUVs) composed of POPC:POPA (50:50%) LUV molar ratios: blue, free PfGACPH in solution; red 1:2; green 1:4; purple 1:7; yellow 1:15; orange 1:20; lime 1:25; black 1:30. (B) Binding curves generated from spectral integration and expressed as the fraction of bound protein for variable LUV compositions (POPC [100%] green, POPC:POPS [50:50%] purple, or POPC:POPA [50:50%] red). (C) Apparent dissociation constants (Kdapp) for binding LUVs were calculated from fitting binding curves. (D) Plot of PfGACPH paramagnetic relaxation enhancements (PREs) with PA-enriched MSP1D1H4-5 nanodiscs top (POPC:POPA:PE-DTPA-Gd3+46:40:14%) and bottom (POPC:PE-DTPA-Gd3+86:14%), against sequence number. Dashed lines represent 1 (yellow), 2 (orange), or 3 (red) SD from the mean noise (baseline). (E) PA PREs mapped onto the structure of PfGACPH, residues and coloured if greater than 2 (orange) or 3 (red). (F) Comparison of contact resides from molecular dynamic (MD) and NMR mapped on to the sequence alignment between PfGACPH and TgGACPH. (G) Electrostatic surface representation of PfGACPH revealing an extensive surface patch of positive charge surface charge with key mutated residues labelled. (H) Binding curves of PfGACPH mutants generated from 1D NMR titration with calculated Kdapp. Data are shown as mean of three replicates ±1σ. Apparent dissociation constants (Kdapp) were calculated from fitted binding curves. Data represent mean ±1σ for fitted curves.
-
Figure 4—source data 1
Raw and processed nuclear magnetic resonance (NMR) data for 1D small unilamellar vescicle (SUV) titration with PfGACPH.
- https://cdn.elifesciences.org/articles/86049/elife-86049-fig4-data1-v2.zip
-
Figure 4—source data 2
Raw and processed nuclear magnetic resonance (NMR) data for paramagnetic relaxation enhancement (PRE) measurements of PfGACPH with nanodiscs.
- https://cdn.elifesciences.org/articles/86049/elife-86049-fig4-data2-v2.zip
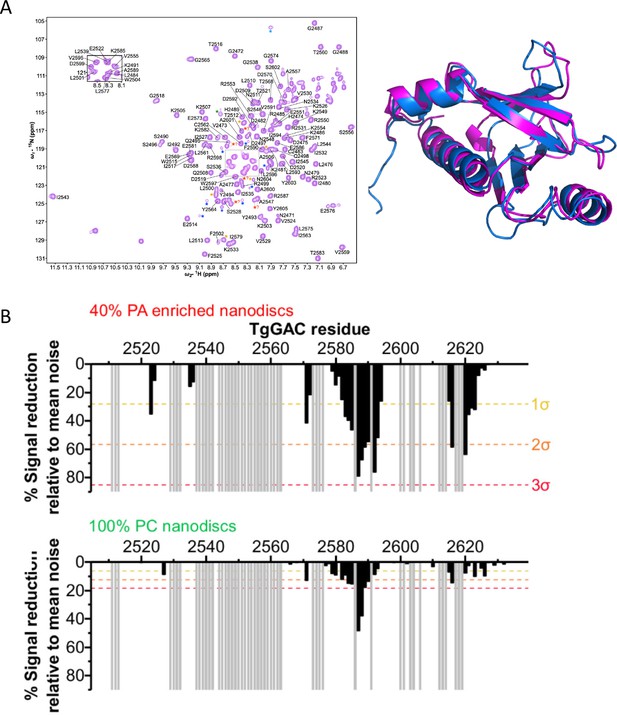
Nuclear magnetic resonance (NMR) assignments of PfGACPH and lipid binding paramagnetic relaxation enhancement (PRE) data for TgGACPH.
(A) Assigned 1H-15N HSQC for PfGACPH and comparison of validated structures for PfGACPH (magenta) and TgGACPH (blue). (B) PRE’s with phosphatidic acid (PA)-enriched (top) or phosphatidylcholine (PC) only MSP1D1H4-5 nanodiscs.
-
Figure 4—figure supplement 1—source data 1
Raw and processed nuclear magnetic resonance (NMR) data for SUV titration of TgGACPH.
- https://cdn.elifesciences.org/articles/86049/elife-86049-fig4-figsupp1-data1-v2.zip
-
Figure 4—figure supplement 1—source data 2
Raw and processed nuclear magnetic resonance (NMR) data for paramagnetic relaxation enhancement (PRE) measurements of TgGACPH with nanodiscs.
- https://cdn.elifesciences.org/articles/86049/elife-86049-fig4-figsupp1-data2-v2.zip
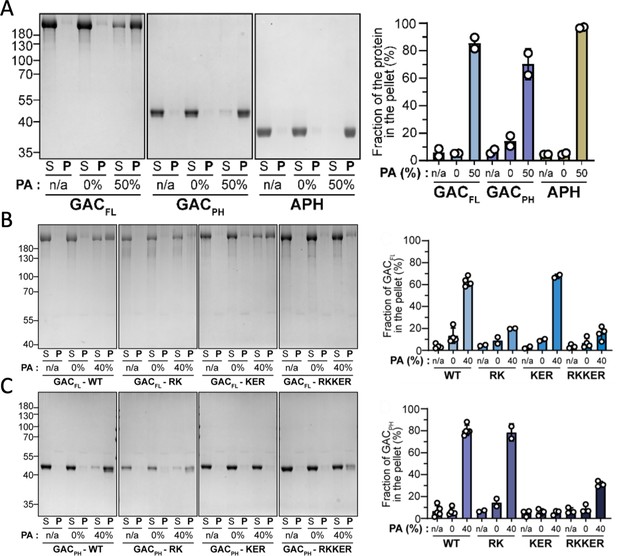
Liposome binding assays of phosphatidic acid (PA) binding by glideosome-associated connector (GAC) and PH domain mutants in vitro.
(A) Liposome binding assay with the three proteins of interest. A representative gel stained by Coomassie blue (left). Quantification of the pellet fraction measured by band densitometry (n=2). n/a=no liposome. S=supernatant fraction after ultracentrifugation. P=pellet fraction after centrifugation (right). (B) Representative gel stained by Coomassie blue from liposome binding assays with full-length TgGACFL and the three mutated versions (left). Quantification of the GACFL pellet fraction measured by band densitometry (n=2–4; right). n/a=no liposome. S=supernatant fraction after ultracentrifugation. P=pellet fraction after centrifugation. RK = RK/AA mutations. KER = KER/AAA mutations. RKKER = RK/AA +KER/AAA mutations. (C) Representative gel stained by Coomassie blue from liposome binding assays with TgGACPH and the three mutated versions (left). Quantification of the GACPH pellet fraction measured by band densitometry (n=2–4; right).
-
Figure 5—source data 1
Full, uncropped SDS-PAGE images for liposome binding experiments.
- https://cdn.elifesciences.org/articles/86049/elife-86049-fig5-data1-v2.zip
-
Figure 5—source data 2
Figure and caption for liposome binding assays using uncropped SDS-PAGE images with key bands labelled.
- https://cdn.elifesciences.org/articles/86049/elife-86049-fig5-data2-v2.pdf
-
Figure 5—source data 3
Liposome binding data tables.
- https://cdn.elifesciences.org/articles/86049/elife-86049-fig5-data3-v2.zip
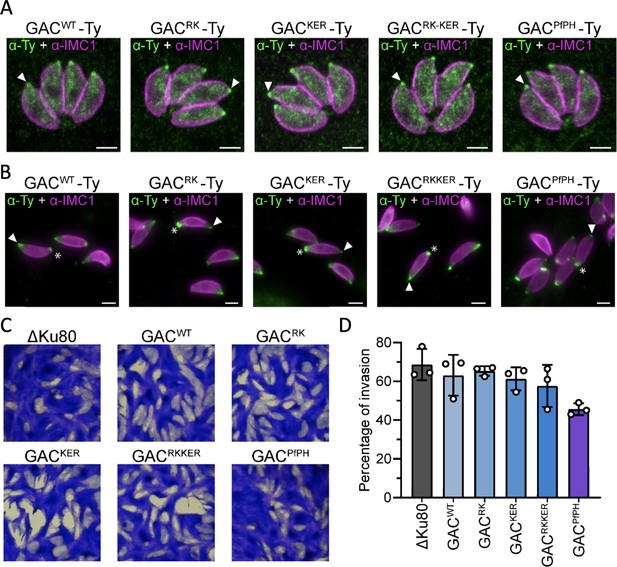
Phenotypical analysis of parasite bearing phosphatidic acid (PA)-binding mutations of glideosome-associated connector (GAC).
(A) GAC localisation by Immunofluorescence assay (IFA) in intracellular parasites. White arrow = apical pole. Scale bar = 2 µm. (B) GAC localisation by IFA in extracellular parasites. White arrow = apical pole. White star = basal pole. Scale bar = 2 µm. (C) Plaque assay of the different mutants analysed. (D) Red/green invasion assay (n=3). A Δku80 background T. gondii strain is used, which is deficient in nonhomologous end joining and provides highly efficient gene replacement.
-
Figure 6—source data 1
Parasite invasion data tables.
- https://cdn.elifesciences.org/articles/86049/elife-86049-fig6-data1-v2.zip
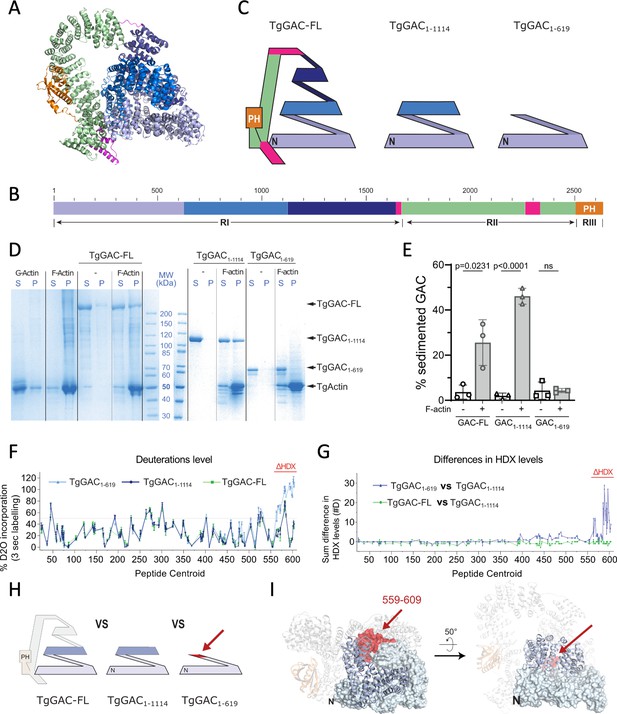
TgGAC interaction with TgActin.
(A) Cartoon representation of the structure of TgGAC coloured according to schematic representation shown in (B). (C) Schematic representation of TgGAC structure coloured according to panel (B) with illustration of the truncated recombinant TgGAC constructs. (D) Coomassie-stained gel analysing proteins remaining in supernatant or sedimenting upon centrifugation at 100,000 g. S: Supernatant. P: Pellet. (E) Graphical representation of the percentage of TgGAC co-sedimenting alone or in the presence of TgActin filaments. Data are mean + SD of three independent experiments. p Value calculated using unpaired t-test. (F) Graph displaying deuteration levels for TgGAC1–619, TgGAC1–1114, and TgGAC-FL. Each dot represents a single peptide where the deuteration level in percentage maximal deuteration is plotted according to the residue number at the centre of the peptide (peptide centroid). (G) Comparison of H/D exchange levels for peptides spanning glideosome-associated connector (GAC) residues 1–619 between (i) TgGAC 1–619 and TgGAC1–1114 (blue dots) and between (ii) TgGAC-FL and TgGAC1–1114 (green). Each dot represents a peptide with the x-axis indicating peptide centroid as in (F). The y-axis indicates the sum of differences in number of deuterons incorporated for each peptide, measured at three deuteration times: 3, 30, and 300 s. Region showing significant differences between TgGAC1–619 and TgGAC1–1114 is indicated in red. (H) Schematic representation of the three GAC constructs studied by hydrogen/deuterium exchange coupled to mass spectrometry (HDX-MS), focussing on the region coloured in light blue (residues 1–619). Red colouring indicates the region where a difference in H/D exchange rate is observed. (I) Structure of TgGAC with fragment 1–619 shown as surface representation and highlighted in light blue. Residues 620–1114 are shown in blue, and the remaining of GAC structure is shown in light white. Region displaying a different conformation in TgGAC1–619 compared to other TgGAC constructs, with increased H/D exchange rate, is coloured in red.
-
Figure 7—source data 1
Full, uncropped SDS-PAGE images for F-actin binding experiments.
- https://cdn.elifesciences.org/articles/86049/elife-86049-fig7-data1-v2.zip
-
Figure 7—source data 2
Figure and caption for uncropped gels of F-actin binding experiments.
- https://cdn.elifesciences.org/articles/86049/elife-86049-fig7-data2-v2.pdf
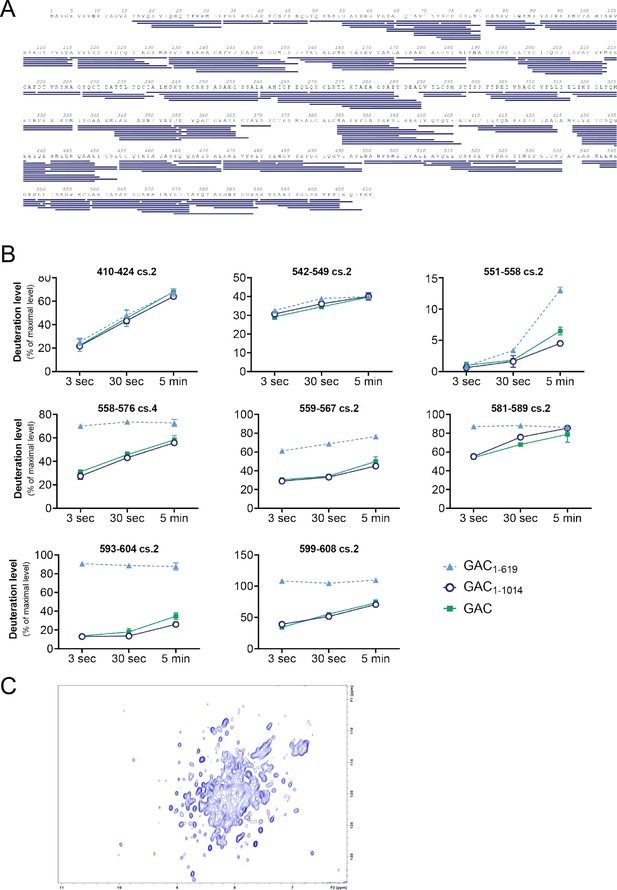
Hydrogen/deuterium exchange coupled to mass spectrometry (HDX-MS) and nuclear magnetic resonance (NMR) data for TgGAC1–619 construct studied.
(A) Peptide map showing all peptides analysed in for TgGAC1–619. (B) Uptake plots for a selection of peptides. (C) 1H-15N HSQC NMR spectrum of TgGAC1–619 showing a well-folded protein.

Production and purification of recombinant GAC-FL and GAC-∆PH.
Both GAC contract constructs are well expressed in BL-21 bacteria, showing an intense band at the expected MW. Purification using a combination of affinity, anion-exchange and size-exclusion chromatography (SEC) shows excellent result for GAC-FL. Although some protein elultes in the void volume 8ml on Superdex200 SEC, most of GAC-FL comes off the column as a symmetrical peak at 9.7ml suggested of monomeric protein. Purification of GAC-∆PH following a similar procedure as for GAC-FL shows many degradation products and absence of the intact protein on the SEC column. The purifications described here are representative of several attempts with no GAC-∆PH protein that could be isolated. The results indicate that C-terminal PH domain is essential for recombinant GAC stability, with removal of the C-terminal PH domain leading to degradation of GAC.
Tables
Data collection, phasing, and refinement statistics.
TgGAC | |||
---|---|---|---|
Space group | P 21 21 21 | ||
Cell dimensions | |||
a, b, and c (Å) | 119.078, 123.605, and 221.508 | ||
α, β, and γ (°) | 90, 90, and 90 | ||
Peak | Edge | Remote | |
Wavelength (Å) | 0.9795 | 0.9796 | 0.9722 |
Resolution range (Å) | 110.75–2.67 (2.72–2.67) | 110.75–2.67 (2.72–2.67) | 110.75–2.67 (2.72–2.67) |
Total number of reflections | 904,519 | 750,918 | 798,669 |
Unique reflections | 92,922 | 76,602 | 81,143 |
Rpim | 0.036 (0.48) | 0.032 (0.517) | 0.031 (0.504) |
I/σI | 14.00 (0.8) | 15.8 (0.73) | 14.70 (0.71) |
Completeness (%) | 100 (100) | 100 (100) | 100 (100) |
Multiplicity | 9.7 (9.7) | 9.7 (10.7) | 9.8 (10.3) |
R-merge | 0.108 (1.627) | 0.096 (1.64) | 0.093 (1.44) |
R-meas | 0.114 (1.698) | 0.101 (1.72) | 0.098 (1.528) |
CC1/2 | 0.99 (0.58) | 0.999 (0.57) | 0.99 (0.65) |
Refinement statistics | |||
No. of reflections for refinement | 92,300 (9069) | ||
No. of reflections for Rfree | 4502 (409) | ||
Rwork | 0.2095 (0.4036) | ||
Rfree | 0.2683 (0.3990) | ||
CC (work) | 0.904 (0.508) | ||
CC(free) | 0.910 (0.440) | ||
No. of non-hydrogen atoms | 18,793 | ||
Macromolecules | 18,679 | ||
Solvent | 114 | ||
Ramachandran favoured/allowed/outliers (%) | 93.4/6.1/0.45 | ||
Average B-factor | 76.88 | ||
Macromolecules | 70.92 | ||
Solvent | 70.07 | ||
Rms bond lengths (Å) | 0.009 | ||
Rms bond angles (°) | 1.47 | ||
Clashscore | 17.98 |
-
Values in parentheses are for highest-resolution shell.
-
Data from a single crystal were used to solve the structure.
Nuclear magnetic resonance (NMR) structure calculation statistics.
20 lowest energy structures | TgGACPH |
---|---|
Assigned nuclear Overhauser effect (NOE) peaks | |
Intra-residue | 1648 |
Medium range (|i – j|)≤4 | 640 |
Long range (|i – j|)>4 | 1260 |
Total | 3548 |
Average CYANA target function value | 12.4 |
Ramachandran plot | |
% in most favoured positions | 79.7±0.7 |
% in allowed regions | 20.2±0.4 |
% in generously allowed | 0.2±0.1 |
% in disallowed regions | 0.0±0.0 |
Atomic coordinates | |
Pairwise backbone RMSD secondary structures (Å) | 0.17±0.01 |
Additional files
-
Supplementary file 1
Table of deuterium incorporation for each of the selected peptides used for hydrogen/deuterium exchange coupled to mass spectrometry (HDX-MS) analysis are presented.
Results are shown both as percentage deuteration compared to a theoretical maximal deuteration level and as number of deuterons incorporated into the peptide (#D).
- https://cdn.elifesciences.org/articles/86049/elife-86049-supp1-v2.xlsx
-
Supplementary file 2
Table of differences in deuterium uptake between two states.
For each state comparison, results are shown for both differences in deuterium uptake by percentage (%D) and by number of deuterons (#D). Largest differences are highlighted in different colours: blue indicates PROTECTION of the peptide, and orange indicates increased EXPOSURE (usually associated with allosteric modifications).
- https://cdn.elifesciences.org/articles/86049/elife-86049-supp2-v2.xlsx
-
Supplementary file 3
Table for hydrogen/deuterium exchange (HDX) experimental details.
- https://cdn.elifesciences.org/articles/86049/elife-86049-supp3-v2.xlsx
-
Supplementary file 4
Key DNA primers used generate mutations and the endogenous locus mutants for parasite experiments.
- https://cdn.elifesciences.org/articles/86049/elife-86049-supp4-v2.docx
-
MDAR checklist
- https://cdn.elifesciences.org/articles/86049/elife-86049-mdarchecklist1-v2.docx