Adipocyte microRNA-802 promotes adipose tissue inflammation and insulin resistance by modulating macrophages in obesity
Abstract
Adipose tissue inflammation is now considered to be a key process underlying metabolic diseases in obese individuals. However, it remains unclear how adipose inflammation is initiated and maintained or the mechanism by which inflammation develops. We found that microRNA-802 (Mir802) expression in adipose tissue is progressively increased with the development of dietary obesity in obese mice and humans. The increasing trend of Mir802 preceded the accumulation of macrophages. Adipose tissue-specific knockout of Mir802 lowered macrophage infiltration and ameliorated systemic insulin resistance. Conversely, the specific overexpression of Mir802 in adipose tissue aggravated adipose inflammation in mice fed a high-fat diet. Mechanistically, Mir802 activates noncanonical and canonical NF-κB pathways by targeting its negative regulator, TRAF3. Next, NF-κB orchestrated the expression of chemokines and SREBP1, leading to strong recruitment and M1-like polarization of macrophages. Our findings indicate that Mir802 endows adipose tissue with the ability to recruit and polarize macrophages, which underscores Mir802 as an innovative and attractive candidate for miRNA-based immune therapy for adipose inflammation.
Editor's evaluation
This important study utilizes a comprehensive array of animal and cellular models, alongside various techniques, to elucidate the mechanism by which adipose tissue miR-802 contributes to inflammation and metabolic dysfunction in obesity. The data are compelling, with consistent findings across replicates and different models. The work will be of interest to medical biologists working on obesity and metabolic diseases.
https://doi.org/10.7554/eLife.99162.sa0Introduction
Obesity is a very powerful health determinant or indicator that facilitates the development and progression of several metabolic diseases, including insulin resistance and type 2 diabetes (Ling and Rönn, 2019; Klein et al., 2022). Adipose tissue is a highly dynamic metabolic organ that plays a central role in the regulation of energy homeostasis and controls glucose metabolism and insulin sensitivity (Scherer, 2006; Kang et al., 2008). A hallmark of obesity is low-grade chronic inflammation in adipose tissue, characterized by the accumulation of macrophages and other immune cells, and by an increase in the levels of pro-inflammatory cytokines (Pellegrinelli et al., 2022; Hägglöf et al., 2022; Kratz et al., 2014). Persistent adipose tissue inflammation is now considered to have a pivotal role in obesity-associated insulin resistance (Kohlgruber and Lynch, 2015; Burhans et al., 2018). Resetting the immunological balance in obesity could represent an innovative approach for the management of insulin resistance and diabetes (Brestoff et al., 2021; Lee et al., 2016). However, the early triggers and signals that sustain adipose tissue inflammation in obesity remain elusive, limiting our ability to effectively intervene this growing public health issue.
Macrophages accumulate in the adipose tissue of obese mice and humans, where they form crown-like structures surrounding dying or dead adipocytes and are key contributors to inflammation and obesity-induced insulin resistance (Weisberg et al., 2003; Hotamisligil, 2006). The number of adipose tissue macrophages is tightly linked to the degree of insulin resistance and metabolic dysregulation (Xu et al., 2003; Chawla et al., 2011). Ablation of pro-inflammatory adipose tissue macrophages leads to a rapid improvement in insulin sensitivity and glucose tolerance, associated with marked decreases in local and systemic inflammation in obese mice (Patsouris et al., 2008; Nomiyama et al., 2007). Targeting the major inflammatory pathways is sufficient to counteract obesity-related systemic inflammation and insulin resistance (Arkan et al., 2005; Patra et al., 2023). However, the molecular links between lipid-overloaded adipocytes and inflammatory macrophages in obese adipose tissue remain elusive.
MicroRNAs (miRNAs) are small non-coding RNAs that post-transcriptionally regulate gene expression by binding to specific regions of target genes to prevent translation or promote mRNA degradation (Ambros, 2004). Emerging evidence suggests that miRNAs are key regulators in a variety of important metabolic organs and substantial contributors to the pathogenesis of complex diseases, including obesity-associated metabolic diseases (Dumortier et al., 2013; Krützfeldt and Stoffel, 2006). In the adipose tissue, miRNAs have dramatic effects on regulating the pathways that control a range of processes including lipogenesis, inflammation, and insulin signaling (Arner and Kulyté, 2015; Thomou et al., 2017). Moreover, mice with alterations in the levels of miRNAs in adipocytes show significantly enhanced inflammation and insulin resistance after feeding with a high-fat diet (HFD), further confirming the contribution of miRNAs to obesity-induced phenotypes (Agbu and Carthew, 2021; Koh et al., 2018). Therefore, adipose-derived miRNAs hold great promise for understanding adipose tissue dysfunction and the relationship between chronic inflammation and obesity and insulin resistance.
In this study, we demonstrated that microRNA-802 (Mir802) promotes inter-cellular communication between lipid-overloaded adipocytes and macrophages, ultimately leading to adipose tissue inflammation and insulin resistance. Adipocyte Mir802 levels are positively associated with obesity in mice and humans. Adipose tissue-specific overexpression of Mir802 in mice fed an HFD exhibited increased severity of systemic insulin resistance compared with wild-type (WT) mice, which was accompanied by macrophage infiltration and a marked increase in adipose tissue inflammation. Adipose tissue-specific knockout of Mir802 achieved the opposite result. Co-culture and other in vitro experiments revealed a vicious cycle of interactions between macrophages and adipocytes ectopically expressing Mir802. We established that Mir802 expression is an inflammatory signal in adipocytes, and this effect occurs through sensitization of the NF-κB signaling pathway. Altogether, our data raise the possibility that manipulation of this microRNA action axis has therapeutic potential for treating adipose inflammation.
Results
Mir802 elevation precedes macrophage accumulation
Consistent with previous studies from our and other laboratories (Zhang et al., 2020; Kornfeld et al., 2013), adipose from obese mice showed significantly higher Mir802 expression than those from normal mice. To evaluate whether Mir802 is involved in adipose inflammation and insulin resistance. We examined the expression profile of Mir802. We observed that Mir802 progressively increased in adipose tissue from week 4 with the development of obesity in mouse models of genetic and dietary obesity (Figure 1A, B, Figure 1—figure supplement 1A). We next compared the expression of Mir802 in different adipose depots and found that it was the highest in epididymal white adipose tissue (epiWAT; Figure 1C). We further isolated mature adipose tissue and stromal vascular fraction (SVF) from epiWAT to examine the expression of Mir802. We found that Mir802 expression was substantially higher in mature adipocytes than in SVF in both mice fed a normal chow diet (NCD) and those fed an HFD (Figure 1D). Through in vitro experiments, we found that Mir802 was dramatically increased in the insulin resistance cell models (Figure 1E, F, Figure 1—figure supplement 1C, D). We have determined that the upregulation of islet Mir802 during obesity is mediated by Forkhead box O1 (FoxO1; Zhang et al., 2020). FoxO1 is predominantly expressed in WAT and is upregulated in WAT of the obese mice (Teaney and Cyr, 2023). Here, we found that ADA-FoxO1 exhibited significantly the higher binding ability of FoxO1 to Mir802 promoter compared to control via ChIP assay (Figure 1G) and over-expression FoxO1 upregulated Mir802 expression (Figure 1H). These findings suggest that obesity induced Mir802 expression via FoxO1 and upregulation of Mir802 in adipocytes may be functionally involved in the pathogenesis of obesity-associated disorders.
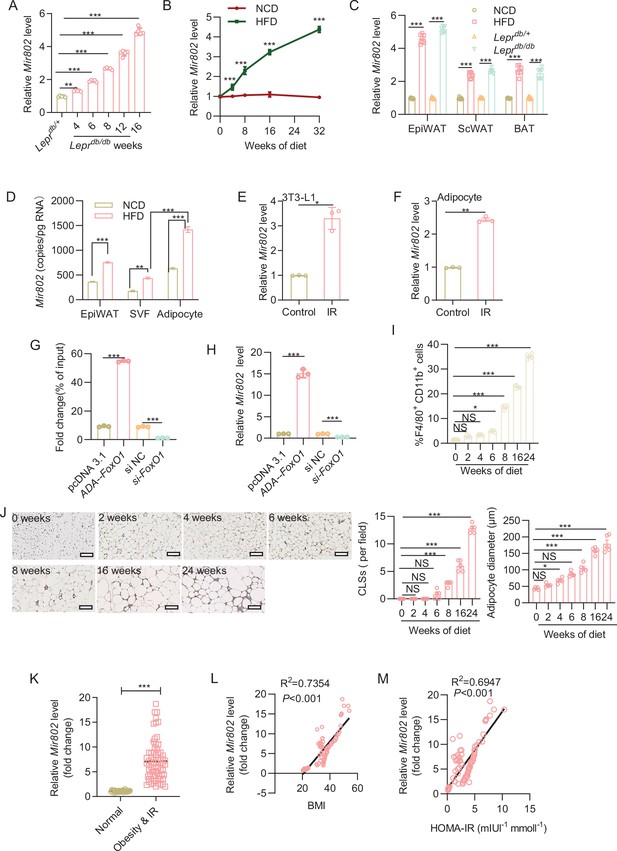
Obesity induced Mir802 elevation precedes macrophage accumulation.
(A) mRNA abundance of Mir802 in the epiWAT of Leprdb/db or control mice at 4, 6, 8, 12, and 16 weeks (n=5). (B) mRNA abundance of Mir802 in the epiWAT of mice fed a normal chow diet (NCD) or HFD for 0, 4, 8, 16, 24, and 32 weeks (n=5). (C) The expression level of Mir802 in epiWAT, scWAT and BAT isolated from mice on HFD for 16 weeks or 10 weeks Leprdb/db mice (n=7). (D) Copy number of Mir802 in mature adipocytes and stromal vascular fraction (SVF) of epiWAT isolated from mice on NCD or HFD for 16 weeks (n=5). (E–F) Mir802 expression levels in insulin resistance 3T3-L1 cell models (E) and insulin resistance WAT SVF cells models (F). (G) ChIP assays was performed to test the binding ability between FoxO1 and Mir802 promoter. (H) Mir802 expression levels in the 3T3-L1 cells transfected with ADA-FoxO1 or FoxO1 siRNA. (I) F4/80 and CD11b positive cells in SVFs isolated from the epiWAT of mice fed an HFD for 0, 2, 4, 6, 8, 16, and 24 weeks (n=5). (J) Representative images of F4/80 staining (left) and quantification of crown-like structures (CLSs; middle) and adipose diameter (Right) in the epiWAT of mice fed an HFD (n=5). (K) Expression levels of Mir802 in human subcutaneous adipose tissue (nnormal = 25, nobesity & IR=70). Scatter plots of Mir802 expression versus BMI (L) and HOMA-IR (M). Pearson’s correlation coefficients (r) are shown. The fold of Mir802 was calculated using 2-ΔΔCt. Data represent mean ± SEM. p-values obtained using a two-tailed unpaired Student’s t-test (E, F, K) or two-way ANOVA (A–D, G–J) are indicated. *p<0.05, **p<0.01, ***p<0.001. Relative levels of Mir802 were normalized to U6. epiWAT: epididymal white adipose tissue, scWAT: subcutaneous white adipose tissue, BAT: brown adipose tissue.
Initial studies have indicated that macrophages are responsible for most inflammatory events in adipose tissue (Weisberg et al., 2003; Hotamisligil, 2006). However, what initiates macrophage infiltration or the resultant inflammatory cascade is still not well defined. We hypothesized that the elevation of Mir802 in adipocytes is associated with adipose inflammation and insulin resistance. To evaluate this hypothesis, we analyzed the relationship between Mir802 elevation and macrophage infiltration during the progression of diet-induced obesity (DIO). We first carried out a set of flow cytometric analyses to determine the dynamic alterations of macrophages in collagenase-digested SVF from epiWAT. Starting from 8 week, there was a gradual and continuous increase in the number of CD11b/F4/80 double-positive macrophages observed in obese mice (Figure 1I, Figure 1—figure supplement 1E). Immunohistochemical analysis of F4/80 expression also revealed that the number of macrophages continued to increase in the epididymal fat pads of obese mice as compared to that in mice on a normal diet (Figure 1J). The dynamic increase in Mir802 preceded the infiltration of macrophages, indicating that Mir802 may play a critical role in the occurrence of adipose inflammation.
To gain additional insight into the clinical importance of Mir802 in obese fat, we analyzed the expression of Mir802 in samples of human subcutaneous adipose tissue. Levels of Mir802 expression were significantly higher in obese subjects (body mass index [BMI]=38.30 ± 5.82 kg/m2, fasting plasma glucose = 8.39 ± 1.54 mM, homeostatic model assessment for insulin resistance [HOMA-IR]=3.77 ± 1.97) than in lean ones (BMI = 22.09 ± 1.09 kg/m2, fasting plasma glucose = 4.84 ± 0.53 mM, HOMA-IR=0.21 ± 0.06; Figure 1K, Figure 1—figure supplement 1F). Pearson’s correlation analysis showed that the BMI and HOMA-IR were positively associated with Mir802 abundance in subcutaneous fat (Figure 1L and M). The same phenomenon was also observed in RNA-FISH analysis (Figure 1—figure supplement 1G), indicating that upregulation of Mir802 in the adipose tissue during obesity is conserved in humans.
Adipose-selective overexpression of Mir802 aggravates inflammatory cascade in obese mice
To further assess the role of adipocyte Mir802, we generated adipose-selective Mir802 konck-in (Mir802 KI) mice by crossing Mir802ki/ki mice (Zhang et al., 2020) with animals expressing Cre recombinase under the control of the promoter of Adiponectin (Figure 2—figure supplement 1A, B). Real-time PCR analysis confirmed that the overexpression of Mir802 was restricted in the adipose tissues of the Mir802 KI mice, Mir802 expressions was up-regulated about 150 times, whereas its expression in other organs was not affected except for BAT (Figure 2—figure supplement 1C), and the upregulation of Mir802 was limited to adipocytes and was not observed in SVFs (Figure 2—figure supplement 1D). There was no obvious difference in food intake, body weight, glucose content, and adiposity between Mir802 KI mice and their WT littermates in both male and female when they were fed with NCD (Figure 2—figure supplement 1E–H). We then fed the mice an HFD and performed metabolic and histological analyses. We detected the presence of adipose inflammation, typified by macrophage crown-like structures (CLSs) in epiWAT at 8 weeks in Mir802 KI mice, which was earlier than their WT littermates, and the number of CLSs was almost doubled at 16 weeks (Figure 2A). No significant differences were observed in CLSs between the control and Mir802 KI mouse groups treated with normal chow diet (NCD, Figure 2—figure supplement 1I). Consistently, flow cytometric analysis showed that HFD-induced elevation in the number of CD11b+F4/80+ macrophages in the SVF of epiWAT in adipose-specific Mir802 KI mice was significantly higher than that in WT littermates in both male and female (Figure 2B, Figure 2—figure supplement 1J). In Mir802 KI mice fed on HFD for 16 weeks, the number of classically activated proinflammatory M1 macrophages (defined as CD86+CD206-) was significantly higher than that of alternatively activated anti-inflammatory M2 macrophages (defined as CD86-CD206+) in epiWAT (Figure 2C, Figure 2—figure supplement 1K). In line with this finding, epiWAT from dietary-obese Mir802 KI mice exhibited obviously higher mRNA expression of the M1 macrophage–related genes (Ccl2, Il1b, Il6, Tnfa, Inos, and Ifng) but significant reductions of M2 macrophage–related genes (Il10, Chil3, Arg1, and Fizz1; Figure 2D). Similarly, HFD also increased the level of several inflammatory factors (chemokine ligand 2 [CCL2], interleukin IL-6, IL-1β, and tumor necrosis factor TNF-α) in the serum of Mir802 KI mice (Figure 2E–H).
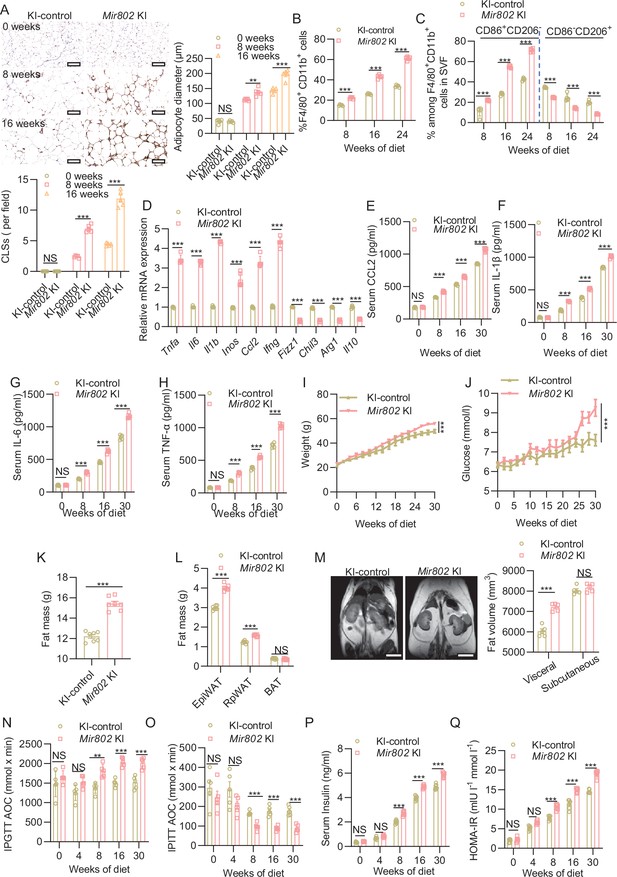
Adipose tissue-specific overexpression of Mir802 exacerbates adipose tissue inflammation and leads to metabolic dysfunction.
(A) Representative images of F4/80 staining (top), quantification of CLSs (bottom) and adipose diameter (right) in epiWAT of WT or Mir802 KI mice on HFD for 0, 8, and 16 weeks (n=5). Scale bar: 40 μm. (B) Percentage of F4/80+/CD11b+ total macrophages in the epiWAT of Mir802 KI and KI-control mice fed with HFD (n=5). (C) M1 (CD86+CD206–) and M2 (CD206+CD86–) within the macrophage population (n=5). (D) qRT-PCR analysis for mRNA levels of the M1 and M2 markers in the epiWAT of mice on KI-control or Mir802 KI at 16 weeks (n=5). (E–H) Serum levels of CCL2 (E), IL-1β (F), IL-6 (G), and TNF-α (H) of Mir802 KI and control mice fed with HFD for 0, 8, 16, and 30 weeks (n=5). (I, J) Dynamic changes in body weight (I) and glucose (J) in WT and Mir802 KI mice during 30 weeks of HFD feeding (n=5). (K, L) Fat mass of whole body (K) and individual tissues (L) (n=7). (M) Representative coronal section MRI images and visceral and subcutaneous adipose tissue volume of HFD-fed control and Mir802 KI mice (n=5). (N, O) Area over the curve (AOC) of the blood glucose level was calculated via intraperitoneal glucose tolerance tests (IPGTTs, 2 g/kg, N, n=5) or intraperitoneal insulin tolerance tests (IPITTs; 0.75 U/kg, O, n=5). (P) Fasting insulin (FINS) levels of HFD-fed mice were measured by ELISA (n=7). (Q) HOMA-IR was calculated with the equation FBG (mmol l−1)×FINS (mIU l−1)/22.5. Data represent mean ± SEM. Differences between groups were determined by ANOVA (A–J, L, and N–Q) or two-tailed unpaired Student’s t-test (K). *p<0.05, ***p<0.001. Gene levels were normalized to Rn18s abundance.
We next explored whether the aggravation of adipose inflammation in adipose-selective Mir802 KI mice in both male and female were associated with exacerbation of metabolism and insulin sensitivity. We found that in Mir802 KI mice, HFD induced weight gain (Figure 2I, Figure 2—figure supplement 2A) and hyperglycemia (Figure 2J) both in male and female. HFD also induced adiposity in Mir802 KI mice, which mainly manifested in the expansion of visceral WAT (Figure 2K and L). MRI analysis confirmed that HFD induced an increase in visceral WAT in Mir802 KI mice (Figure 2M). We next monitored the dynamic changes in insulin sensitivity at different time points (0, 4, 8, 16, and 30 weeks) after feeding the two groups of mice with an HFD. As expected, Mir802 KI mice on a HFD exhibited progressive development of glucose intolerance (Figure 2N, Figure 2—figure supplement 2B-F) and insulin resistance (Figure 2O, Figure 2—figure supplement 2G-K) at 8 weeks, as compared to their WT littermates. These differences became even more obvious after 16 and 30 weeks, coupled with an increase in fasting insulin levels (Figure 2P) and HOMA-IR (Figure 2Q). Collectively, these effects of adipose-selective overexpression of Mir802 show that Mir802 is sufficient for the recruitment of macrophages into obese adipose tissue and for the initiation and propagation of the inflammatory cascade.
Mir802 depletion ameliorates obesity-induced metabolic dysfunction
Given the striking effects of adipose-selective overexpression of Mir802 on metabolism, we next investigated whether selectively ablated Mir802 in adipose tissue could mitigate metabolic disturbance and inflammation induced by obesity. We generated Mir802 conditional knockout mice using the Cre/Lox system (Figure 3—figure supplement 1A). Mir802fl/fl were crossed with Adipoq-Cre transgenic animals to selectively ablate Mir802 in adipose tissues (Figure 3—figure supplement 1B). Expression analysis showed that total Mir802 levels were reduced by approximately 70% in the adipose tissue but not in SVFs of Mir802 KO mice compared with WT littermates (Figure 3—figure supplement 1C, D). The knockout of Mir802 in adipose tissue did not alter food intake, body weight, glucose levels, and adiposity compared with their WT littermates in both males and females when they were fed with NCD (Figure 3—figure supplement 1E–I); however, this approach could prevent HFD-induced weight gain and hyperglycemia (Figure 3A, B, Figure 3—figure supplement 1J). Adipose-selective ablation of Mir802 also alleviated HFD-induced adiposity, mainly by reducing the expansion of visceral WAT, including epiWAT and retro-peritoneal WAT (Figure 3C and D). MRI analysis confirmed this result (Figure 3E). Histological and FACS analysis showed that Mir802 depletion reduced macrophage infiltration, which mainly manifested as a decrease in the number of CLSs and macrophages (Figure 3F, G, Figure 3—figure supplement 1K), while there was minimal difference observed between the two groups fed with NCD (Figure 3—figure supplement 1L). Notably, the Mir802 KO mice exhibited obvious reductions in mRNA expression of the M1 macrophage-related genes (Ccl2, Il1b, Il6, Tnfa, Inos, and Ifng) but significant upregulation of M2 macrophage-related genes (Fizz1, Chil3, Arg1, and Il10; Figure 3H). The Mir802 KO mice also markedly blunted HFD-induced elevation in serum levels of several inflammatory factors (TNF-α, IL-6, IL-1β, and CCL2; Figure 3I). In addition, the insulin resistance and glucose intolerance induced by an HFD were ameliorated by Mir802 depletion (Figure 3J, R, Figure 3—figure supplement 1M-R). These phenomena were the same both in male and female Mir802 KO mice.
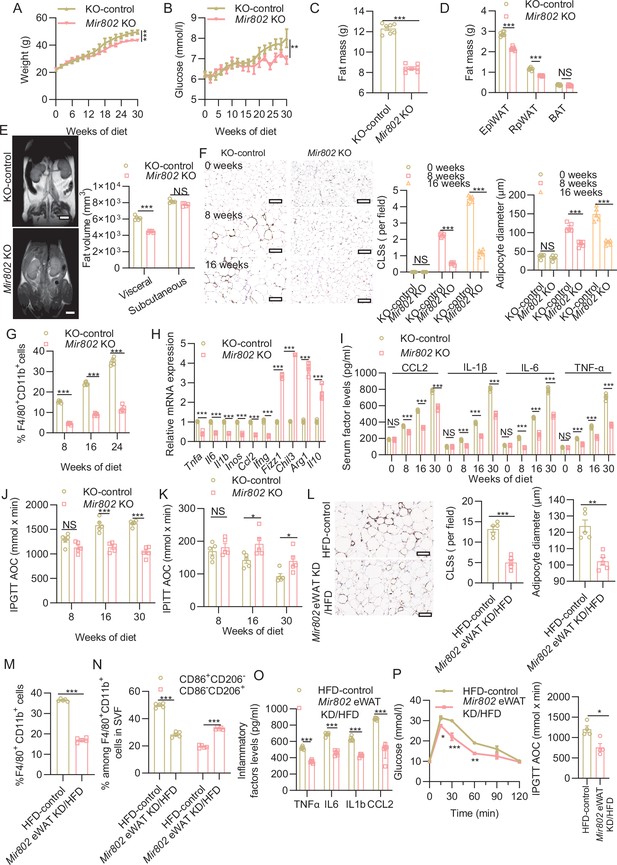
Adipose tissue–specific ablation of Mir802 protects mice from obesity-induced metabolic dysfunction.
(A–B) Dynamic changes in body weight (A) and glucose (B) of KO control and Mir802 KO mice during 30 weeks of HFD feeding (n=7). (C–D) Fat mass of whole body (C) and individual tissues (D) (n=7). (E) Representative coronal section MRI images and visceral and subcutaneous adipose tissue volume of HFD-fed control and Mir802 KO mice (n=5). (F) Representative images of F4/80 staining (left), quantification of CLSs (middle) and adipose diameter (right) in epiWAT of WT or Mir802 KO mice on HFD for 0, 8, and 16 weeks (n=5). Scale bar: 40 μm. (G) Cells isolated from SVFs of epiWAT in Mir802 KO and WT mice fed with HFD for 8, 16, and 24 weeks were subjected to flow cytometry analysis for percentage of CD11b+/F4/80+ total macrophages (n=5). (H) qRT-PCR analysis for the mRNA levels of the M1 and M2 markers in epiWAT of mice on HFD 16 weeks (n=5). (I) Serum levels of CCL2, IL-1β, IL-6, TNF-α determined with ELISA (n=5). (J–K) AOC of the blood glucose level was calculated via IPGTT (1.5 g/kg, J, n=5) or IPITT (0.75 U/kg, K, n=5). (L) Representative images of F4/80 staining (left), quantification of CLSs (middle) and adipose diameter (right) (n=5) in the epiWAT of WT or Mir802 KO mice. Scale bar: 40 μm. (M–N) The percentage of CD11b+/F4/80+ total macrophages (M, n=5) and M1 (CD86+CD206–), and M2 (CD206+CD86–) within the macrophage population (N, n=5) in the SVFs isolated from epiWAT in the HFD-control or Mir802 eWAT KD/HFD mice. (O) Serum levels of TNF-α, IL-6, IL-1β, CCL2 determined with ELISA (n=6). (P) IPGTT was performed in HFD-control mice or Mir802 eWAT KD/HFD mice(n=5). Data represent mean ± SEM. Differences between groups were determined by ANOVA (A–B, D, E–K, N–P) or two-tailed unpaired Student’s t test (C, L–M). ***p<0.001. Gene levels were normalized to Rn18s abundance.
We next examined the activity of Mir802 in obese adipose tissues in which inflammation had already been established. We performed the acute deletion of adipocyte Mir802 that did not influence whole-body weight. To address this question, we depleted Mir802 in eWAT using an approach of adeno-associated virus (AAV, Mir802 eWAT KD) to 16-week-old DIO mice that had been fed an HFD since they were 4 weeks old (Figure 3—figure supplement 2A). After 7 days, we detected 70% lower expression of Mir802 compared with the control in the epididymal fat pad; Mir802 expression was unaffected in other organs except for BAT (Figure 3—figure supplement 2B). The weight and number of CLSs were lowered with Mir802 sponge treatment (Figure 3—figure supplement 2C, Figure 3L), and the reduction in macrophage infiltration was confirmed by CD11b and F4/80 flow cytometry analysis (Figure 3M, Figure 3—figure supplement 2D). Phenotypic analysis indicated that Mir802 inhibitor also lowered the M1 (CD86+CD206-) macrophage fraction, while it increased the M2 macrophage (CD206+CD86-) fraction (Figure 3N, Figure 3—figure supplement 2E). DIO led to upregulated mRNA expression of proinflammatory cytokines (Il1b, Il6, and Tnfa) in the adipose tissue that was suppressed in the Mir802 eWAT KD mice (Figure 3O). Mir802 inhibitor treatment also ameliorated insulin resistance and glucose intolerance in DIO mice (Figure 3P, Figure 3—figure supplement 2F). These results clearly show that Mir802 inhibitor treatment suppresses preexisting adipose inflammation, which strongly suggests that Mir802 is required for the maintenance of inflammatory reactions in obese adipose tissue.
Interplay between Mir802 ectopically expressed adipocytes and macrophages
We next analyzed the cellular interplay via which inflammation develops in obese adipose tissue. Based on the findings of the in vivo experiments summarized above, we hypothesized that obese adipose tissue upregulates Mir802, and Mir802-overexpressing adipocytes in turn recruit and activate macrophages. To test this hypothesis, we first co-cultured bone-marrow-derived differentiated macrophages (BMDMs) with differentiated WAT SVF cells obtained from either lean or obese mice to assess whether adipose tissue from obese mice can influence the behavior of BMDMs (Figure 4A). EdU assays and flow cytometric analysis showed that obese differentiated WAT SVF induced the proliferation of BMDMs, whereas lean fat did so only mildly (Figure 4—figure supplement 1A, B). Transwell co-culture further showed that obese WAT differentiated SVF also promoted BMDMs migration and invasion (Figure 4B). We next explored the effects of obese mice’ WAT differentiated SVF on the characteristics of BMDMs. After co-culture BMDMs and differentiated WAT SVF of obese mice, BMDMs had elevated expression of classical activation (M1-like) marker CD86, whereas the alternative activation marker (M2-like) CD206 was decreased (Figure 4C, Figure 4—figure supplement 1C). The results of ELISA indicated that obese mice’ WAT SVF-induced BMDMs were predominantly polarized to pro-inflammatory macrophages (Figure 4D).
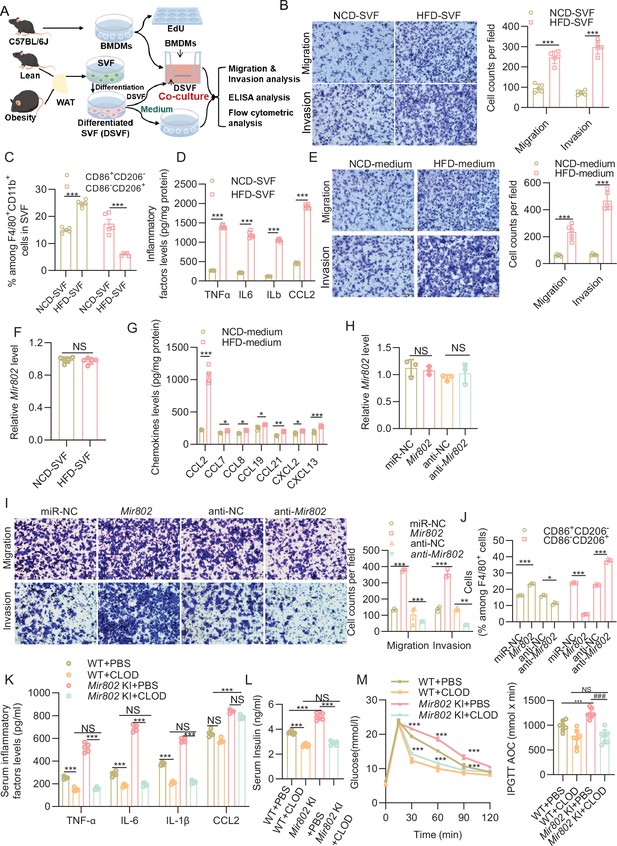
Interplay between Mir802 ectopically expressed adipocytes and macrophages.
(A) Flowchart of the co-culture experiments designed for determining WAT SVF of obese adipose tissue can affect macrophages (bone marrow derived macrophages BMDMs). (B) Obesity promoted BMDMs migration and invasion in transwell migration and invasion assay. (C) M1 (CD86+CD206–) and M2 (CD206+CD86–) within the macrophage population. (D) The levels of TNF-α, IL-6, IL-1β, and CCL2 determined with ELISA. (E) Migration and invasion ability of BMDMs treated with a medium conditioned with obese or lean SVF cells. (F) The Mir802 expression levels in the BMDMs after co-cultured WAT SVF cells. (G) Chemokine levels in the medium conditioned with obese or lean SVF cells. (H) The Mir802 expression levels in the RAW264.7 cells after co-cultured with 3T3-L1 cells transfected with Mir802 mimics or Mir802 inhibitor. (I) Mir802 induced 3T3-L1 cells recruitment more RAW 264.7 cells in transwell migration and invasion assay. (J) Mir802 mimics-transfected 3T3-L1 cells promoted RAW 264.7 cells M1-like polarization. (K–M) Clodronate-conjugated liposomes (CLOD-liposomes) was injected into WT and Mir802 KI mice. Serum levels of TNF-α, IL-6, IL-1β, CCL2 determined with ELISA n=5, (K); serum insulin levels tested with ELISA n=5, (L); glucose tolerance tested by IPGTT n=7, (M). Data represent mean ± SEM. Differences between groups were determined by ANOVA (D–E, G–M), or two-tailed unpaired Student’s t test (F). **p<0.01, ***p<0.001.
We next to investigate which factors induce the crosstalk between adipose and macrophage. We plated BMDMs in boyden chambers and treated them with a medium conditioned with obese mice’ WAT differentiated SVF or lean mice’ WAT differentiated SVF, the number of BMDMs that migrated through the pores between chamber wells with obese mice’ WAT differentiated SVF conditioned medium was significantly higher than the number of cells cultured in lean mice’ WAT differentiated SVF conditioned medium (Figure 4E). qRT-PCR results showed that Mir802 expression in the BMDMs has no change (Figure 4F), while ELISA results showed that conditioned medium of obese differentiated WAT SVF can secrete more humoral factors known to induce BMDMs migration, especially CCL2 (Figure 4G).
To further confirm the function of Mir802 in adipose tissue, the adipocyte cell line 3T3-L1 was transfected with Mir802 mimics (Mir802) or Mir802 inhibitor (anti-Mir802). We then explored the effect of Mir802 ectopically expressed 3T3-L1 cells on the macrophage cell line RAW 264.7 in co-culture (Figure 4—figure supplement 1D). The knockdown and overexpression efficiencies were approximately 80% and 240-fold, respectively (Figure 4—figure supplement 1E). First, we found that the Mir802 levels were no different in the RAW 264.7 cells (Figure 4H), Mir802-overexpressing 3T3-L1 cells had no effect on the proliferation and lipid droplet production of RAW 264.7 cells (Figure 4—figure supplement 1F, G). However, Mir802-overexpressing 3T3-L1 cells promoted the migration and invasion of RAW 264.7 cells, whereas 3T3-L1 cells knocked down by anti-Mir802 had the opposite effect (Figure 4I). Mir802 mimics-transfected 3T3-L1 cells also promoted RAW 264.7 cells M1-like polarization (Figure 4J, Figure 4—figure supplement 1I). We also found higher level of CCL2 in the medium conditioned with Mir802-overexpressed 3T3-L1 cells (Figure 4—figure supplement 2A). Moreover, we found that without co-culture, only CCL2 was increased, TNF-α, IL-6, IL-1β levels have no difference (Figure 4—figure supplement 2B). Additionally, we have performed the migration/invasion assay with no adipocyte, co-culture with adipocyte, co-culture with adipocyte transfected Mir802 mimics, co-culture with adipocyte transfected Mir802 mimics and added emapticap pegol (also known as NOX-E36, CCL2 inhibitor). The results showed that no adipocyte, RAW 264.7 cells almost have no ability to migration and invasion, co-culture with Mir802 mimics promoted the migration and invasion of RAW 264.7 cells compared to co-culture with miR-NC, while blocking CCL2 in Mir802-overexpressed 3T3-L1 cells exhibited reduced RAW 264.7 cells recruitment ability (Figure 4—figure supplement 2C).
We next investigated whether the metabolic phenotypes in adipose-selective Mir802 KI mice depend on the presence of macrophages. To this end, both adipose-specific Mir802 KI mice and WT littermates were intraperitoneally injected with clodronate-conjugated liposomes (CLOD-liposomes) to deplete macrophages (Hui et al., 2015), and PBS-liposomes as vehicle control. The clodronate liposomes treatment ameliorated systematic inflammation, as displayed by the decreased levels of serum inflammatory factors (TNF-α, IL-6, IL-1β, and CCL2), and the differences in these circulating factors except CCL2 between Mir802 KI mice and WT littermates became indistinguishable after macrophage depletion, suggesting that the macrophages are not the main source of CCL2 (Figure 4K). Furthermore, macrophage depletion also led to recover hyperinsulinemia (Figure 4L) and HOMA-IR (Figure 4—figure supplement 2D) in Mir802 KI mice after the treatment. Similarly, Macrophage depletion with CLOD-liposomes obviously alleviated the HFD-induced glucose intolerance in Mir802 KI mice, and abrogated the differences of these metabolic indicators between Mir802 KI mice and WT mice (Figure 4M). Taken together, these data support macrophages as an important mediator for adipose Mir802–induced systemic inflammation (Figure 4—figure supplement 2E).
miRNA-802 promotes adipose tissue inflammation and insulin resistance by targeting TRAF3
To better understand the role of Mir802 in regulating macrophage-mediated adipose tissue inflammation and insulin resistance, we next set out to identify the target genes of Mir802 in adipocytes. For that, we utilized RNA-sequencing of samples derived from the epiWAT of Mir802 KI mice and their WT littermates. A total of 191 differentially expressed genes were identified. The cutoff criteria for significant differentially expressed genes were log fold change >2 and adjusted p-value <0.05. We identified 29 upregulated genes and 57 downregulated genes (Figure 5A left, Supplementary file 1b). Then, we combined the multiMiR database (Ru et al., 2014) with prediction programs (TargetScan Release 7.0 and miRPathDB) to predict possible targets of Mir802. Among 18 tested potential targets, TNF-receptor-associated factor 3 (Traf3) was identified as a genuine target of Mir802, which was among the genes that were significantly downregulated in Mir802 KI versus WT epiWAT (Figure 5A right, Figure 5—figure supplement 1A). Indeed, we observed that TRAF3 was decreased in both mRNA and protein levels in obese humans and in the WAT of HFD, Lepob/ob and Leprdb/db mice (Figure 5B, Figure 5—figure supplement 1B). The targeting potential between Mir802 and Traf3 was also observed in Mir802 KI and Mir802 KO mice (Figure 5D, Figure 5—figure supplement 1D). We then demonstrated Mir802 binding to the Traf3 3’-UTR by transiently co-expressing luciferase reporter fusions of Traf3 and Mir802 mimics in 3T3-L1 cells. The results of these co-transfection experiments indicated that the relative luciferase activity in Traf3 3’-UTR-expressing cells was significantly inhibited by Mir802, whereas other Traf3 3’-UTR fusions that contained mutations (Traf3-MUT) in Mir802 binding sites were unaffected (Figure 5E). Consistent with these findings, the ectopic expression of Mir802 in 3T3-L1 cells effectively regulated the mRNA and protein levels of endogenous Traf3 (Figure 5F, Figure 5—figure supplement 1E). Moreover, we conducted anti-Ago2 RIP in 3T3-L1 cells, which transiently overexpressed Mir802. Endogenous Traf3 pulldown by Ago2 was specifically enriched in Mir802-transfected cells (Figure 5G) and vice versa (Figure 5—figure supplement 1F). Overall, these data suggest that Traf3 is a direct target of Mir802.
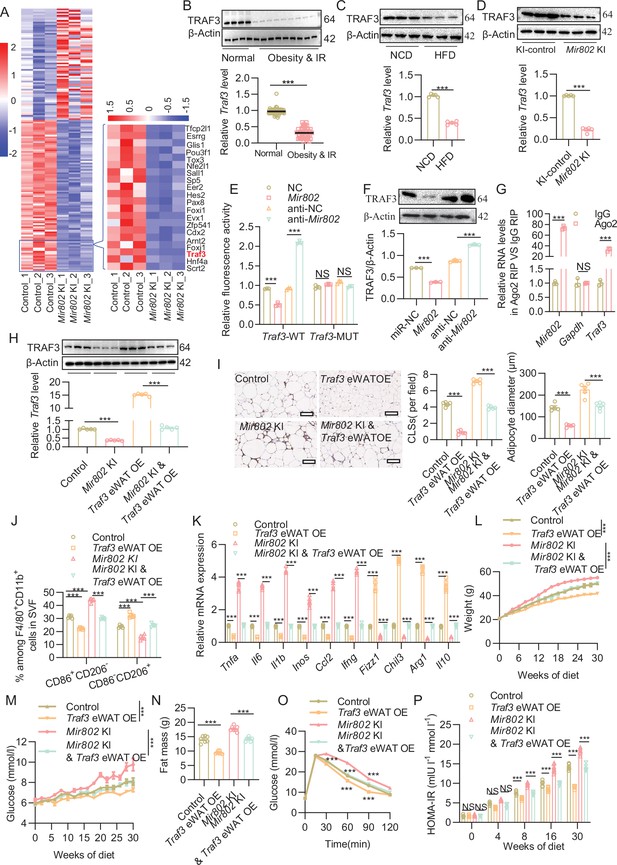
Adipose Mir802 modulates recruitment and polarization of macrophages by directly targeting Traf3.
(A) Heat map illustrating the differential expression of mRNAs in the epiWAT of Mir802 KI mice compared to their WT Mir802ki/ki littermates (n=3). (B) mRNA and protein levels of TRAF3 in human subcutaneous adipose tissues from obese and normal individuals (nnormal = 4 and nobesity&IR=9). (C, D) mRNA and protein levels of TRAF3 in the epiWAT of HFD mice (C, n=3–5) or Mir802 KI mice (D, n=3–5). (E) Relative luciferase activity in 3T3-L1 cells co-transfected with Mir802 mimics and a luciferase reporter containing either Traf3-WT or Traf3-MUT. Data are presented as the relative ratio of Renilla luciferase activity to firefly luciferase activity. (F) Protein levels of TRAF3 in 3T3-L1 cells transfected with Mir802 mimics or Mir802 inhibitor. (G) Anti-Ago2 RIP was performed in 3T3-L1 cells transiently overexpressing Mir802, followed by qRT-PCR to detect Traf3 associated with Ago2 (nonspecific IgG served as a negative control). (H) mRNA and protein levels of TRAF3 in the epiWAT of control, Mir802 KI, Traf3 eWAT OE, and Mir802 KI and Traf3 eWAT OE mice (n=3–5). (I) Representative images of F4/80 staining (left), quantification of CLSs (middle) and adipose diameter (right, n=5). (J) M1 (CD86+CD206–) and M2 (CD206+CD86–) within the macrophage population (n=5). (K) qRT-PCR analysis of the mRNA levels of M1 and M2 markers in the epiWAT of HFD-fed control, Traf3 eWAT OE Mir802 KI, and Mir802 KI & Traf3 eWAT OE(n=5). (L, M) Dynamic changes in body weight (L), glucose level (M), fat mass (N), glucose tolerance (O), and HOMA-IR (P) of control, Mir802 KI, Traf3 eWAT OE and Mir802 KI and Traf3 eWAT OE mice during 30 weeks of HFD feeding (n=7). Data represent mean ± SEM. Differences between groups were determined by ANOVA (E–P). ***p<0.001. Mir802 abundance was normalized to U6 level, and other genes levels were normalized to Rn18s abundance.
-
Figure 5—source data 1
Related to Figure 5B.
The original files of the full raw unedited blots of TRAF3 and β-Actin in human subcutaneous adipose tissues from obese and normal individuals (nnormal = 4 and nobesity&IR=9).
- https://cdn.elifesciences.org/articles/99162/elife-99162-fig5-data1-v2.zip
-
Figure 5—source data 2
Related to Figure 5C.
The original files of the full raw unedited blots of TRAF3 and β-Actin in the epiWAT of HFD mice (n=3).
- https://cdn.elifesciences.org/articles/99162/elife-99162-fig5-data2-v2.zip
-
Figure 5—source data 3
Related to Figure 5D.
The original files of the full raw unedited blots of TRAF3 and β-Actin in the epiWAT of Mir802 KI mice (n=3).
- https://cdn.elifesciences.org/articles/99162/elife-99162-fig5-data3-v2.zip
-
Figure 5—source data 4
Related to Figure 5F.
The original files of the full raw unedited blots of TRAF3 and β-Actin in 3T3-L1 cells transfected with Mir802 mimics or Mir802 inhibitor.
- https://cdn.elifesciences.org/articles/99162/elife-99162-fig5-data4-v2.zip
-
Figure 5—source data 5
Related to Figure 5H.
The original files of the full raw unedited blots of TRAF3 and β-Actin in the epiWAT of control, Mir802 KI, Traf3 eWAT OE, and Mir802 KI and Traf3 eWAT OE mice (n=3).
- https://cdn.elifesciences.org/articles/99162/elife-99162-fig5-data5-v2.zip
To address whether the increase in inflammation and insulin resistance in Mir802 KI mice was attributable to decreased Traf3, 8-week-old male Mir802 KI mice were given AAV-Adipoq-Traf3 (Mir802 KI & Traf3 eWAT OE) through epididymal fat pad. At 1 week after injection of adeno-associated virus (AAV) expressing Traf3, Traf3 expression in the epiWAT of Mir802-KI mice was increased to a level similar to that in WT mice (Figure 5H). Notably, upregulation of Traf3 led to significant decreases in the counts of total macrophages (Figure 5J, Figure 5—figure supplement 1G) and M1 macrophages (Figure 5J, Figure 5—figure supplement 1H) in the epiWAT of HFD-fed Mir802 KI mice compared with those treated with AAV8-vector. Coherently, the increased expression of M1 macrophage-associated proinflammatory factors (Tnfa, Il6, Inos, Il1b, and Ifng) in the epiWAT of HFD-fed Mir802 KI mice was reversed by the AAV-mediated upregulation of Traf3 (Figure 5K). In addition, Traf3 eWAT OE reversed the weight gain (Figure 5L), hyperglycemia (Figure 5M), and adiposity (Figure 5N) induced by overexpression of Mir802. MRI analysis further confirmed that Traf3 can reverse the increase in visceral fat caused by Mir802 (Figure 5—figure supplement 1I). Consistent with these findings, upregulation of Traf3 led to restoration of glucose intolerance (Figure 5O) and insulin resistance (Figure 5—figure supplement 1J) after 16 weeks of Traf3 eWAT treatment in HFD-fed Mir802 KI mice, coupled with a decrease in fasting insulin levels (Figure 5—figure supplement 1K) and ameliorative HOMA-IR (Figure 5P). Taken together, these findings support the notion that elevated Mir802 induces macrophage recruitment and polarization at least partly via downregulation of Traf3, thereby leading to adipose tissue inflammation and insulin resistance.
Mir802 activates noncanonical and canonical NF-κB pathways leading to macrophage recruitment
To further unravel the mechanism by which inhibition of TRAF3 expression induces adipose tissue inflammation, we looked for TRAF3 downstream cascades. Several studies have suggested that TRAF3 negatively regulates the noncanonical NF-κB pathway (Liao et al., 2004; He et al., 2004; He et al., 2007), which is consistent with the KEGG analysis based on our RNA-seq results (Figure 6—figure supplement 1A). This prompted us to measure NF-κB inducing kinase (NIK) protein levels and to explore the processing of p100 to p52. To test whether Mir802 is required for the suppression of NIK protein levels, western blot analysis of NIK was performed on Mir802-overexpressed 3T3-L1 cells and Mir802 ectopically expressed adipose tissue. As shown in Figure 6A and B, profound accumulation of NIK was observed in all cells with overexpression of Mir802, which correlated well with decreased TRAF3. Mir802 selectively ablated adipose tissues showed the opposite result (Figure 6—figure supplement 1B). Processing of the p100 precursor to p52, the hallmark of noncanonical NF-κB activation, was also assessed by immunoblotting. Although 3T3-L1 cells exhibited the normal kinetics of p100 processing with substantial p52 accumulation by 48 hr after treatment with the empty vector, Mir802-overexpressing 3T3-L1 cells and Mir802 selectively overexpressed adipose tissues showed constitutive and total processing of the p100 precursor protein (Figure 6C and D). On the contrary, there was less accumulation of p52 in Mir802 selectively deleted adipose tissue (Figure 6—figure supplement 1C). As expected, IKK-α phosphorylation levels were also enhanced in 3T3-L1 cells and in the epiWAT of Mir802 KI mice (Figure 6E, Figure 6—figure supplement 1D). To confirm that Mir802 activates the noncanonical NF-κB pathway through TRAF3, Traf3 plasmid was transfected into Mir802-overexpressing 3T3-L1 cells, then NIK protein levels and processing of p100 to p52 were again assessed by immunoblotting. As shown in Figure 6G, Traf3 restored the levels of NIK and the processing of p100 to p52 in Mir802-overexpressing 3T3-L1 cells. Moreover, these results were confirmed in Mir802 KI mice (Figure 6H), indicating that Mir802 regulated the noncanonical NF-κB pathway via TRAF3.
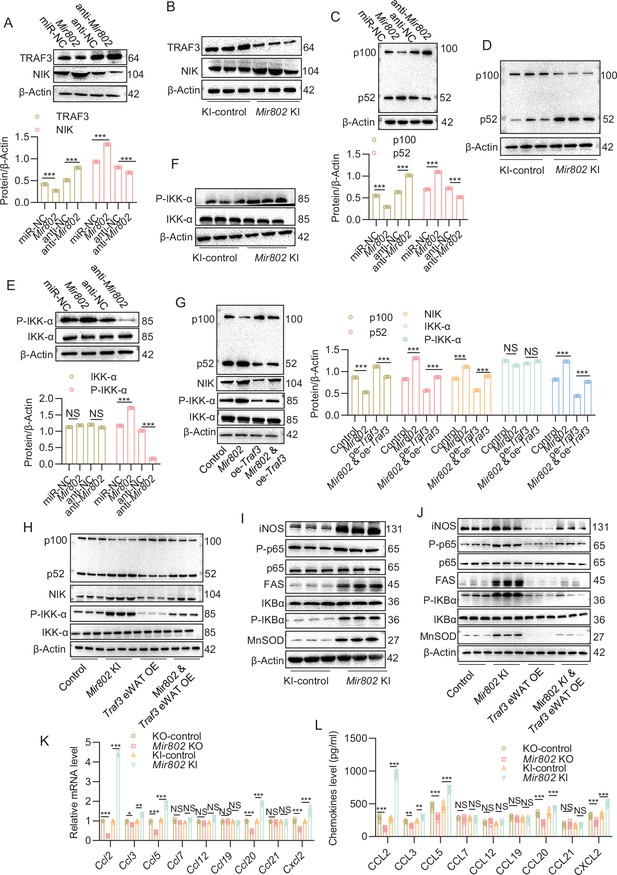
Mir802 activates noncanonical and canonical NF-κB pathways by recruiting macrophages.
(A, B) NIK protein levels in 3T3-L1 cells transfected with Mir802 mimics or Mir802 inhibitor (A) in the epiWAT of Mir802 KI mice (B, n=3). (C, D) P100/52 protein levels in 3T3-L1 cells transfected with Mir802 mimics or Mir802 inhibitor (C), and in the epiWAT of Mir802 KI mice (D, n=3). (E, F) Protein levels of IKK-α and P-IKK-α in 3T3-L1 cells transfected with Mir802 mimics or Mir802 inhibitor (E) in the epiWAT of Mir802 KI mice (F, n=3). (G, H) Overexpression of Traf3 reverses the protein levels of NIK, P-IKK-α, and P100/52 in 3T3-L1 cells (G) and in the epiWAT of Mir802 KI mice (H, n=3). (I, J) Protein levels of some major canonical NF-κB signaling targets in the epiWAT of Mir802 KI mice (I, n=3) and Traf3 eWAT OE rescued mice (J, n=3). (K, L) qRT-PCR (K) and ELISA (L) were performed to detect major chemokine levels. Data represent mean ± SEM. Differences between groups were determined by ANOVA (K–L). ***p<0.001. Genes levels were normalized to Rn18s abundance.
-
Figure 6—source data 1
Related to Figure 6A.
The original files of the full raw unedited blots of TRAF3, NIK, and β-Actin in 3T3-L1 cells transfected with Mir802 mimics or Mir802 inhibitor.
- https://cdn.elifesciences.org/articles/99162/elife-99162-fig6-data1-v2.zip
-
Figure 6—source data 2
Related to Figure 6B.
The original files of the full raw unedited blots of TRAF3, NIK, and β-Actin in the epiWAT of Mir802 KI mice (n=3).
- https://cdn.elifesciences.org/articles/99162/elife-99162-fig6-data2-v2.zip
-
Figure 6—source data 3
Related to Figure 6C.
The original files of the full raw unedited blots of p100/p52 and β-Actin in 3T3-L1 cells transfected with Mir802 mimics or Mir802 inhibitor.
- https://cdn.elifesciences.org/articles/99162/elife-99162-fig6-data3-v2.zip
-
Figure 6—source data 4
Related to Figure 6D.
The original files of the full raw unedited blots of p100/p52 and β-Actin in the epiWAT of Mir802 KI mice (n=3).
- https://cdn.elifesciences.org/articles/99162/elife-99162-fig6-data4-v2.zip
-
Figure 6—source data 5
Related to Figure 6E.
The original files of the full raw unedited blots of P-IKK-α, IKK-α, and β-Actin in 3T3-L1 cells transfected with Mir802 mimics or Mir802 inhibitor.
- https://cdn.elifesciences.org/articles/99162/elife-99162-fig6-data5-v2.zip
-
Figure 6—source data 6
Related to Figure 6F.
The original files of the full raw unedited blots of P-IKK-α, IKK-α, and β-Actin in the epiWAT of Mir802 KI mice (n=3).
- https://cdn.elifesciences.org/articles/99162/elife-99162-fig6-data6-v2.zip
-
Figure 6—source data 7
Related to Figure 6G.
The original files of the full raw unedited blots of p100/p52, P-IKK-α, IKK-α, NIK, and β-Actin in the 3T3-L1 cells.
- https://cdn.elifesciences.org/articles/99162/elife-99162-fig6-data7-v2.zip
-
Figure 6—source data 8
Related to Figure 6H.
The original files of the full raw unedited blots of p100/p52, P-IKK-α, IKK-α, NIK, and β-Actin in the epiWAT of Mir802 KI and Traf3 eWAT OE mice (n=3).
- https://cdn.elifesciences.org/articles/99162/elife-99162-fig6-data8-v2.zip
-
Figure 6—source data 9
Related to Figure 6I.
The original files of the full raw unedited blots of some major canonical NF-κB signaling targets in the epiWAT of Mir802 KI mice(n=3).
- https://cdn.elifesciences.org/articles/99162/elife-99162-fig6-data9-v2.zip
-
Figure 6—source data 10
Related to Figure 6J.
The original files of the full raw unedited blots of some major canonical NF-κB signaling targets in the epiWAT of Mir802 KI mice and Traf3 eWAT OE rescued mice (n=3).
- https://cdn.elifesciences.org/articles/99162/elife-99162-fig6-data10-v2.zip
Previous studies have suggested that TRAF3 also suppresses activation of the canonical NF-κB pathway (Zarnegar et al., 2008; Bista et al., 2010). To verify whether Mir802 can regulate the canonical NF-κB pathway through TRAF3, nuclear extract was harvested from the adipose tissue of 16-week-old WT and mice with adipose-selective forced expression of Mir802. NF-κB activation status was then assessed by measuring p65, IκBα, and some major targets associated with the pathway. As shown in Figure 6I and p65 and IκBα were phosphorylated, and some major targets of canonical NF-κB signaling, such as MnSOD, FAS, and iNOS, were activated in Mir802 KI mice. As expected, the activation of canonical NF-κB signaling in Mir802 KI mice was partially reversed by overexpression of Traf3 (Figure 6J). To assess the potential impact of heightened NF-κB activity, we harvested mRNA from the adipose tissue of WT and Mir802 KI mice and analyzed the expression levels of multiple noncanonical and canonical NF-κB pathway target genes (Akhter et al., 2021; Cildir et al., 2016) using qRT-PCR. Here, we observed that the expression levels of CCL2, CCL3, CCL5, CCL20, and CXCL2 were elevated in the adipose tissue of Mir802 KI mice (Figure 6K), which is consistent with ELISA results using the serum of Mir802 KI mice (Figure 6L). Taken together, these data indicate that Mir802 activates the noncanonical and canonical NF-κB pathways via TRAF3, leading to macrophage recruitment.
Mir802 promotes lipid synthesis and M1 macrophage polarization in adipose tissue through activating SREBP1
The mechanism of how Mir802 increasing M1 polarization by inducing NF-κB pathways remains unclear. To better understand the role of Mir802 in regulating macrophage polarization, we performed transcriptome sequencing using epiWAT derived from Mir802 KI mice. In the adipose tissues of Mir802 KI mice, the expression of 191 mRNAs was significantly altered compared to that of mRNAs in WT mice, of which the expression of 75 mRNAs increased (Figure 7A, Supplementary file 1b). We found that seven mRNAs were upregulated more than tenfold (Figure 7A, right), and these mRNAs were annotated using UCSC and Ensemble. Among these upregulated genes, we focused on the lipogenic gene sterol regulatory element-binding protein 1 a (SREBP1a), which is involved in fatty acid synthesis and lipid droplet formation (Shimano and Sato, 2017). We verified that the mRNA levels of Srebp1a were increased in both the epiWAT of Mir802 KI mice and in 3T3-L1 cells transfected with Mir802 mimics in qRT-PCR analysis (Figure 7—figure supplement 1A and B). We also found that the mature form of the SREBP-1 protein (m-SREBP1) was significantly higher in the epiWAT of Mir802 KI and 3T3-L1 cells transfected with Mir802 mimics (Figure 7B and C). As expected, overexpression of Traf3 reduced the upregulation level of mature SREBP1 induced by Mir802 (Figure 7D). However, target gene prediction algorithms as well as luciferase reporter and Ago2-RIP assays confirmed that Srebp1a is not the direct target gene for Mir802 (data not shown). This prompted us to verify whether Srebp1a is a downstream gene in the NF-κB pathway.
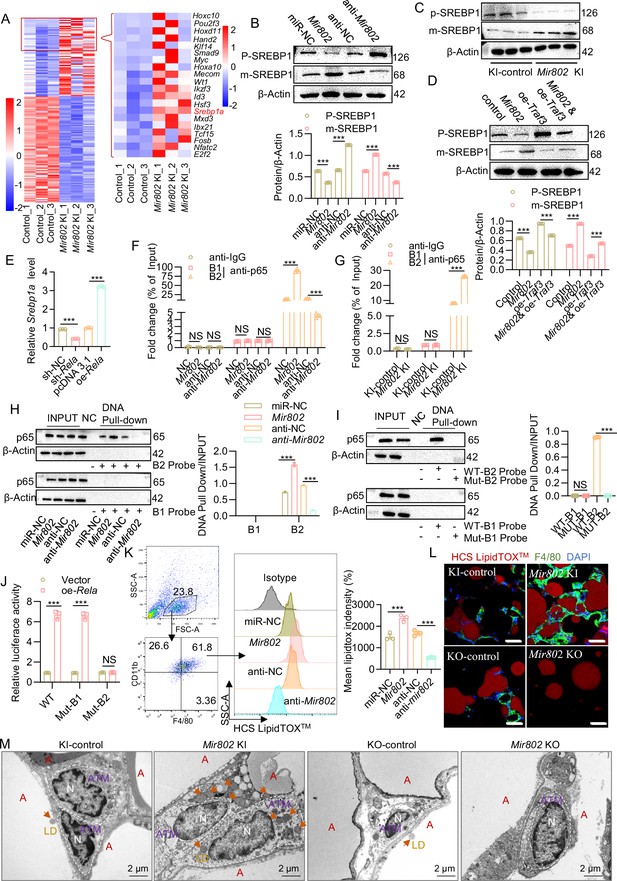
Mir802 promotes lipogenesis and induces M1 macrophage polarization in adipose tissue through activating SREBP1.
(A) Heat map illustrating the top 20 upregulated mRNAs in the epiWAT of Mir802 KI mice compared to their WT Mir802fl/fl littermates (n=3). (B–C) Protein levels of the mature form of SREBP-1 protein (m-SREBP1) and the precursor form of SREBP-1 (P-SREBP1) in mature 3T3-L1 cells transfected with Mir802 mimics or Mir802 inhibitor (B), in the epiWAT of Mir802 KI mice (C, n=3). (D) The protein levels of m-SREBP1 and P-SREBP1 were reversed by Traf3. (E) Srebp1a mRNA levels in 3T3-L1 cells transfected with Rela-overexpressing plasmid or Rela shRNA plasmid. (F, G) ChIP-qPCR assays were conducted to verify that Rela binds to the Srebp1 promoter in 3T3-L1 cells transfected with Mir802 mimics or Mir802 inhibitor (F) and in the epiWAT of Mir802 KI mice (G, n=3). (H) DNA pull-down assay using a biotinylated DNA probe corresponding to the −360 to −400 or −1198 to −1237 region of the Srebp1 promoter in 3T3-L1 cells transfected with Mir802 mimics or Mir802 inhibitor. (I) DNA pull-down assay using a biotinylated DNA probe corresponding to the −1198 to −1237 region of the wild-type (WT) or a mutant sequence of the Srebp1 promoter in 3T3-L1 cells stimulated with Rela plasmid for 48 hr. (J) Luciferase reporter assays in 3T3-L1 cells transfected with the indicated plasmids for 48 hr. Dual-luciferase activity was determined. (K) Mir802 mimics or Mir802 inhibitor was transfected into 3T3-L1 cells, then direct contact co-culture with mature 3T3-L1 and RAW264.7 cells, flow cytometry analysis of cellular neutral lipid content using the LipidTOX in cells. (L) Representative images of the immunofluorescence of lipid droplets (HCS LipidTOX, Red) and F4/80 (Green, n=3). Scale bar: 20 μm. (M) Transmission electron microscopy (TEM) was performed to detect the contact between lipid droplets and macrophages (n=3). Data represent mean ± SEM. Differences between groups were determined by ANOVA (E–G and J). ***p<0.001. Genes levels were normalized to Rn18s abundance.
-
Figure 7—source data 1
Related to Figure 7B.
The original files of the full raw unedited blots of m-SREBP1, P-SREBP1, and β-Actin in mature 3T3-L1 cells transfected with Mir802 mimics or Mir802 inhibitor.
- https://cdn.elifesciences.org/articles/99162/elife-99162-fig7-data1-v2.zip
-
Figure 7—source data 2
Related to Figure 7C.
The original files of the full raw unedited blots of m-SREBP1, P-SREBP1, and β-Actin in in the epiWAT of Mir802 KI mice (n=3).
- https://cdn.elifesciences.org/articles/99162/elife-99162-fig7-data2-v2.zip
-
Figure 7—source data 3
Related to Figure 7D.
The original files of the full raw unedited blots of m-SREBP1, P-SREBP1, and β-Actin in the 3T3-L1 cells.
- https://cdn.elifesciences.org/articles/99162/elife-99162-fig7-data3-v2.zip
-
Figure 7—source data 4
Related to Figure 7H.
The original files of the full raw unedited blots of p65 and β-Actin in 3T3-L1 cells transfected with Mir802 mimics or Mir802 inhibitor.
- https://cdn.elifesciences.org/articles/99162/elife-99162-fig7-data4-v2.zip
-
Figure 7—source data 5
Related to Figure 7I.
The original files of the full raw unedited blots of p65 and β-Actin in 3T3-L1 cells stimulated with Rela plasmid for 48 hr.
- https://cdn.elifesciences.org/articles/99162/elife-99162-fig7-data5-v2.zip
For this purpose, we first predicted the nuclear factor-κB (NF-κB) family (Rela, Relb, Rel, Nfκb1, and Nfκb2) sites in the promoter of Srebp1a using JASPAR and the Promo database. Two Rela potential binding sites (B1 and B2) were found in the promoter of Srebp1a (Figure 7—figure supplement 1C). We next overexpressed Rela in 3T3-L1 cells; qRT-PCR results showed that Rela could increase Srebp1a expression in 3T3-L1 cells (Figure 7E). To determine whether Srebp1a is a direct Rela target gene, ChIP-qPCR assays were used. The results showed that occupancy of Rela binding site 2 (B2) on the Srebp1a promoter was significantly increased in Mir802-overexpressing 3T3-L1 cells and Mir802 selectively overexpressed adipose tissues (Figure 7F and G, Figure 7—figure supplement 1D). We then conducted DNA pull-down assays to examine the binding of Rela to the Srebp1a promoter in vitro. We constructed two DNA probes containing −360 to −400 or −1198 to −1237 regions that contained the predicted binding site 1 (B1) and predicted binding site 2 (B2), respectively, to detect binding to Rela in nuclear extracts. Similar findings were obtained in that the B2 DNA probe, but not the B1 DNA probe, bound to Rela in the 3T3-L1 cell line overexpressing Mir802 (Figure 7H). However, with mutant B2 (agggaatgct, Mut2), DNA pull-down results showed that Rela could not bind to Mut2 (Figure 7I). Moreover, we constructed a luciferase reporter plasmid containing the Srebp1a promoter region from −1295 to +1 WT and two mutant reporter plasmids mutated in −375 to −385 (Mut1) or in −1211 to −1221 (Mut2). Overexpression of Rela significantly enhanced WT and Mut1, but not Mut2-driven activity luciferase in 3T3-L1 cells (Figure 7J). Taken together, these results indicated that Mir802 indirectly stimulates Srebp1a expression via the canonical NF-κB signaling pathway (Figure 7—figure supplement 1F).
As previously described, Srebp1a is a well-established regulator of lipid synthesis (Shimano and Sato, 2017). Accordingly, Mir802 overexpression significantly increased the number and fluorescence intensity of lipid droplets, which correlated well with increased SREBP1. Conversely, knockdown of Mir802 strongly reduced lipid droplet formation in 3T3-L1 cells and Mir802-KO mouse adipose tissue (Figure 7—figure supplement 1G and H). Lipid droplets have been shown to play a crucial role in M1 macrophage polarization (Lumeng et al., 2007a; Prieur et al., 2011). Consistent with this, we found overexpression-Mir802 3T3-L1 cells promoted RAW264.7 cells to engulf more lipid droplets through direct contact co-culture with mature 3T3-L1 and RAW264.7 cells, while the content of lipid droplets is decreased in the knockdown-Mir802 3T3-L1 cells (Figure 7K). Moreover, we also observed that adipose tissue macrophages (ATMs) of the Mir802 KI mice could engulf more lipid droplets (Figure 7L and M). The elevated expression of the classical activation marker further indicated that lipid droplets induced the ATMs in Mir802 KI mice to the pro-inflammatory phenotype (Figure 7—figure supplement 1I). Altogether, these data show that Mir802 indirectly regulates lipid droplet formation through SREBP1 and ultimately promotes macrophage M1 polarization.
Discussion
Macrophage infiltration of adipose tissue has been described in both mice and humans during obesity. However, how lipid-loaded hypertrophic adipocytes send signals to trigger infiltration and alter the polarization of macrophages in obesity remains poorly understood. In this study, we found that Mir802 endows adipose tissue with the ability to interact with macrophages and regulate the inflammatory cascade. Mechanistically, Mir802 promotes adipose cells to secrete CCL2 recruiting macrophages, and Mir802 increases lipid synthesis and promote macrophage to engulf lipids driving the polarization program toward proinflammatory M1 phenotype by targeting the cytoplasmic adaptor protein TRAF3 (Figure 8). Our findings indicate that Mir802 has essential roles in the initiation and maintenance of adipose tissue inflammation and systemic insulin resistance.
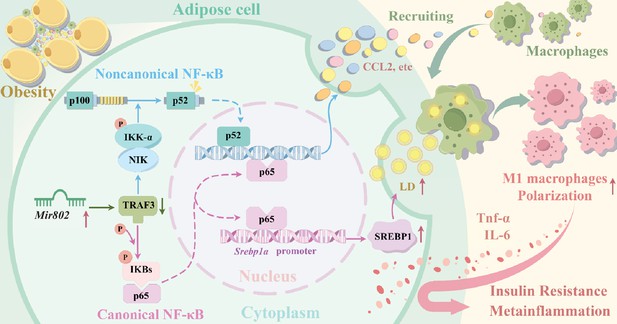
Schematic illustration for the mechanism of Mir802 exacerbates adipose tissue inflammation and leads to metabolic dysfunction during obesity.
We found that Mir802 endows adipose tissue with the ability to interact with macrophages and regulate the inflammatory cascade. During obesity, Mir802 promotes adipose tissue secretion more chemokines to recruit macrophages by targeting Traf3 activating canonical and noncanonical NF-κB signaling pathways; and Mir802 increases lipogenesis through promoting Srebp1 transcription, leading macrophages toward proinflammatory M1 phenotype by engulfing lipid droplets. Schematic illustration was drawn by figdraw.
Adipose tissue inflammation is a hallmark of obesity and a causal factor of metabolic disorders such as insulin resistance. Mice fed an HFD frequently develop chronic low-grade inflammation within adipose tissues, characterized by increased infiltration of macrophages and the production of pro-inflammatory cytokines. Here, we showed that the increasing trend of Mir802 in adipocytes is an early event during the development of adipose tissue obesity induced by an HFD. Mir802 expression in visceral fat was progressively increased with the development of dietary obesity, whereas adipose-selective ablation of Mir802 protected mice from exacerbation of meta-inflammation and insulin resistance caused by dietary stress. The high level of Mir802 expression in visceral fat may partly explain why this adipose depot is more prone to inflammation and is closely related to insulin resistance. Mir802 is required for adipose tissue inflammation and has major roles in macrophage recruitment and polarization. Thus, Mir802 is crucially involved in initiating inflammatory cascades in obese adipose tissue. Moreover, the finding that Mir802 inhibitor treatment ameliorated pre-established adipose inflammation in DIO mice indicates that Mir802 is also essential for maintenance of the inflammatory response. Although previous studies have found that Mir802 was up-regulated in the adipose tissue during obesity (Zhang et al., 2020; Kornfeld et al., 2013; Ge et al., 2022; Goga et al., 2021), the function of Mir802 was focused on cancers (Gao et al., 2021), liver (Seok et al., 2021; Ni et al., 2021), small intestine (Goga et al., 2021), and pancreas (Ge et al., 2022). And Yao et al., 2020 have determined that up-regulation of Mir802 exacerbates inflammatory process of inflammatory bowel disease via targeting Socs5, which indicates that Mir802 play an important role in regulating the inflammatory process. Whether Mir802 can regulate adipose function is still confused, our study is the first to address that Mir802 intensifies adipose tissue inflammation by affecting crosstalk between adipose and macrophage. The findings that systemic insulin resistance is ameliorated by Mir802 depletion and is aggravated by adoptive transfer of Mir802 mimics strongly suggest that Mir802-dependent adipose inflammation has an impact on systemic metabolism.
Like most other miRNAs, Mir802 regulates the expression of multiple genes in different tissues. In the liver, Mir802 is induced by obesity and impaired glucose tolerance, and it attenuates insulin sensitivity by downregulation of Hnf1b (Kornfeld et al., 2013). Genetic ablation of Mir802 in the small intestine of mice leads to decreased glucose uptake, impaired enterocyte differentiation, increased Paneth cell function, and intestinal epithelial proliferation through derepression of Tmed9 (Goga et al., 2021). We recently discovered that in pancreatic islet cells, elevated Mir802 causes impaired insulin transcription and secretion by targeting Neurod1 and Fzd5 (Zhang et al., 2020). In this study, we found that Mir802 promotes adipose tissue inflammation and insulin resistance by targeting TRAF3 in adipocytes. As a member of the TNF receptor (TNFR) superfamily, TRAF3 plays vital roles in inflammatory responses via activation of both the canonical and noncanonical NF-κB signaling pathways He et al., 2007; Zarnegar et al., 2008 following engagement of a variety of TNFR superfamily members such as Baff receptor, lymphotoxin β receptor, and CD40 (Häcker et al., 2011). Here, we found that Mir802 can regulate the NF-κB pathway by directly targeting TRAF3 rather than by activating the classic receptor, which enriches the understanding of the NF-κB pathway.
Macrophage accumulation was significantly higher in adipose tissue from HFD-fed Mir802 KI mice than in WT mice, suggesting that overexpression of Mir802 enhances the infiltration ability of macrophages. Correspondingly, we observed that Mir802-overexpressing adipocytes released more chemokines by activating NF-κB pathway, such as CCL2, CCL5, CCL20, and CXCL2. Adipose tissue inflammation is well documented as an important contributor to systemic insulin resistance (Lumeng et al., 2007b). This was further validated by our observation of enhanced adipose tissue inflammatory responses in Mir802 KI mice. Moreover, HFD-fed Mir802 KI mice exhibited adipose tissue macrophage infiltration, proinflammatory cytokine expression, and NF-κB pathway activation. Genes that are crucial for meta-inflammation and insulin resistance were directly affected by the enhancement of Mir802 in adipose tissue. Thus, increased adipose tissue inflammation resulting from Mir802 overexpression contributed, in large part, to systemic insulin resistance in Mir802 KI mice.
The chronic inflammation microenvironment is one of the main features of obesity. A recent study found that adipocytes can release lipid-filled vesicles that become a source of lipids for local macrophages (Flaherty et al., 2019). Phagocytosis or excessive accumulation of lipid droplets can induce macrophage M1 polarization (Flaherty et al., 2019; Batista-Gonzalez et al., 2019). In our study, we observed the same phenomenon, that is, in Mir802 KI mice, macrophages accumulated more lipid droplets and exhibited an inflammatory phenotype. SREBP1 has been found to promote the acute inflammatory response and lipogenesis (Im et al., 2011; Fei et al., 2023). Here, we found that Mir802 increased SREBP1 expression inducing lipogenesis by activating canonical NF-κB signaling pathways, then macrophage engulf lipid droplet promoting macrophage M1 polarization. This has enriched our understanding of the functionality of SREBP1 to some extent. However, Mir802 only indirectly regulates SREBP1, but it still has a considerable impact on macrophages, indicating the importance of miRNA positive or indirect regulation. Though Jae-Ho Lee (Lee et al., 2018) determined that Srebp1a provides lipids essential for maintaining association between the actin cytoskeletal network and plasma membranes, thus enhancing phagocytosis, whether Mir802 promotes macrophage to engulf lipid droplets via regulation the actin cytoskeletal network and plasma membranes still need to further research.
Taken together, our results support the idea that obese adipose tissue activates Mir802, which, in turn, initiates and propagates inflammatory cascades, including the recruitment of macrophages into obese adipose tissues and their subsequent induction of the inflammatory phenotype. Thus, Mir802 appears to have a primary role in obese adipose tissue inflammation. In our study, we have found that a high-fat diet (nutrition factor) and genetic factors can induce an increase in adipose Mir802. Some environmental factors including circadian rhythms (Chaix et al., 2019) and exposure to Di (2-ethylhexyl) phthalate (DEHP; Zhang et al., 2023) are also important for obesity development. The current research lacks evidence on the impact of environmental factors on Mir802 expression in adipose tissue. So future studies are needed to clarify which environmental cues such as within obese adipose tissue initiate Mir802 elevation. The present observations indicate that Mir802 inhibitors might offer a novel approach to prevent diseases associated with insulin resistance.
Materials and methods
Animal studies
Request a detailed protocolAll mice used were of mixed strain backgrounds with approximately equal contributions from C57BL/6 J, with the exceptions of Leprdb/db mice (C57BLKS/J). Mir802 conditional knockout (Mir802fl/fl) and knock-in (Mir802ki/ki) mice were generated previously via CRISPR/Cas9 system from Model Animal Research Center of Nanjing University (Nanjing, China). The detailed information was initially described in Zhang et al., 2020. Adipoq-Cre BAC transgenic mice on a C57BL/6 J background were obtained from Jackson Laboratories (#028020). Adipoq-Cre intercrossed with Mir802fl/fl or Mir802ki/ki mice to generate Mir802fl/+:Adipoq-Cre or Mir802ki/+:Adipoq-Cre mice. Mir802fl/+:Adipoq-Cre or Mir802ki/+:Adipoq-Cree mice were then bred with Mir802fl/fl or Mir802ki/ki to generate Mir802fl/fl:Adipoq-Cre/ Mir802ki/ki:Adipoq-Cre (Mir802 KO/Mir802 KI) along with Mir802fl/fl/ Mir802ki/ki and Adipoq-Cre mice. Genotyping was performed as described (Zhang et al., 2020) and the PCR primers were listed in Supplementary file 1c. Studies were performed on 8-week-old male and female mice initially housed under standard conditions with full access to standard mouse chow and water. After this time, mice were switched to a 60% high-fat diet (HFD, Research Diets, Cat# D12492) or normal chow diet (NCD, Research Diets, Cat#D12450J) consisting of a 10% fat diet for 30 weeks. All mice had free access to food and water ad libitum. Animals were housed in a temperature-controlled environment with a12 h dark–light cycle. At the end of the 30-week period, mice were euthanized via overdose of isoflurane anesthesia, and tissues were immediately weighed, dissected, and frozen in liquid nitrogen. Tissue samples were stored at –80 °C until use. Care of all animals was within institutional animal-care committee guidelines, and all procedures were approved by the animal ethics committee of China Pharmaceutical University (Permit Number: 2162326) and were in accordance with the international laws and policies (EEC Council Directive 86/609,1987).
For administration of AAV8-Adipoq-Mir802 sponge vector, AAV8-Adipoq-Traf3 vector to epididymal adipose tissue, mice were anesthetized with pentobarbital sodium (60 mg/kg) intraperitoneally and the laparotomy was performed. Each epididymal fat pad was given eight injections of 5 µl (1×1010 viral genome copies) of AAV solution.
Human adipose samples of lean and overweight individuals
Request a detailed protocolAdipose and clinicopathological data were collected from Sir Run Run Hospital, Nanjing Medical University (Nanjing, China). All patients enrolled in this study were obese (BMI >25 kg/m2). The negative controls were normal-weight individuals (20≤BMI ≤ 25 kg/m2). All human subjects provided informed consent. All human studies were conducted according to the principles of the Declaration of Helsinki and were approved by the Ethics Committees of the Department Sir Run Run Hospital (Nanjing, China, 2023-SR-046). The clinical features of patients are listed in Supplementary file 1a.
Adipose sample preparation
Request a detailed protocolSVF and mature adipocytes were obtained as follows: adipose tissue samples were digested with collagenase type 1 in Krebs-RingerHenseleit (KRH) buffer for 30 min at 37 °C. Cell suspensions containing mature adipocytes and SVF were then filtered with nylon mesh and washed three times with KRH buffer. Mature adipose was floated to the surface and the remaining solution containing the SVF was centrifuged at 1500 rpm for 5 min. The pellet was washed with pre-adipocyte growth medium (DMEM-F12 supplemented with 10% calf serum and 1% penicillin-streptomycin), followed by a second centrifugation. SVF cells were then cryopreserved using a freezing medium (DMEM-F12 supplemented with 60% FBS and 10% DMSO). The medium was added to the pellet and frozen with a temperature gradient (−1 °C/min) and stored in liquid nitrogen until analysis. Following collection, whole adipose tissue samples were quickly frozen in liquid nitrogen and stored until analysis.
3T3-L1 cell culture and differentiation
Request a detailed protocol3T3-L1 cells (1×105 well−1 in six-well plate) counted by blood counting chamber were cultured in DMEM (Gibco) containing 10% calf serum with high glucose at 37 °C, 5% CO2 and full saturation humidity until they reached 80–90% confluence, at which point the media was changed to the first differentiation medium containing high glucose DMEM, 10% FBS, 0.5 mM 3-isobutyl-1-methylxanthine (IBMX), 1 μM dexamethasone and 10 μg/ml insulin for 48 hr, then the media was changed to the terminal differentiation cocktail containing high glucose DMEM, 10% FBS, and 10 μg/ml insulin for 48 hr obtained mature 3T3-L1 cells. 3T3-L1 cell line were purchased from the Chinese Academy of Sciences (Shanghai, China). Authentication of all cells was done by short tandem repeat (STR) profiling, confirming the absence of mycoplasma contamination.
Insulin-resistant cell model
Request a detailed protocolThe insulin-resistant (IR) cell models were established in mature 3T3-L1 and mature WAT SVF cells by 0.5 mM palmitate acid (Cayman Chemical Company, Ann Arbor, Ml, USA), 10 μg/ml insulin (Sigma-Aldrich) and 25 mM glucose for 24 hr. Relevant cellular samples were collected for subsequent qRT-PCR analysis.
Bone-marrow-derived macrophages
Request a detailed protocolFor isolation of bone-marrow-derived macrophages (BMDMs), tibias and femurs were removed from 8-week-old mice and flushed with media using a 26-gauge needle. Bone marrow was collected at 500 × g for 2 min at 4 °C, resuspended with complete DMEM medium and filtered through a 70 μm cell strainer. We then obtained the nonadherent cells and suspended them in BMDM culture medium (DMEM supplemented with 10% fetal bovine serum, 20 ng/ml M-CSF) at a concentration of 107 cells/ml in a total of 10 ml of medium. Fluid was changed every 2 days after inoculation until maturation on the 7th day.
Migration and invasion assays
Request a detailed protocolThe 3T3-L1 cells or differentiated SVF cells were evenly plated in 24-well plates. To differentiate mature cells, migration and invasion assays were performed using a transwell chamber (Millipore, Billerica, MA, USA). For the migration assay, RAW 264.7 macrophage cells were seeded in the upper chamber with serum-free medium (1.0×105 cells); the bottom chamber contained mature 3T3-L1 cells. For the invasion assay, the chamber was coated with Matrigel (BD Biosciences, Franklin Lakes, NJ, USA); the subsequent steps were similar to the migration assay. After the cells migrated or invaded for 24 hr, they were fixed and stained with crystal violet. Migrated and invaded RAW 264.7 cells were counted under an inverted light microscope. The number of migrated or invaded cells was quantified by counting the number of cells from 10 random fields at ×100 magnification.
RNA-sequencing analysis
Request a detailed protocolTotal RNA from epididymis white adipose tissue of wild type control mice (n=3) and Mir802 KI mice (n=3) was isolated using the RNeasy mini kit (QIAGEN) following the protocol. The quality of the samples, the experiment, and the analysis data was completely finished by the HaploX (Shangrao, China). Cuffdiff (v2.2.1) 51 was used to calculate the fragments per kilobase million (FPKM) for mRNAs in each group. A difference in gene expression with a <i>P-value ≤ 0.05 was considered significant. The raw data is presented in Supplementary file 1b. The RNA-seq raw data that support the findings of this study has been deposited in the NCBI’s Sequence Read Archive (SRA) database (PRJNA1021754).
Fluorescence in situ hybridization (FISH)
Request a detailed protocolCy3 labeled Mir802 probe was designed and synthesized by GenePharma (Shanghai, China). The frozen sections of adipose tissue from obese patients, normal persons or obese mice were fixed with 4% formaldehyde at room temperature for 10 min. The probe was hybridized at 37℃ for 16 hr. DAPI was added at 1:5000 for 15 min after washing with probe detergent. Images were obtained with confocal laser scanning microscope (CLSM, LSM800, Zeiss, Germany) and processed using the ZEN imaging software.
Plasmid and shRNA construction
Request a detailed protocolThe coding sequences for Traf3 (NM_001286122.1), Rel (NM_ 009045.5), Relb (001290457.2), Srebp1 (001313979.1) were amplified by PCR from full-length cDNA of mice, and then cloned in pcDNA 3.1 (+) vector (Addgene, Watertown, MA, USA). All plasmids were confirmed to be correct by sequencing. The primer sequences for PCR are listed in Supplementary file 1c.
The shRNA of Traf3, Srebp1, Rela, Relb were constructed in plvx-shRNA2 lentivirus vector (Takara). The plvx-shRNA2 lentivirus vector was digested with EcoR I and BamH I. The shRNA primer sequences are listed in Supplementary file 1d.
Luciferase assay
Request a detailed protocolMir802 mimics/Mir802 inhibitor (anti-Mir802)/miRNA NC (NC)/miRNA inhibitor NC was purchased from GenePharma (Shanghai, China). The construction of Traf3 (both wild type and mutants) was achieved by digestion of pmir-PGLO vector (Addgene, Watertown, MA, USA) with double restriction enzymes (Xhol I and Xbal I), followed by ligation of sequences encoding the corresponding 3’UTR of the target genes. Sequences of the synthetic oligonucleotides encoding the 3’UTR of the target genes and their mutants are listed in Supplementary file 1c. 3T3-L1 cells were transfected with one of the above-mentioned plasmids using Lipofectamine 2000 (Invitrogen), according to the manufacturer’s instructions. At 48 hr after transfection, the cells were lysed and the luciferase activity was assayed with a dual-luciferase reporter assay kit (Vazyme, Nanjing, China). Data are presented as the ratio of Renilla luciferase activity to firefly luciferase activity.
Flow cytometric analysis of macrophage polarization
Request a detailed protocolSVF cells were stained with 50 μL Zombie NIR (1:200 in PBS for analysis on a Cytek Aurora spectral flowwas cytometer, #423105, Biolegend) at room temperature for 10 min. Afterward, the cells were washed once with FACS buffer (1% BSA in 1×PBS) and centrifuged at 500×g for 5 min at 4 °C. The cells were incubated with 50 µL of FcBlock (1:100, BD Pharmigen in FACS buffer) at room for 10 min, and then an equal volume of a 2×stain cocktail was added and mixed. For flow cytometry analysis of macrophages, 1×106 freshly isolated cells were triple stained with CD11b-APC (#101211, Biolegend; 1:100), and F4/80-PE (#157304, Biolegend; 1:100), or stained with F4/80-FITC (#123108, Biolegend; 1:100), CD206-APC (#141708, Biolegend;1:100), CD86-PE (#105007, Biolegend; 1:100), or their isotype controls (Biolegend) on ice for 30 min in the dark. Samples were acquired on a 12-color Cytoflex (BD Biosciences) for each analysis. Dead cells were excluded from the analysis using Near-IR Live/Dead fixable staining reagent (BioLegend). Macrophages were identified as CD11b+F4/80+ and further as CD86+CD206- or CD86-CD206+ cells within this gate. Data were analyzed using FlowJo software version X.0.7 (Tree Star, Inc).
Mouse metabolic studies
Request a detailed protocolAfter 12 hr fasting treatment, mice fasting blood glucose (FBG) levels were examined via using a glucometer (OMRON, Japan) and fasting serum insulin (FINS) levels were tested by insulin ELISA kit (Crystal Chem, USA). And the homeostatic model assessment indices of insulin resistance (HOMA-IR) was calculated with the equation (FBG (mmol/l)×FINS (mIU/l))/22.5.
During Glucose Tolerance Test (GTT), Mir802 KO, Mir802 KI and control group mice were fasted for 12 hr (overnight) and then intraperitoneally (i.p.) injected with 2 g/kg glucose (Sigma-Aldrich, StLouis, MO, USA) of body weight. Blood glucose was determined from tail vein blood at the indicated time (0, 15, 30, 60, 90, and 120 min) after glucose injection or oral using a glucometer (ACCU-CHEK Perfor, Roche).
For Insulin Tolerance Test (ITT), the mice were fasted for 6 hr before 0.75 U/kg human insulin (Roche) of body weight i.p. injected. Blood glucose was determined from tail vein blood at the indicated time (0, 15, 30, 60, 90, and 120 min) after insulin injection or oral using a glucometer (ACCU-CHEK Perfor, Roche). Subtracting the baseline area, by subtracting the starting glucose value from the value at each time point, generates the area of the curve (AOC; Virtue and Vidal-Puig, 2021).
Body composition
Request a detailed protocolThe changes in body composition were assessed as we have previously described (Gordon et al., 2020). In brief, mice were anesthetized with 2% isoflurane by volume in a box and fixed on an MRI platform (Bruker BioSpec 7T/20 USR). Anesthesia was also maintained with isoflurane of 1% by volume. After turning on the instrument, the mice were scanned layer by layer according to the cross-section of their internal adipose tissue content, using ImageJ software analysis and statistics of the lipid distribution in mice.
RNA isolation and qRT-PCR analysis
Request a detailed protocolTotal RNA from adipose tissues or its fractions or 3T3-L1 cells was extracted with TRIzol reagent (Invitrogen). For mRNA expression analysis, 500 ng of total RNA was used for synthesis of cDNA using PrimeScriptTM RT reagent Kit (Takara, Tokyo, Japan). For miRNA expression analysis, 150 ng total RNA was reverse-transcription into cDNA using miRNA-specific primers supplied with TaqMan MicroRNA Reverse Transcription kit. The quantitative real-time PCR was performed using the LightCycle 480 (Roche). The relative level of gene expressions were calculated by the 2-ΔΔCT method, after normalization with the abundance of Rn18s or U6. For Mir802-5p and U6, TaqMan probes (Ambion) were used to confirm our results. The sequences of genes were listed in Supplementary file 1e.
Western blot analysis
Request a detailed protocolProteins were extracted from tissues or cells in radioimmunoprecipitation assay (RIPA) buffer (Beyotime) containing a complete protease inhibitor cocktail (Roche), resolved by SDSPAGE, transferred onto polyvinlidene fluoride (PVDF) membranes (Bio-Rad), and then probed with primary antibodies against TRAF3 (#ab36988), NIK (#ab314146) were from Abcam, NF-κB2 p100/p52 (#4882), NF-κB Rel (#8242) were from CST, and Relb (#A23389), NF-κB1 (#ab125611), IKK-α (#A2062), phospho-IKK-α (#AP0546), IKBα (#A19714), phosho-IKBα (#AP0614), iNOS (#A14031), phosho-Rel (#AP0123), Tubulin (#AC008), β-Actin (#AC038), and Histone H3 (#A2348) were from ABclonal. The protein bands were visualized with enhanced chemiluminescence reagents (GE Healthcare) and quantified by using the ImageJ software.
RNA immunoprecipitation (RIP)
Request a detailed protocolRNA immunoprecipitation was performed using an EZMagna RIP Kit (Millipore, Billerica, MA, USA) following the manufacturer’s protocol. 3T3-L1 cells transfected with Mir802 or oe-Traf3 were lysed in complete RIP lysis buffer, and then, 100 μl of whole cell extract was incubated with RIP buffer containing magnetic beads conjugated with anti-Ago2 (#ab186733, Abcam) antibody or negative control normal mouse IgG (#ab172730, Abcam). Furthermore, purified RNA was subjected to qRT-PCR analysis to demonstrate the presence of the binding targets using the respective primers. The primer sequences are listed in Supplementary file 1e.
Chromatin immunoprecipitation assay (ChIP)
Request a detailed protocolChIP experiments were strictly performed according to the manual for the ChIP Assay Kit (#17–10086, Millipore) and the manufacturer’s protocol. 3T3-L1 cells transfected with Mir802 mimics, Mir802 inhibitor, oe-Traf3, or Mir802 & oe-Traf3 were fixed with 37% formaldehyde for 10 min, followed by 30 rounds of sonication, each for 3 s, to fragment the chromatin. The chromatin was incubated with NF-κB Rel antibody (#8242, CST) at 4 °C overnight and then immunoprecipitated with Proteinase K (Millipore). Purified DNA was amplified by PCR using primer pairs that spanned the predicted Rela binding sites on the Srebp1a promoter. The primer sequences are listed in Supplementary file 1e.
Agarose-oligonucleotide pull-down assay
Request a detailed protocolThe oligonucleotides for the mouse Srebp1a promoter and their complementary strands were synthesized by GenePharma (Shanghai, China) and biotinylated using a Pierce Biotin 3' End DNA Labeling Kit (Cat. #89818; Thermo Fisher Scientific). These oligonucleotides were annealed to form double-stranded oligonucleotides, which were then incubated with streptavidin-conjugated agarose beads at 4 °C for 60 min and washed twice with IP lysis buffer. Next, the nuclear extract (50 μg each) in 200 μl IP lysis buffer was pre-cleared with agarose beads at 4 °C for 90 min to reduce any nonspecific binding and then incubated with oligo/streptavidin-conjugated beads at 4 °C overnight. The mixtures were washed three times with IP lysis buffer via centrifugation the following day, and the affinity-purified proteins were eluted by boiling in SDS sample buffer for 10 min. Samples were then subjected to analysis by western blot. Primer sequences are listed in Supplementary file 1e.
Histological and immunochemical analysis
Request a detailed protocolThe white adipose tissue was fixed in 4% formalin solution at 4 °C for 24 hr, embedded in paraffin, and sectioned at 5 μm. Deparaffinized and rehydrated sections were stained with hematoxylin and eosin (Sigma), or with reagents for Sirius red staining, or immunohistological staining of HCS LipidTOX red neutral stain (#H34467, Invitrogen) and F4/80 (#GB113373, Servicebio, china). The slides were analyzed using a confocal laser scanning microscope (CLSM, Carl Zeiss LSM800) at ×20 magnification.
Transmission electron microscopy
Request a detailed protocolFor transmission electron microscopy, mouse epiWAT was dissected, sliced into small fragments of 1–2 mm each, and then fixed in 5% glutaraldehyde for 2 days. Specimens were post-fixed in 1% osmium tetroxide. After staining with 2% aqueous uranyl acetate for 2 h, the samples were sequentially dehydrated in 50%, 70%, 90%, and 100% ethanol for 15 min respectively, then embedded in epoxy resin. Ultrathin sections were cut with an EM UC7 ultramicrotome (Leica) and poststained with lead nitrate. Ultrathin sections were mounted in formvar-coated nickel grids and observed under an FEI Tecnai G2 Electron Microscope (FEI Tecnai G2).
EdU labeling
Request a detailed protocolCell proliferation was detected with BeyoClick EdU Alexa Fluor 488 Imaging Kit (Beyotine, China). Briefly, 1×105 macrophage cells or 1×104 RAW264.7 cells were plated on 24-well plates. After co-cultured with 3T3-L1 cells or SVF cells, cells were gently washed twice with PBS, and further incubated with 10 μM EdU for 4 hr. Treated cells were fixed in 4% paraformaldehyde solution at room temperature for 15 min and EdU detection was carried out according to manufacturer’s instructions.
Sample size and replication
Request a detailed protocolSample size varied between experiments, depending on the number of mice allocated for each experiment. The minimum sample size was three.
Data inclusion/exclusion criteria
Request a detailed protocolAll patients enrolled in this study were obese (BMI >25 kg/m2). The negative controls were normal-weight individuals (20 kg/m2≤BMI ≤ 25 kg/m2). Data or samples were not excluded from analysis for other reasons.
Randomization
Request a detailed protocolMice used for the experiments were randomly selected and randomly assigned to experimental groups.
Blinding
Request a detailed protocolDuring experimentation and data acquisition, blinding was not applied to ensure tractability.
Statistical analysis
Request a detailed protocolAll in vivo experiments represent individual mice as biological replicates, the exact values of n are reported in figure legends. The in vitro cell assay was performed in triplicates, each experiment was independently replicated at least three times. Data are presented as mean ± SEM. Comparisons were performed using the Student’s t test between two groups or ANOVA in multiple groups. Dunn’s multiple comparisons for one-way ANOVA and Fisher’s least significant difference (LSD) for two-way ANOVA were used. The level of significance was set at *p<0.05, **p<0.01, ***p<0.001. Graphpad prism 8 (GraphPad, San Diego, CA, USA) was used for all calculations.
Data availability
Sequencing data have been deposited in the NCBI's Sequence Read Archive (SRA) database (PRJNA1021754).
-
NCBI BioProjectID PRJNA1021754. RNA-sequ raw data.
References
-
MicroRNA-mediated regulation of glucose and lipid metabolismNature Reviews. Molecular Cell Biology 22:425–438.https://doi.org/10.1038/s41580-021-00354-w
-
ROS/TNF-α crosstalk triggers the expression of IL-8 and MCP-1 in human monocytic THP-1 Cells via the NF-κB and ERK1/2 mediated signalingInternational Journal of Molecular Sciences 22:10519.https://doi.org/10.3390/ijms221910519
-
IKK-beta links inflammation to obesity-induced insulin resistanceNature Medicine 11:191–198.https://doi.org/10.1038/nm1185
-
MicroRNA regulatory networks in human adipose tissue and obesityNature Reviews. Endocrinology 11:276–288.https://doi.org/10.1038/nrendo.2015.25
-
TRAF3 controls activation of the canonical and alternative NFkappaB by the lymphotoxin beta receptorThe Journal of Biological Chemistry 285:12971–12978.https://doi.org/10.1074/jbc.M109.076091
-
Macrophage-mediated inflammation in metabolic diseaseNature Reviews. Immunology 11:738–749.https://doi.org/10.1038/nri3071
-
Noncanonical nf-κb signaling in health and diseaseTrends in Molecular Medicine 22:414–429.https://doi.org/10.1016/j.molmed.2016.03.002
-
MicroRNAs and metabolism crosstalk in energy homeostasisCell Metabolism 18:312–324.https://doi.org/10.1016/j.cmet.2013.06.004
-
Dysfunction of miR-802 in tumorsJournal of Clinical Laboratory Analysis 35:e23989.https://doi.org/10.1002/jcla.23989
-
Expanding TRAF function: TRAF3 as a tri-faced immune regulatorNature Reviews. Immunology 11:457–468.https://doi.org/10.1038/nri2998
-
TRAF3 forms heterotrimers with TRAF2 and modulates its ability to mediate NF-{kappa}B activationThe Journal of Biological Chemistry 279:55855–55865.https://doi.org/10.1074/jbc.M407284200
-
Specificity of TRAF3 in its negative regulation of the noncanonical NF-kappa B pathwayThe Journal of Biological Chemistry 282:3688–3694.https://doi.org/10.1074/jbc.M610271200
-
Why does obesity cause diabetes?Cell Metabolism 34:11–20.https://doi.org/10.1016/j.cmet.2021.12.012
-
Adipose tissue inflammation in the pathogenesis of type 2 diabetesCurrent Diabetes Reports 15:92.https://doi.org/10.1007/s11892-015-0670-x
-
Regulation of the NF-kappaB-inducing kinase by tumor necrosis factor receptor-associated factor 3-induced degradationThe Journal of Biological Chemistry 279:26243–26250.https://doi.org/10.1074/jbc.M403286200
-
Epigenetics in human obesity and type 2 diabetesCell Metabolism 29:1028–1044.https://doi.org/10.1016/j.cmet.2019.03.009
-
Obesity induces a phenotypic switch in adipose tissue macrophage polarizationThe Journal of Clinical Investigation 117:175–184.https://doi.org/10.1172/JCI29881
-
Osteopontin mediates obesity-induced adipose tissue macrophage infiltration and insulin resistance in miceThe Journal of Clinical Investigation 117:2877–2888.https://doi.org/10.1172/JCI31986
-
Defective FXR-SHP regulation in obesity aberrantly increases miR-802 expressionPromoting Insulin Resistance and Fatty Liver. Diabetes 70:733–744.https://doi.org/10.2337/db20-0856
-
SREBP-regulated lipid metabolism: convergent physiology - divergent pathophysiologyNature Reviews. Endocrinology 13:710–730.https://doi.org/10.1038/nrendo.2017.91
-
FoxO1 as a tissue-specific therapeutic target for type 2 diabetesFrontiers in Endocrinology 14:1286838.https://doi.org/10.3389/fendo.2023.1286838
-
GTTs and ITTs in mice: simple tests, complex answersNature Metabolism 3:883–886.https://doi.org/10.1038/s42255-021-00414-7
-
Obesity is associated with macrophage accumulation in adipose tissueThe Journal of Clinical Investigation 112:1796–1808.https://doi.org/10.1172/JCI19246
-
Chronic inflammation in fat plays a crucial role in the development of obesity-related insulin resistanceThe Journal of Clinical Investigation 112:1821–1830.https://doi.org/10.1172/JCI19451
-
Long-term exposure to low-dose Di(2-ethylhexyl) phthalate aggravated high fat diet-induced obesity in female miceEcotoxicology and Environmental Safety 253:114679.https://doi.org/10.1016/j.ecoenv.2023.114679
Article and author information
Author details
Funding
National Natural Science Foundation of China (82100858)
- Fangfang Zhang
National Natural Science Foundation of China (82373925)
- Liang Jin
National Natural Science Foundation of China (82073227)
- Yi Pan
Natural Science Foundation of Jiangsu Province (BK20221520)
- Liang Jin
Natural Science Foundation of Jiangsu Province (BK20200569)
- Fangfang Zhang
National Natural Science Foundation of China (82370804)
- Fangfang Zhang
National Natural Science Foundation of China (82070801)
- Liang Jin
Jiangsu Province Outstanding Postdoctoral Program (2023ZB342)
- Yue Yang
Jiangsu Province Research Founding for Postdoctoral (1412000016)
- Fangfang Zhang
‘111’ project (B16046)
- Liang Jin
Priority Academic Program Development of Jiangsu Higher Education Institutions (2632023TD03)
- Fangfang Zhang
Priority Academic Program Development of Jiangsu Higher Education Institutions (PAPD)
- Liang Jin
China Postdoctoral Science Foundation (2022T150726)
- Fangfang Zhang
Fundamental Research Funds for the Central Universities (2020M671661)
- Fangfang Zhang
Fundamental Research Funds for the Central Universities (2632023GR07)
- Yue Yang
The funders had no role in study design, data collection and interpretation, or the decision to submit the work for publication.
Acknowledgements
This work was supported by the National Natural Science Foundation of China: Grant No. 82100858, 82370804 (To FF Z), 82373925, 82070801 (To L J), 82073227 (To Y P). Supported by Natural Science Foundation of Jiangsu Province, BK20221520 (To L J), BK20200569 (To FF Z). Supported by grants from the ‘111’ project, B16046 (To L J). Supported by the Priority Academic Program Development of Jiangsu Higher Education Institutions, PAPD (To L J), 2632023TD03 (to FF Z). Supported by China Postdoctoral Science Foundation, 2022T150726, Supported by the Fundamental Research Funds for the Central Universities 2020M671661 (To FF Z) and 2632023GR07 (To Y Y). Supported by Jiangsu Province Research Founding for Postdoctoral, 1412000016 (To FF Z). Supported by Jiangsu Province Outstanding Postdoctoral Program (2023ZB342 to Y Y). We would like to thank Xiaonan Ma for providing technical assistance of Carl Zeiss LSM 800 on the Public Experimental Platform of China Pharmaceutical University. We thank Yumeng Shen (Public Platform of State Key Laboratory of Natural Medicines, China Pharmaceutical University) for her assistance with flow analysis. We thank LetPub (https://www.letpub.com) for its linguistic assistance during the preparation of this manuscript.
Ethics
All human subjects provided informed consent. All human studies were conducted according to the principles of the Declaration of Helsinki and were approved by the Ethics Committees of the Department Sir Run Run Hospital (Nanjing, China, 2023-SR-046).
Care of all animals was within institutional animal-care committee guidelines, and all procedures were approved by the animal ethics committee of China Pharmaceutical University (Permit Number: 2162326) and were in accordance with the international laws and policies (EEC Council Directive 86/609,1987).
Copyright
© 2024, Yang, Huang, Qin et al.
This article is distributed under the terms of the Creative Commons Attribution License, which permits unrestricted use and redistribution provided that the original author and source are credited.
Metrics
-
- 621
- views
-
- 91
- downloads
-
- 3
- citations
Views, downloads and citations are aggregated across all versions of this paper published by eLife.
Citations by DOI
-
- 3
- citations for umbrella DOI https://doi.org/10.7554/eLife.99162