fmo-4 promotes longevity and stress resistance via ER to mitochondria calcium regulation in C. elegans
eLife Assessment
This important study offers convincing evidence that fmo-4 plays essential roles in established lifespan interventions and downstream of its paralog fmo-2. The work is of substantial benefit for our understanding of this enzyme family, underscoring their importance in longevity and stress resistance. The study also suggests a connection between fmo-4 and dysregulation of calcium signalling, with conclusions and interpretations based on solid genetic methodology and evidence.
https://doi.org/10.7554/eLife.99971.3.sa0Important: Findings that have theoretical or practical implications beyond a single subfield
- Landmark
- Fundamental
- Important
- Valuable
- Useful
Convincing: Appropriate and validated methodology in line with current state-of-the-art
Solid: Methods, data and analyses broadly support the claims with only minor weaknesses
- Exceptional
- Compelling
- Convincing
- Solid
- Incomplete
- Inadequate
During the peer-review process the editor and reviewers write an eLife Assessment that summarises the significance of the findings reported in the article (on a scale ranging from landmark to useful) and the strength of the evidence (on a scale ranging from exceptional to inadequate). Learn more about eLife Assessments
Abstract
Flavin-containing monooxygenases (FMOs) are a conserved family of xenobiotic enzymes upregulated in multiple longevity interventions, including nematode and mouse models. Previous work supports that C. elegans fmo-2 promotes longevity, stress resistance, and healthspan by rewiring endogenous metabolism. However, there are five C. elegans FMOs and five mammalian FMOs, and it is not known whether promoting longevity and health benefits is a conserved role of this gene family. Here, we report that expression of C. elegans fmo-4 promotes lifespan extension and paraquat stress resistance downstream of both dietary restriction and inhibition of mTOR. We find that overexpression of fmo-4 in just the hypodermis is sufficient for these benefits, and that this expression significantly modifies the transcriptome. By analyzing changes in gene expression, we find that genes related to calcium signaling are significantly altered downstream of fmo-4 expression. Highlighting the importance of calcium homeostasis in this pathway, fmo-4 overexpressing animals are sensitive to thapsigargin, an ER stressor that inhibits calcium flux from the cytosol to the ER lumen. This calcium/fmo-4 interaction is solidified by data showing that modulating intracellular calcium with either small molecules or genetics can change expression of fmo-4 and/or interact with fmo-4 to affect lifespan and stress resistance. Further analysis supports a pathway where fmo-4 modulates calcium homeostasis downstream of activating transcription factor-6 (atf-6), whose knockdown induces and requires fmo-4 expression. Together, our data identify fmo-4 as a longevity-promoting gene whose actions interact with known longevity pathways and calcium homeostasis.
Introduction
Aging is a major risk factor for the onset of cancer, cardiovascular disease, dementia, and many other serious illnesses. Studying the aging process is crucial because a more comprehensive understanding can lead to the development of therapeutics that treat multiple diseases simultaneously. The use of model organisms, from yeast to mammals, has helped define genetic and environmental pathways that influence aging (Kenyon, 2010). Many of these pathways, such as dietary restriction (DR), defined as a decrease in nutrient intake without malnutrition, involve active modification of metabolism that robustly and reproducibly improve health and longevity across species (Bodkin et al., 2003; Swindell, 2012; Kapahi et al., 2017). Similarly, the response to oxygen deficiency, or hypoxic response, can lead to increased longevity, healthspan, and stress resistance (Shen et al., 2005). Interestingly, in C. elegans, both DR and the hypoxic response converge upon a single gene, fmo-2, that is necessary and sufficient to improve health and longevity (Leiser et al., 2015).
FMOs are a family of enzymes that use oxygen and NADPH to oxygenate nucleophilic substrates. FMOs oxygenate a wide array of xenobiotic substrates and were discovered ~50 y ago for their role in drug metabolism (Krueger and Williams, 2005; Rossner et al., 2017). Consequently, a majority of published data on FMOs relate to this role. Recently, studies have begun to focus on the endogenous role(s) of FMOs. Results show that multiple mammalian FMO proteins are involved in systemic metabolism (Steinbaugh et al., 2012; Choi et al., 2023). We initially discovered a role for C. elegans fmo-2 in regulating stress resistance and longevity downstream of DR and hypoxia and have continued to identify role(s) for FMO-2 in C. elegans metabolism and longevity (Choi et al., 2023). However, the conserved mechanisms for FMO-mediated health benefits are still unclear, as are the roles of individual FMO enzymes. Thus, to best understand how FMOs could be leveraged to improve health, we need to understand the conserved mechanism of these enzymes in longevity and metabolism.
There are five C. elegans FMOs, and three of the five share significant structural overlap with mammalian FMOs. This overlap involves a predicted endoplasmic reticulum (ER) localization and a membrane-spanning domain, which are each found in fmo-1, fmo-2, and fmo-4. In comparison to the more well-studied longevity gene fmo-2, fmo-4 in particular shares 88% identity in the catalytic domain amino acids, suggesting that FMO-2 and FMO-4 may bind similar substrates and could plausibly have overlapping endogenous roles. Published data also show that DR induces both fmo-2 and fmo-4 gene expression, implying a role for fmo-4 in longevity regulation (Rossner et al., 2017). Based on this, we hypothesized that fmo-4 may play a role in C. elegans longevity and that studying this role can help to understand this gene family and its relevance to aging.
FMOs are transmembrane enzymes thought to primarily reside in the ER. While mainly studied for its involvement in protein and lipid synthesis, the ER also plays a key role in metabolism and maintaining homeostasis within the cell. For instance, the ER responds to misfolded proteins by eliciting its unfolded protein response (UPRER), thereby removing dysfunctional proteins and restoring homeostasis (Read and Schröder, 2021). The ER is also responsible for storing and releasing calcium in a coordinated manner with the cytosol and mitochondria (Groenendyk et al., 2021). The ER establishes calcium homeostasis through processes involving (1) sequestering calcium in the lumen with the calreticulin chaperone (Groenendyk et al., 2021; Park et al., 2001), (2) releasing calcium into the cytosol through the inositol triphosphate receptor (IP3R) (Groenendyk et al., 2021; Berridge, 1993), and (3) bringing calcium into the ER lumen through the sarcoplasmic endoplasmic reticulum ATPase (SERCA) pump (Groenendyk et al., 2021; Marchi and Pinton, 2014). Interestingly, the ER’s role in regulating metabolic and calcium homeostasis in the cell has recently been linked to longevity in C. elegans. Knocking out activating transcription factor (atf)–6, one of the three branches of the UPRER, modulates calcium signaling from the ER to the mitochondria, resulting in increased mitochondrial turnover and lifespan extension in C. elegans (Burkewitz et al., 2020).
Given that (1) fmo-4 is induced by a longevity-promoting pathway (DR), (2) fmo-4 is predicted to be an ER transmembrane protein, and (3) the ER’s role in cellular homeostasis is linked to aging, we hypothesized that fmo-4 regulates longevity by modulating ER-related processes. Here, we test this hypothesis by modifying fmo-4 expression and interrogating interactions between fmo-4, stress resistance, longevity, and the ER. Our resulting data show that fmo-4 promotes longevity and paraquat stress resistance downstream of mTOR and DR through calcium homeostasis. We find that not only is fmo-4 a regulator of multiple longevity-promoting pathways, but it is also sufficient to extend lifespan and confer paraquat stress resistance when overexpressed either ubiquitously or in the hypodermis. Transcriptomics data and stress assays reveal that calcium signaling is altered downstream of fmo-4 expression, and that fmo-4 interacts with calcium regulation between the ER and mitochondria to promote longevity and paraquat stress resistance. Together, these results establish fmo-4 as a longevity-promoting gene and provide evidence as to how fmo-4 extends C. elegans lifespan through calcium-mediated ER to mitochondrial processes.
Results
Cefmo-4 is required for DR and mTOR-mediated lifespan extension
C. elegans fmo-4 shares significant similarities to its family member and longevity gene, fmo-2, including 88% conservation in catalytic residues (Figure 1—figure supplement 1), a transmembrane domain (Figure 1A) and predicted subcellular localization in the ER (Figure 1—figure supplement 2; Kishore et al., 2020). Additionally, fmo-4 is induced by the longevity intervention DR (Rossner et al., 2017). These structural similarities and DR-mediated induction led us to hypothesize that fmo-4 may play a role in aging. To test this, we first asked whether fmo-4 is required for well-studied longevity pathways, including DR. We utilized fmo-4(ok294) knockout (KO) animals (C. elegans Deletion Mutant Consortium, 2012) on five conditions reported to extend lifespan in C. elegans. Since fmo-4 is induced by dietary restriction, we started with fed and DR (sDR Greer and Brunet, 2009) conditions. Our results show that loss of fmo-4 has no significant effect on control-fed worms but prevents nearly all the lifespan extension seen under DR (Figure 1B). Wild-type (WT) worms on DR experience a ~35% lifespan extension compared to fed WT worms, but when fmo-4 is knocked out this extension is reduced to ~10% and this interaction is significant by Cox regression (p-value <4.50e–6). These data support that fmo-4 is required for the DR longevity pathway (Figure 1B). Having established this role, we continued lifespan analyses of fmo-4 KO worms exposed to RNAi knockdown of the S6-kinase gene rsks-1 (mTOR signaling; Pan et al., 2007), the Von Hippel-Lindau gene vhl-1 (hypoxic signaling; Leiser et al., 2013), the insulin receptor daf-2 (insulin-like signaling; Kimura et al., 1997), and the cytochrome c reductase gene cyc-1 (mitochondrial electron transport chain, cytochrome c reductase; Lee et al., 2010; Figure 1C–F). The resulting data show that fmo-4 is fully required for rsks-1-mediated longevity as marked by a complete abrogation of the rsks-1 RNAi lifespan extension when fmo-4 is knocked out (Figure 1C), placing fmo-4 downstream of mTOR. fmo-4 was not required for the hypoxic response (Figure 1D), insulin-like signaling (Figure 1E), or cytochrome c reductase inhibition pathways (Figure 1F) to increase lifespan. It is notable that, unlike fmo-4, fmo-2 is required for vhl-1-mediated longevity (Leiser et al., 2015). This result, coupled with fmo-4 and fmo-2 each being required for DR, suggests that these two genes in the same family are overlapping but distinct in their requirement. Together, these data suggest that fmo-4 plays a necessary role in C. elegans longevity regulation downstream of at least two pathways: DR and mTOR signaling.
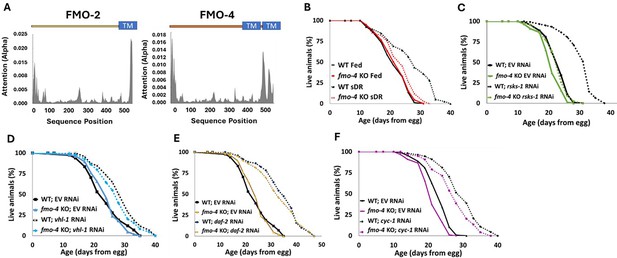
Cefmo-4 regulates mTOR and dietary restriction-mediated longevity.
(A) Hydropathy plots of FMO-2 and FMO-4 predicting endoplasmic reticulum (ER) transmembrane (TM) domains based on protein sequence analysis. Analysis was done using Deeploc-2.0. (B) Lifespan analysis of wild-type (WT) worms and fmo-4 knockout (fmo-4 KO) worms on fed and dietary restricted (DR) conditions. (C) Lifespan analysis of WT and fmo-4 KO worms on empty vector (EV) RNAi and rsks-1 RNAi. (D) Lifespan analysis of WT and fmo-4 KO worms on EV and vhl-1 RNAi. (E) Lifespan analysis of WT and fmo-4 KO worms on EV and daf-2 RNAi. (F) Lifespan analysis of WT and fmo-4 KO worms on EV and cyc-1 RNAi. For each lifespan, n = ~120 worms per condition per experiment, and three replicate experiments were performed. Significance was determined at p<0.05 using log-rank analysis and significant interactions between the condition of interest and genotype were determined at p<0.01 using Cox regression analysis. All replicate data can be found in Figure 1—source data 1; Figure 1—source data 2; Figure 1—source data 3; Figure 1—source data 4; Figure 1—source data 5.
-
Figure 1—source data 1
DR lifespan replicates.
- https://cdn.elifesciences.org/articles/99971/elife-99971-fig1-data1-v1.zip
-
Figure 1—source data 2
mTOR (rsks-1 RNAi) lifespan replicates.
- https://cdn.elifesciences.org/articles/99971/elife-99971-fig1-data2-v1.zip
-
Figure 1—source data 3
vhl-1 RNAi lifespan replicates.
- https://cdn.elifesciences.org/articles/99971/elife-99971-fig1-data3-v1.zip
-
Figure 1—source data 4
daf-2 RNAi lifespan replicates.
- https://cdn.elifesciences.org/articles/99971/elife-99971-fig1-data4-v1.zip
-
Figure 1—source data 5
cyc-1 RNAi lifespan replicates.
- https://cdn.elifesciences.org/articles/99971/elife-99971-fig1-data5-v1.zip
fmo-4 is required for fmo-2-mediated longevity, stress resistance, and healthspan
Having established that, like fmo-2, fmo-4 is required for DR-mediated longevity, we next hypothesized that fmo-2 and fmo-4 may be acting in the same pathway. To test this, we crossed fmo-2 overexpressing (OE) worms with fmo-4 KO worms to create an fmo-2 OE; fmo-4 KO strain (fmo-2OE;4KO). We validated this strain via qPCR analysis (Supplementary file 1). Upon measuring their lifespan compared to fmo-2 OE animals, we find that knocking out fmo-4 completely abrogates the lifespan extension from fmo-2 OE (Figure 2A). This result suggests that fmo-4 is required for fmo-2-mediated longevity and thus may act downstream of fmo-2 to promote longevity in C. elegans.
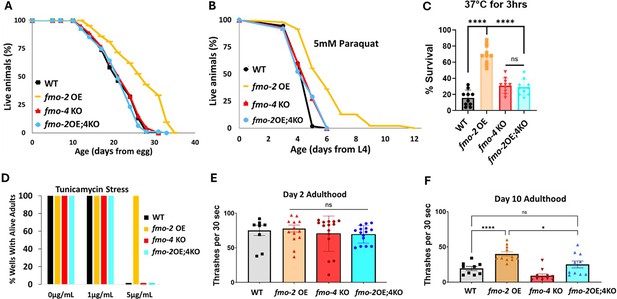
fmo-4 is required for the health benefits of fmo-2 overexpression.
(A) Lifespan analysis of wild-type (WT), fmo-2 overexpressing (fmo-2 OE), fmo-4 knockout (fmo-4 KO), and fmo-2 OE;fmo-4 KO (fmo-2OE;4KO) worms on E. coli OP50 (n = ~120 worms per condition, three replicate experiments). Significance was determined at p< 0.05 using log-rank analysis. (B) Survival of WT, fmo-2 OE, fmo-4 KO, and fmo-2OE;4KO worms exposed to 5 mM paraquat at L4 stage (n = ~90 worms per condition, three replicate experiments). Significance was determined at p<0.05 using log-rank analysis. (C) Survival of worms exposed to 37 °C heat shock for 3 hr (hours) at L4 stage (n=100 worms per condition, three replicate experiments). (D) Survival of worms exposed to 0, 1, and 5 µg/mL tunicamycin from the egg until day 1 of adulthood (n = ~60 eggs per condition, three replicate experiments). (E) Healthspan analysis of worm thrashing in a drop of M9 solution for 30 s on day 2 of adulthood (n=10 worms per condition, three replicate experiments). (F) Healthspan analysis of worm thrashing in a drop of M9 solution for 30 s on day 10 of adulthood (n=10 worms per condition, three replicate experiments). For heat stress, tunicamycin stress, and healthspan assessments, * denotes significant change at p<0.05 using unpaired two-tailed t-test or one-way ANOVA. N.S. = not significant. All replicate data can be found in Figure 2—source data 1; Figure 2—source data 2; Figure 2—source data 3; Figure 2—source data 4; Figure 2—source data 5.
-
Figure 2—source data 1
fmo-2 OE;4KO lifespan replicates.
- https://cdn.elifesciences.org/articles/99971/elife-99971-fig2-data1-v1.zip
-
Figure 2—source data 2
fmo-2 OE;4KO paraquat stress replicates.
- https://cdn.elifesciences.org/articles/99971/elife-99971-fig2-data2-v1.zip
-
Figure 2—source data 3
fmo-2 OE;4KO heat stress replicates.
- https://cdn.elifesciences.org/articles/99971/elife-99971-fig2-data3-v1.zip
-
Figure 2—source data 4
fmo-2 OE;4KO tunicamycin stress replicates.
- https://cdn.elifesciences.org/articles/99971/elife-99971-fig2-data4-v1.zip
-
Figure 2—source data 5
fmo-2 OE;4KO healthspan replicates.
- https://cdn.elifesciences.org/articles/99971/elife-99971-fig2-data5-v1.zip
We previously showed that fmo-2 OE is not just sufficient for lifespan extension but is also sufficient to promote resistance to multiple forms of stress, including oxidative stress (paraquat), heat stress, and ER stress (tunicamycin; Leiser et al., 2015). Since fmo-4 is required for fmo-2-mediated longevity, we hypothesized that fmo-4 would also be required for fmo-2-mediated stress resistance. Following exposure to 5 mM paraquat, 37 °C heat stress, or 5 µg/mL tunicamycin, we find that the fmo-2OE;4KO worms survive similarly to WT worms and are no longer resistant to these stresses (Figure 2B–D). Thus, knocking out fmo-4 blocks fmo-2-mediated broad stress resistance.
Healthspan is a crucial component to longevity, and we previously found that fmo-2 OE is sufficient to promote healthspan benefits with age in C. elegans (Leiser et al., 2015). As fmo-4 is required for both the lifespan extension and stress resistance observed with fmo-2 OE, we hypothesized that fmo-4 would also be required for fmo-2-mediated healthspan benefits. To test this, we measured the thrashing rate of fmo-2 OE;4KO worms at day 2 and day 10 of adulthood. We find that knocking out fmo-4 abrogates the healthspan benefits of the fmo-2 OE worms with age (Figure 2E, F). Thus, fmo-4 is required for fmo-2-mediated health and resilience benefits, further supporting its acting downstream of fmo-2 to promote health and longevity.
Overexpression of fmo-4 promotes longevity, healthspan, and paraquat stress resistance
While being necessary for increased healthspan, lifespan, and stress resistance shows that fmo-4 is required for these benefits, it does not test whether it can modulate aging directly. Therefore, to better understand fmo-4’s role in longevity and health, we next asked whether fmo-4 is sufficient for lifespan extension, stress resistance, and healthspan benefits. We created a ubiquitous fmo-4 OE worm strain with fmo-4 expressed under the eft-3 promoter via multicopy extrachromosomal array followed by random integration. qPCR analysis shows that fmo-4 is overexpressed ~150 fold over WT in the ubiquitous fmo-4 OE worms (Supplementary file 1), and their development time is slightly (~1.5 hr) but statistically significantly delayed compared to WT (Figure 3—figure supplement 1). Interestingly, we find that ubiquitous fmo-4 OE is sufficient to reproducibly extend lifespan by ~20% (Figure 3A). Epistatic analysis with fmo-2 shows that fmo-4 OE lifespan extension is not additive with fmo-2 OE (Figure 3—figure supplement 2A), as determined by comparing the individual OE strains to the fmo-2 OE;fmo-4 OE (fmo-2OE;4 OE) worm strain (Supplementary file 1). This further supports that these two genes in the same family act in the same pathway. To test whether fmo-4 requires fmo-2, we overexpressed fmo-4 in the context of fmo-2 knockout (fmo-4 OE;2KO) (Supplementary file 1). The results show that fmo-4 OE does not require fmo-2 for its longevity benefits (Figure 3—figure supplement 3A). This further validates that fmo-4 likely acts downstream of fmo-2.
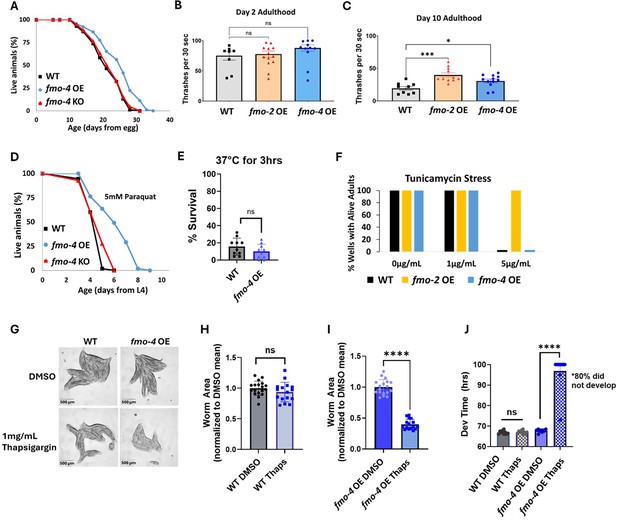
Overexpressing fmo-4 is sufficient for lifespan extension, paraquat stress resistance, and improved healthspan.
(A) Lifespan analysis of wild-type (WT), fmo-4 overexpressing (fmo-4 OE), and fmo-4 knockout (fmo-4 KO) worms on E. coli OP50 (n = ~120 worms per condition, three replicate experiments). Significance was determined at p<0.05 using log-rank analysis. (B) Healthspan analysis of worms thrashing in a drop of M9 solution for 30 s (seconds) on day 2 of adulthood (n=10 worms per condition, three replicate experiments). (C) Healthspan analysis of worms thrashing in a drop of M9 solution for 30 s on day 10 of adulthood (n=10 worms per condition, three replicate experiments). (D) Survival of worms exposed to 5 mM paraquat starting from L4 stage (n = ~90 worms per condition, three replicate experiments). Significance was determined at p<0.05 using log-rank analysis. (E) Survival of worms exposed to 37 °C for 3 hr (hours) at L4 stage (n=100 worms per condition, three replicate experiments). (F) Survival of worms exposed to 0, 1, and 5 µg/mL tunicamycin starting from the egg until day 1 of adulthood (n = ~60 eggs per condition, three replicate experiments). (G) Brightfield images of WT and ubiquitous fmo-4 OE worms exposed to DMSO or 1 mg/mL thapsigargin (n = ~20 worms per condition, three replicate experiments). Quantifications of (G) images in (H, I). (J) Development (Dev) time in hours (hrs) of WT and fmo-4 OE worms grown on DMSO control or 1 mg/mL thapsigargin (Thaps) from L2 stage (n=~10 worms per condition, three replicate experiments). * denotes significant change at p<0.05 using t-test. N.S. = not significant. All replicate data can be found in Figure 3—source data 1; Figure 3—source data 2; Figure 3—source data 3; Figure 3—source data 4; Figure 3—source data 5; Figure 3—source data 6; Figure 3—source data 7.
-
Figure 3—source data 1
mo-4 OE ubuitous lifespan replicates.
- https://cdn.elifesciences.org/articles/99971/elife-99971-fig3-data1-v1.zip
-
Figure 3—source data 2
fmo-4 OE ubiquitous healthspan replicates.
- https://cdn.elifesciences.org/articles/99971/elife-99971-fig3-data2-v1.zip
-
Figure 3—source data 3
fmo-4 OE ubuitous paraquat stress replicates.
- https://cdn.elifesciences.org/articles/99971/elife-99971-fig3-data3-v1.zip
-
Figure 3—source data 4
fmo-4 OEubuitous heat stress replicates.
- https://cdn.elifesciences.org/articles/99971/elife-99971-fig3-data4-v1.zip
-
Figure 3—source data 5
fmo-4 OE ubuitous tunicamycin stress replicates.
- https://cdn.elifesciences.org/articles/99971/elife-99971-fig3-data5-v1.zip
-
Figure 3—source data 6
fmo-4 OE ubuitous thapsigargin stress replicates.
- https://cdn.elifesciences.org/articles/99971/elife-99971-fig3-data6-v1.zip
-
Figure 3—source data 7
fmo-4 OE ubuitous thapsigargin development replicates.
- https://cdn.elifesciences.org/articles/99971/elife-99971-fig3-data7-v1.zip
Increased longevity is frequently observed in tandem with increased healthspan and resistance to toxic stress (Soo et al., 2023). We assessed the healthspan of the ubiquitous fmo-4 OE worms at days 2 and 10 of adulthood by measuring thrashing rates (movement). We find that ubiquitous fmo-4 OE is sufficient for an improvement (p=0.011) in healthspan benefits with age (Figure 3B, C). We next measured the stress resistance of the ubiquitous fmo-4 OE worms to 5 mM paraquat (oxidative stress), 37 °C heat stress, 5 µg/mL of tunicamycin (ER glycosylation stress), and 1 mg/mL thapsigargin (ER calcium stress). We included two ER stresses because FMO-4 is predicted to be an ER transmembrane protein. We find that ubiquitous fmo-4 OE is sufficient to convey paraquat stress resistance (Figure 3D) but does not offer any protection from heat (Figure 3E) or tunicamycin stress (Figure 3F). Interestingly, ubiquitous fmo-4 overexpressing animals are sensitive to thapsigargin, as shown by the worms’ significantly diminished size and inability to develop compared to WT control worms (Figure 3G–J). These results suggest a narrow stress resistance and even some sensitivity for fmo-4 in comparison to other longevity genes. To test whether fmo-4 OE would interact with fmo-2 OE, we combined the strains and measured their stress resistance. We find that the fmo-2 OE strain is still resistant to paraquat, heat, and tunicamycin stress in the presence of increased fmo-4, suggesting that overexpression of fmo-4 does not affect resistance to these stresses (Figure 3—figure supplement 2B–D). Interestingly, the fmo-2 OE strain is sensitive to thapsigargin stress in the presence of increased fmo-4, suggesting that fmo-4 likely also acts downstream of fmo-2 in this stress pathway (Figure 3—figure supplement 2E–I). Overall, these results suggest that fmo-4 (1) is sufficient to improve healthspan, (2) is both necessary and sufficient to promote paraquat resistance, (3) is necessary but not sufficient to improve heat and tunicamycin resistance, and (4) promotes sensitivity to calcium-mediated ER stress from thapsigargin. As expected under the hypothesis that fmo-4 acts downstream of fmo-2, the fmo-4OE;2KO strain phenocopies the fmo-4 OE strain in all stress assays (Figure 3—figure supplement 3B–I). Together, these data suggest that fmo-4 OE is sufficient to promote lifespan extension and paraquat stress resistance as well as a healthspan benefit, while exhibiting sensitivity to ER calcium stress.
Hypodermal overexpression of fmo-4 is sufficient for longevity and paraquat resistance
While using the ubiquitous overexpressing worm strain is helpful to probe into fmo-4’s involvement in longevity, stress resistance, and healthspan, it is also important to note that fmo-4 is normally localized and expressed primarily in the hypodermis (Figure 4A–C; Petalcorin et al., 2005). Thus, we asked whether hypodermal-specific fmo-4 overexpression is sufficient for these health benefits. We created a hypodermal-specific fmo-4 OE worm strain expressing fmo-4 under the dpy-7 promoter via multicopy extrachromosomal arrays followed by random integration. qPCR data show that fmo-4 is expressed ~45 fold over WT in the hypodermal-specific fmo-4 OE worms (Supplementary file 1), and their developmental time does not differ from WT (Figure 3—figure supplement 1). We measured lifespan, resistance to paraquat, heat, tunicamycin, and thapsigargin stress, as well as the healthspan of the hypodermal-specific fmo-4 OE worms (fmo-4 OEHyp). We find that the fmo-4 OEHyp strain exhibits a lifespan extension (Figure 4D), resistance to paraquat (Figure 4E) but not heat or tunicamycin stress (Figure 4F, G), and sensitivity to thapsigargin stress (Figure 4H–J). However, the fmo-4 OEHyp strain did not have a statistically significant effect on healthspan unlike the ubiquitous fmo-4 OE strain (Figure 4K, L). Overall, these results agree with the C. elegans single-cell atlas as well as previously published work (Petalcorin et al., 2005) showing that fmo-4 is mostly localized to the hypodermis (Figure 4A) and is consistent with the ubiquitous strain primarily benefiting from expressing additional fmo-4 in the hypodermis. Thus, ubiquitous or hypodermal-specific overexpression of fmo-4 is sufficient for longevity and paraquat stress resistance (Figures 3A, D, 4D, E), does not affect heat or tunicamycin resistance (Figure 3E, F, Figure 4F, G), and results in thapsigargin sensitivity (Figures 3G–J–4H–J).
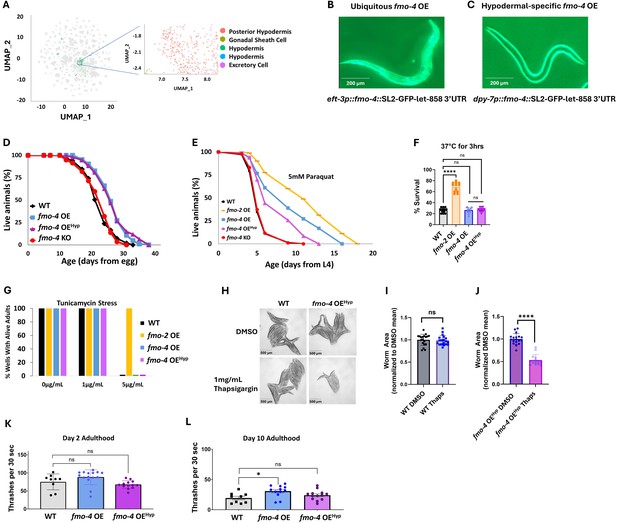
Overexpressing fmo-4 in the hypodermis is sufficient for lifespan extension and paraquat stress resistance.
(A) Complete cell atlas of C. elegans aging cluster map highlighting regions of fmo-4 gene expression. (B) Image of the ubiquitous fmo-4 overexpressing (fmo-4 OE) worm by the eft-3 promoter showing expression throughout its body. (C) Image of the hypodermal-specific fmo-4 OE worm by the dpy-7 promoter showing expression in the hypodermis. (D) Lifespan analysis of wild-type (WT), fmo-4 OE, hypodermal-specific fmo-4 OE (fmo-4 OEHyp), and fmo-4 knockout (fmo-4 KO) worms (n = ~120 worms per condition, three replicate experiments). Significance was determined at p<0.05 using log-rank analysis. (E) Survival of worms exposed to 5 mM paraquat starting at L4 stage (n = ~90 worms per condition, three replicate experiments). Significance was determined at p<0.05 using log-rank analysis. (F) Survival of worms exposed to 37 °C heat for 3 hr (hours) at L4 stage (n=100 worms per condition, three replicate experiments). (G) Survival of worms exposed to 0, 1, and 5 ug/mL tunicamycin starting at egg until day 1 of adulthood (n = ~60 eggs per condition, three replicate experiments). (H) Brightfield images of WT and fmo-4 OEHyp worms exposed to DMSO or 1 mg/mL thapsigargin (n = ~20 worms per condition, three replicate experiments). Quantification of (H) in (I–J). (K) Healthspan analysis of worms thrashing in a drop of M9 solution for 30 s (seconds) on day 2 of adulthood (n=10 worms per condition, three replicate experiments). (L) Healthspan analysis of worms thrashing in a drop of M9 solution for 30 s on day 10 of adulthood (n=10 worms per condition, three replicate experiments). For heat stress, tunicamycin stress, thapsigargin stress, and healthspan assessments, * denotes significant change at p<0.05 using t-test. N.S. = not significant. All replicate data can be found in Figure 4—source data 1; Figure 4—source data 2; Figure 4—source data 3; Figure 4—source data 4; Figure 4—source data 5; Figure 4—source data 6.
-
Figure 4—source data 1
fmo-4 OE hypodermal lifespan replicates.
- https://cdn.elifesciences.org/articles/99971/elife-99971-fig4-data1-v1.zip
-
Figure 4—source data 2
fmo-4 OE hypodermal paraquat stress replicates.
- https://cdn.elifesciences.org/articles/99971/elife-99971-fig4-data2-v1.zip
-
Figure 4—source data 3
fmo-4 OE hypodermal heat stress replicates.
- https://cdn.elifesciences.org/articles/99971/elife-99971-fig4-data3-v1.zip
-
Figure 4—source data 4
fmo-4 OE hypodermal tunicamycin stress replicates.
- https://cdn.elifesciences.org/articles/99971/elife-99971-fig4-data4-v1.zip
-
Figure 4—source data 5
fmo-4 OE hypodermal thapsigargin stress replicates.
- https://cdn.elifesciences.org/articles/99971/elife-99971-fig4-data5-v1.zip
-
Figure 4—source data 6
fmo-4 OE hypodermal healthspan replicates.
- https://cdn.elifesciences.org/articles/99971/elife-99971-fig4-data6-v1.zip
fmo-4 OE transcriptomics reveals a link to calcium regulation
To identify the downstream effects of fmo-4 expression, we analyzed the transcriptome of fmo-4 OE animals and compared them with control animals (Figure 5A). Based on a p-value of <0.05, we find ~800 transcripts are upregulated and ~500 transcripts are downregulated when fmo-4 is overexpressed (Source data 1). Of the upregulated and downregulated transcripts unique to the fmo-4 OE profile, we noticed that some of the significant pathways include transcription, Wnt signaling, TGFβ signaling, protein processing in the ER, and other subsets of signaling, as determined by the Database for Annotation, Visualization and Integrated Discovery (DAVID) Functional Annotation Bioinformatics Microarray Analysis (Figure 5A). Based on its known role as a xenobiotic enzyme, we were intrigued to find that fmo-4 OE is modulating signaling pathways. We were further intrigued to find that one of the signaling pathways affected by fmo-4 is calcium signaling (Source data 2). This was interesting considering that fmo-4 OE worms are sensitive to thapsigargin (Figure 3G–J), implicating an interaction between calcium signaling and fmo-4. To further verify this possibility, we used another tool called PANTHER Classification System Protein Class, which identified FMO-4 as a putative transmembrane signal receptor (G-protein coupled receptor), ion transporter, and/or calcium-binding protein (Source data 2). Together, these results support the possibility that FMO-4 is involved in calcium-related processes.
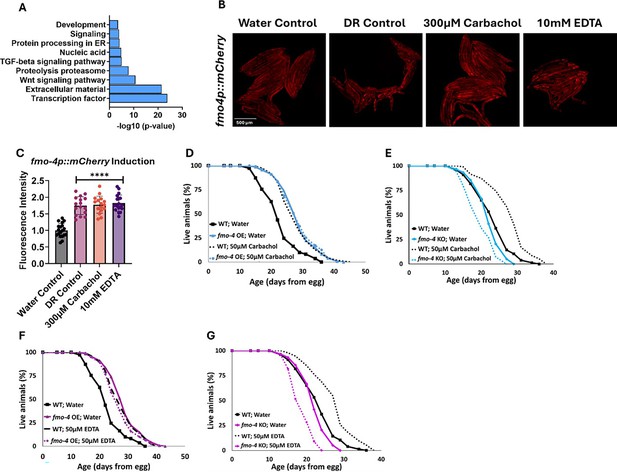
fmo-4 OE transcriptomics reveals a link to calcium regulation.
(A) Gene ontology (GO) analysis of significantly regulated pathways unique to fmo-4 overexpression (fmo-4 OE). (B) Fluorescence images of fmo-4p::mCherry reporter worms exposed to a water control, dietary restriction (DR) control, 300 µM carbachol, or 10 mM EDTA, which is quantified in (C) (n = ~20 worms per condition, three replicate experiments). (D) Lifespan assessment of wild-type (WT) and fmo-4 OE worms exposed to a water control or 50 µM carbachol. (E) Lifespan assessment of WT and fmo-4 knockout (fmo-4 KO) worms exposed to a water control or 50 µM carbachol (F) Lifespan assessment of WT and fmo-4 OE worms exposed to a water control or 50 µM EDTA. (G) Lifespan assessment of WT and fmo-4 KO worms exposed to a water control or 50 µM EDTA. For all lifespan analyses, n = ~120 worms per condition, three replicate experiments performed. Significance was determined at p<0.05 using log-rank analysis and significant interactions between the condition of interest and genotype was determined at p<0.01 using Cox regression analysis. For imaging experiments, * denotes significant change at p<0.05 using unpaired two-tailed t-test. N.S. = not significant. All replicate data can be found in Figure 5—source data 1; Figure 5—source data 2; Figure 5—source data 3.
-
Figure 5—source data 1
Carbachol and EDTA imaging replicates.
- https://cdn.elifesciences.org/articles/99971/elife-99971-fig5-data1-v1.zip
-
Figure 5—source data 2
Quantification of Carbachol and EDTA imaging replicates.
- https://cdn.elifesciences.org/articles/99971/elife-99971-fig5-data2-v1.zip
-
Figure 5—source data 3
Carbachol and EDTA lifespan replicates.
- https://cdn.elifesciences.org/articles/99971/elife-99971-fig5-data3-v1.zip
To begin exploring this interaction, we determined how changes in intracellular calcium levels impact fmo-4 expression and lifespan. We first manipulated calcium levels by supplementing the acetylcholine agonist, carbachol. Carbachol activates acetylcholine receptors, increasing overall intracellular calcium levels (Masoumi et al., 2023). We first validated that carbachol increases calcium levels by utilizing worms that neuronally express the calcium indicator GCaMP7f (SWF702; Dag et al., 2023). When these worms were exposed to 300 µM carbachol, a significant increase in GFP fluorescence was reported, indicating an increase in calcium levels (Figure 5—figure supplement 1A–D). We then measured fmo-4 gene expression upon exposure to carbachol using an fmo-4p::mCherry transcriptional reporter strain. Interestingly, we find that supplementation of 300 µM carbachol induces fmo-4 promoter expression fluorescence nearly twofold over a water control (Figure 5B, C). This was similar to the level of induction of fmo-4 in fasted (DR-like) conditions (Figure 5B, C). We postulate that fmo-4 is induced by carbachol because increased intracellular calcium activates fmo-4 gene expression. Since carbachol supplementation induces fmo-4, we hypothesized that carbachol may affect lifespan and would interact with fmo-4. We measured the lifespan of worms supplemented with 50 µM carbachol and find that while WT lifespan is significantly extended by carbachol, this extension is not additive with fmo-4 OE (Figure 5D), and requires fmo-4, as the fmo-4 KO is shorter-lived when exposed to carbachol (Figure 5E).
To test whether reducing calcium has an opposing effect to increasing it, we utilized EthyleneDiamineTetraAcetic acid (EDTA) to deplete calcium levels (Mellau and Jørgensen, 2003). We validated that EDTA depletes calcium levels by exposing GCaMP7f expressing worms (Dag et al., 2023) to 10 mM EDTA and we observed a significant decrease in GFP fluorescence, indicating a decrease in calcium levels (Figure 5—figure supplement 1A, B, E, F). Then, we placed fmo-4p::mCherry reporter worms on plates containing 10 mM EDTA and measured fluorescence. Surprisingly, we find that EDTA also significantly induces fmo-4 expression (Figure 5B, C). We then assessed the survival of WT, fmo-4 OE and KO worms on plates supplemented with 50 µM EDTA. We find that EDTA significantly extends WT lifespan without further extending fmo-4 OE lifespan (Figure 5F), while shortening the lifespan of fmo-4 KO animals (Figure 5G). Overall, these data suggest that fmo-4 is highly sensitive to changing calcium levels in either direction, and that both increasing and depleting calcium (1) induces fmo-4, (2) extends WT lifespan, (3) is not additive with fmo-4 OE lifespan, and (4) is deleterious to fmo-4 KO animals. Thus, these results support an interaction between fmo-4 and calcium signaling.
fmo-4 genetically interacts with calcium signaling to promote longevity and paraquat resistance
Having established an interaction between fmo-4 and calcium perturbations, we next asked if fmo-4 interacts with genes involved in calcium signaling. These genes include calreticulin (crt-1), inositol triphosphate receptor (IP3R, itr-1), and the mitochondrial calcium uniporter (mcu-1; Burkewitz et al., 2020). Calreticulin is responsible for binding and sequestering calcium in the ER lumen (Groenendyk et al., 2021; Park et al., 2001). It was previously shown that FMO protein extracted from rabbit lung can form a complex with calreticulin, suggesting a physical interaction (Guan et al., 1991). When testing for genetic interactions between crt-1 and fmo-4, we find that crt-1 RNAi extends WT lifespan, as previously reported (Burkewitz et al., 2020), and that this lifespan extension is not additive with fmo-4 OE (Figure 6A). Treating fmo-4 KO worms with crt-1 RNAi can significantly extend lifespan (Figure 6—figure supplement 1A), but this result was inconsistent (Source data 3). Overall, this suggests that fmo-4 and crt-1 are acting in the same genetic pathway. IP3R, or itr-1 in C. elegans, is located in the ER membrane and is critical for exporting calcium from the ER lumen into the cytosol (Groenendyk et al., 2021; Berridge, 1993). Previous results suggest that loss of itr-1 decreases WT lifespan (Burkewitz et al., 2020), which our results confirm (Figure 6B). Importantly, we also find that itr-1 RNAi decreases lifespan of fmo-4 KO worms similarly (Figure 6—figure supplement 1B) and fmo-4 OE (Figure 6B) worms to a greater extent than WT, negating the relative longevity of fmo-4 OE worms compared to WT. Thus, itr-1 is required to increase lifespan downstream of fmo-4. MCU (mcu-1) is located in the inner mitochondrial membrane and allows cytosolic calcium into the inner mitochondrial matrix (Groenendyk et al., 2021; Marchi and Pinton, 2014). We also find that mcu-1 is required downstream of fmo-4 OE, as mcu-1 RNAi abrogates the fmo-4 OE lifespan (Figure 6C). The fmo-4 KO lifespan is slightly decreased on mcu-1 RNAi (Figure 6—figure supplement 1C). These data support that fmo-4 extends lifespan through its interactions with the calcium signaling genes, crt-1, itr-1, and mcu-1.
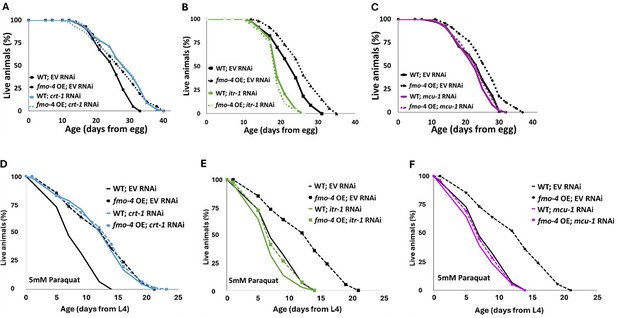
fmo-4 interacts with calcium signaling genes to promote longevity and paraquat resistance.
(A) Survival analysis of wild-type (WT) and fmo-4 overexpressing (fmo-4 OE) worms on empty vector (EV) and crt-1 RNAi. (B) Survival analysis of worms on EV and itr-1 RNAi. (C) Survival analysis of worms on EV and mcu-1 RNAi. For all lifespan analyses, n = ~120 worms per condition, three replicate experiments were performed. (D) Survival of WT and fmo-4 OE worms on EV and crt-1 RNAi exposed to 5 mM paraquat at L4 stage. (E) Survival of worms on EV and itr-1 RNAi exposed to 5 mM paraquat at L4 stage. (F) Survival of worms on EV and mcu-1 RNAi exposed to 5 mM paraquat at L4 stage. For all paraquat survival assays, n = ~90 worms per condition, three replicate experiments performed. Significance was determined at p<0.05 using log-rank analysis and significant interactions between the condition of interest and genotype were determined at p <0.01 using Cox regression analysis. All replicate data can be found in Figure 6—source data 1; Figure 6—source data 2; Figure 6—source data 3; Figure 6—source data 4.
-
Figure 6—source data 1
fmo-4 OE worms on crt-1 RNAi lifespan replicates.
- https://cdn.elifesciences.org/articles/99971/elife-99971-fig6-data1-v1.zip
-
Figure 6—source data 2
fmo-4 OE worms on itr-1 RNAi lifespan replicates.
- https://cdn.elifesciences.org/articles/99971/elife-99971-fig6-data2-v1.zip
-
Figure 6—source data 3
fmo-4 OE worms on mcu-1 RNAi lifespan replicates.
- https://cdn.elifesciences.org/articles/99971/elife-99971-fig6-data3-v1.zip
-
Figure 6—source data 4
Paraquat stress replicates of fmo-4 OE worms on crt-1, itr-1 and mcu-1 RNAi.
- https://cdn.elifesciences.org/articles/99971/elife-99971-fig6-data4-v1.zip
In addition to lifespan assessment, we were curious whether these genes also interact with fmo-4 expression to modify resistance to paraquat. We exposed WT, fmo-4 OE, and fmo-4 KO worms to 5 mM paraquat plates with RNAi for crt-1, itr-1 or mcu-1 and assessed survival over time. Interestingly, we find that crt-1 RNAi promotes resistance in WT worms and this effect is not additive in fmo-4 OE worms (Figure 6D). Furthermore, crt-1 RNAi extends the lifespan of fmo-4 KO worms (Figure 6—figure supplement 1D), suggesting that crt-1 and fmo-4 act in the same pathway and that crt-1 may act downstream of fmo-4 to promote paraquat stress resistance. Neither itr-1 RNAi nor mcu-1 RNAi confers resistance to paraquat, and fmo-4 OE resistance is lost when exposed to these RNAi (Figure 6E, F). The fmo-4 KO worms show a decrease in paraquat survival when treated with itr-1 and mcu-1 RNAi (Figure 6—figure supplement 1E, F). We also measured paraquat stress resistance of fmo-4 OE and KO worms exposed to RNAi of the voltage-dependent anion channel 1 (vdac-1), which is located in the outer mitochondrial membrane and also regulates calcium flow into and out of the mitochondria (Shoshan-Barmatz et al., 2018). Interestingly, fmo-4 OE resistance is lost when exposed to vdac-1 RNAi and the fmo-4 KO worms show no difference in paraquat survival compared to the EV RNAi control (Figure 6—figure supplement 1G, H). These data support a model where fmo-4 OE converges onto a shared pathway mediating paraquat stress resistance and longevity regulation. Together, we conclude that fmo-4 interacts with ER to mitochondrial calcium signaling through crt-1, itr-1, and mcu-1, to promote longevity and paraquat stress resistance.
atf-6 KD regulates fmo-4-mediated longevity and paraquat resistance
Our data establish that fmo-4 interacts with ER and mitochondrial calcium signaling to promote lifespan extension and resistance to paraquat. A previous study linked many of these components to a major regulator of UPRER, activating transcription factor-6 (atf-6) (Burkewitz et al., 2020). When atf-6 is lost, lifespan is extended through changes in calcium signaling between the ER and mitochondria requiring crt-1, itr-1, and mcu-1 (Burkewitz et al., 2020). Based on our transcriptomics data and fmo-4’s interactions with calcium signaling genes, we hypothesized that fmo-4 could interact with atf-6 to promote longevity and paraquat resistance. To test whether atf-6 is regulating fmo-4, we utilized our fmo-4p::mCherry transcriptional reporter strain. We measured fmo-4 gene expression after RNAi knockdown of atf-6 and find that fmo-4p::mCherry worms on atf-6 RNAi show a consistent ~two fold increase in fluorescence (Figure 7A, B), similar to what we observe from calcium perturbations (Figure 5B, C). Since atf-6 is one of three branches of UPRER, we were curious to see if fmo-4 interacts specifically with atf-6 or also with the other two branches, ire-1/xbp-1 and pek-1/atf-4 (Read and Schröder, 2021). We treated the fmo-4p::mCherry reporter worms with ire-1, xbp-1, pek-1, or atf-4 RNAi and measured fluorescence. We find that knocking down the components of the IRE-1 or PEK-1 branches does not induce fmo-4 gene expression (Figure 7—figure supplement 1A, B). Thus, only knockdown of the ATF-6 branch of UPRER induces fmo-4 expression.
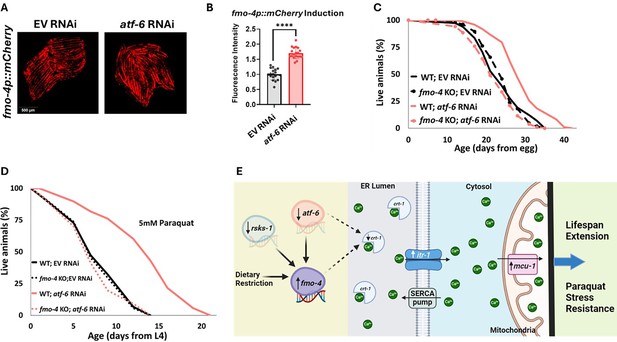
atf-6 KD regulates fmo-4-mediated longevity and paraquat stress resistance.
(A) Fluorescence imaging of the fmo-4p::mCherry reporter worms on empty vector (EV) and atf-6 RNAi. (B) Quantification of (A) (n = ~20 worms per condition, three replicate experiments). (C) Lifespan analysis of wild-type (WT) and fmo-4 knockout (fmo-4 KO) worms on EV and atf-6 RNAi (n = ~120 worms per condition, three replicate experiments). (D) Survival of WT and fmo-4 KO worms on EV and atf-6 RNAi exposed to 5 mM paraquat at L4 stage (n = ~90 worms per condition, three replicate experiments). Significance was determined at p<0.05 using log-rank analysis and significant interactions between the condition of interest and genotype was determined at p<0.01 using Cox regression analysis. (E) Working model showing that fmo-4 acts downstream of multiple longevity promoters including dietary restriction, reduction in rsks-1, and reduction in atf-6. Reduced expression of atf-6 induces fmo-4 expression, which regulates calcium regulation from the endoplasmic reticulum (ER) to the mitochondria to promote lifespan extension and paraquat stress resistance. For imaging experiments, * denotes significant change at p<0.05 using unpaired two-tailed t test. N.S. = not significant. All replicate data can be found in Figure 7—source data 1; Figure 7—source data 2; Figure 7—source data 3; Figure 7—source data 4.
-
Figure 7—source data 1
atf-6 RNAi imaging replicates.
- https://cdn.elifesciences.org/articles/99971/elife-99971-fig7-data1-v1.zip
-
Figure 7—source data 2
Quantification of atf-6 RNAi imaging replicates.
- https://cdn.elifesciences.org/articles/99971/elife-99971-fig7-data2-v1.zip
-
Figure 7—source data 3
fmo-4 KO worms on atf-6 RNAi lifespan replicates.
- https://cdn.elifesciences.org/articles/99971/elife-99971-fig7-data3-v1.zip
-
Figure 7—source data 4
fmo-4 KO worms on atf-6 RNAi paraquat stress replicates.
- https://cdn.elifesciences.org/articles/99971/elife-99971-fig7-data4-v1.zip
We hypothesized that atf-6 limits fmo-4 expression and thus fmo-4 acts downstream of atf-6 knockdown to modulate lifespan extension. To test this, we assessed survival and find that while atf-6 RNAi extends the lifespan of WT worms, as reported (Burkewitz et al., 2020), this effect is abrogated when fmo-4 is knocked out (Figure 7C). This result suggests that fmo-4 is indeed acting downstream of atf-6 to promote longevity. To determine if atf-6 is also involved in fmo-4-mediated paraquat resistance, we exposed WT and fmo-4 KO worms to 5 mM paraquat plates with atf-6 RNAi. We find that atf-6 RNAi promotes stress resistance to paraquat, but this effect is lost when fmo-4 is knocked out, supporting the hypothesis that atf-6 is involved in fmo-4-mediated paraquat resistance (Figure 7D). Together, these data suggest that fmo-4 modulates lifespan and paraquat stress resistance downstream of the reduction in atf-6, ultimately regulating ER to mitochondria calcium signaling (Figure 7E).
Discussion
Together, our data present a model where fmo-4 acts downstream of DR and mTOR to positively affect healthspan and longevity (Figure 1B–C). fmo-4 overexpression is sufficient to provide these benefits (Figure 3) and does not require the DR-mediating family member, fmo-2 (Figure 2). Our results also suggest that fmo-4 gene expression is upregulated upon changes in intracellular calcium, and that fmo-4 interacts with calcium signaling through key genes like crt-1, itr-1, and mcu-1, to promote longevity and paraquat stress resistance (Figures 5–6). The relationship between fmo-4 and calcium is further illustrated by fmo-4 OE’s susceptibility to thapsigargin, a calcium-mediated ER stress. Furthermore, we find that knocking down the UPRER transcription factor and calcium regulator, atf-6, induces fmo-4 gene expression and requires fmo-4 to promote longevity (Figure 7). Collectively, our data suggest that fmo-4 promotes longevity and paraquat stress resistance by regulating calcium homeostasis between the ER and mitochondria (Figure 7E).
Previous studies from our lab have elucidated how C. elegans fmo-2 promotes longevity through endogenous metabolism (Choi et al., 2023). Based on the conservation within the fmo gene family and that multiple Fmos are induced in long-lived worms and mammals, it was reasonable to hypothesize that Fmos could play overlapping roles in longevity regulation. Our data here support the broader hypothesis that Fmos have a conserved role in aging, but interestingly, that the mechanisms by which they modulate aging are separable. Importantly, we find that fmo-4 is required for multiple longevity-promoting pathways, including DR and mTOR, but is not required for lifespan extension through the hypoxic response, insulin-like signaling, or cytochrome c reductase pathways. We also find that fmo-4 does not require fmo-2, but that fmo-2 does require fmo-4. This is interesting because not only do these data tell us that fmo-4 is important in the context of longevity, but also that it acts distinctly from fmo-2.
After creating ubiquitous and hypodermal-specific fmo-4 overexpressing strains, we find that fmo-4 is sufficient for longevity and paraquat stress resistance but not heat, tunicamycin, or thapsigargin stress. These results further differentiate fmo-4 from fmo-2 and also provide insight into fmo-4’s potential roles in the cell. For instance, fmo-4 OE worms’ resistance to paraquat could suggest a role in responding to or controlling reactive oxygen species (ROS) production in the mitochondria. Additionally, fmo-4 OE worms’ sensitivity to thapsigargin likely points towards an involvement in ER calcium regulation. These are both interesting plausible roles for fmo-4, and may go hand in hand, as calcium levels are known to impact ROS levels (Görlach et al., 2015), and changes in ROS levels are known to impact ER calcium release (Görlach et al., 2015). Based on this and the data with calcium perturbations, we speculate that FMO-4 acts as a calcium sensor, and when calcium levels are too high or too low, FMO-4 responds by regulating downstream calcium signaling proteins to restore homeostasis in the cell. As FMO-4 is a predicted ER transmembrane protein, we hypothesize that FMO-4 is sensing changes in calcium levels in the ER and/or cytosol.
We note that our data reveal genetic interactions between fmo-4 and other longevity pathways like DR, mTOR, FMO-2, and ATF-6. However, it is important to also understand on the protein level how FMO-4 is interacting with these pathways and how FMO-4 is regulating downstream calcium signaling components to promote longevity and paraquat stress resistance. For instance, it was previously shown that rabbit FMO protein forms a complex with calreticulin (Guan et al., 1991). It is possible that an increase in FMO-4 leads to more binding between FMO-4 and calreticulin, which ultimately prevents calreticulin from binding ER calcium. This would then allow for proper calcium flux from the ER to the mitochondria via the IP3R and MCU, respectively. Our future work will look into these protein interactions so that we can further tease apart the FMO-4-mediated longevity pathway. We also note that we have utilized RNAi knockdown rather than knockout mutants to assess these genetic interactions with fmo-4. In particular, we were wary of knocking out crucial calcium regulating genes, like itr-1 and mcu-1, that already result in some level of sickness in the worms when knocked down (Figure 6B) or could potentially lead to other confounding metabolic changes if knocked out. We were able to obtain robust and reproducible results using RNAi knockdown, but recognize the caveats that come with not testing full deletion mutants.
While our transcriptomics analysis and calcium manipulations suggest fmo-4 involvement in calcium regulation, there are other measures that can better assess an interaction between fmo-4 and calcium. Carbachol and EDTA supplementation are not perfect assessments of altered calcium levels, as carbachol does not act in every tissue and EDTA may be depleting more than just calcium from the cell. Thus, future studies should measure calcium levels and calcium flux in fmo-4 OE and KO worms using GCaMP expressing worms to determine the direct link between fmo-4 expression and calcium in the cell. Additionally, to determine if FMO-4 is acting as a calcium sensor or interacting with one to regulate calcium homeostasis, development of FMO-4 antibodies for immunoprecipitation assays would be useful (Kaufmann and Sauter, 2017). Together, it will be important to establish the biochemical parameters of the FMO-4 calcium interaction.
As calcium signaling occurs between the ER and the mitochondria, our results could have interesting implications in ER-mitochondrial metabolism. We would expect that FMO-4 regulates ER-mitochondria metabolism because (1) FMO-4 is a predicted ER transmembrane protein and blocking the expression of another ER transmembrane protein, ATF-6, induces the expression of fmo-4, (2) fmo-4 OE worms are highly sensitive to ER calcium stress but resistant to ROS induced stress, (3) lifespan extension driven by fmo-4 depends on mitochondrial calcium import (mcu-1), and (4) Fmo gene expression is induced by mitochondrial inhibitors (Huang et al., 2021). Additionally, changes in mitochondrial calcium import affect various mitochondrial measures like respiration, dynamics (i.e. fission and fusion), membrane potential, and TCA cycle activity (Duchen, 2000). Future studies will delve into how fmo-4 perturbations impact each of these measures and if they act in the fmo-4-mediated longevity pathway. Based on our data, we speculate that fmo-4 OE may increase mitochondrial calcium by regulating mcu-1 activity, and that fmo-4 OE worms may in turn be regulating ROS production in the mitochondria. We hypothesize that changes in fmo-4 expression will alter mitochondrial respiration, dynamics, membrane potential, and TCA cycle activity. Taken together, future experiments will shed light on the role that FMOs play in calcium regulation and mitochondrial metabolism and will help to further tease apart the FMO-mediated longevity pathway.
Materials and methods
Strains and growth conditions
Request a detailed protocolStandard C. elegans cultivation procedures were used as previously described (Leiser et al., 2015). Briefly, all worm strains were maintained on solid nematode growth media (NGM) using E. coli OP50 throughout life except where double stranded (ds) RNAi (E. coli HT115) were used. Worms were transferred using a platinum wire. All worm strains were kept at 20 °C. RNAi used is listed in Supplementary file 3. Worm strains are listed in Supplementary file 4. Genotyping primers are listed in Supplementary file 5.
Development assays
Request a detailed protocolAnimals were synchronized by placing 10 gravid adult worms on NGM plates seeded with E. coli OP50 to lay eggs for 1 hr at 20 °C. The gravid adult worms were then removed, and the eggs were allowed to hatch and develop at 20 °C until larval stage 2 (L2). At this point the L2 worms were moved to individual 35 mm NGM plates seeded with E. coli OP50, one worm per plate. This was done for each worm strain tested and ten total 35 mm plates per worm strain were prepared. The worms were then followed through development and watched hourly during young adulthood to score the time of the first egg lay, as previously described (Leiser et al., 2015). Development time was reported in hours since egg lay. Three replicate experiments were performed. For development on DMSO control or thapsigargin, the same protocol was followed except that the L2 worms were moved to individual 35 mm NGM plates seeded with E. coli OP50 that were spotted with either DMSO or 1 mg/mL thapsigargin, one worm per plate.
Stress resistance assays
Paraquat stress assay
Request a detailed protocolParaquat (Methyl viologen dichloride hydrate, 856177, Sigma-Aldrich) was used to induce oxidative stress. Worms were synchronized from eggs on either NGM plates seeded with E. coli OP50 or RNAi plates seeded with HT115 strain expressing dsRNAi for a particular gene. At L4 stage, 30 worms were transferred to either NGM plates or RNAi-FUdR (40690016, Bioworld) plates containing 5 mM paraquat dissolved in water. Three plates per strain per condition were prepared, for a total of 90 worms per condition. As previously described, worms were then scored every other day and considered dead when they did not move in response to prodding under a dissection microscope (Leiser et al., 2015). Worms that crawled off the plate were not considered, but ruptured worms were noted and considered. Three replicate experiments were performed. Log-rank test was used to derive p-value for paraquat stress resistance survival assays using p<0.05 cut-off threshold compared to EV or wild-type controls. Cox regression was also used to assess interactions between genotype and condition for paraquat stress resistance survival assays using p<0.01 cut-off threshold compared to controls.
Heat stress assay
Request a detailed protocolWorms were synchronized from eggs on NGM plates seeded with E. coli OP50. Once the worms reached day 1 of adulthood, 25 worms of each strain were transferred to 35 mm NGM plates seeded with E. coli OP50 (Burkewitz et al., 2020). Four plates per strain per condition were prepared, for a total of 100 worms per condition. The plates were placed in a single layer on a tray and incubated at 37 °C for 3 hr. After 3 hr, the plates were removed and placed at 20 °C for 24 hr to give the worms time to recover. After 24 hr, the number of dead and alive worms was counted. % Alive was calculated as (# alive/# total)×100 and graphed in Graphpad Prism using unpaired two-tailed t tests with Welch’s correction as well as one-way ANOVA to determine significance. Three replicate experiments were performed.
Tunicamycin stress assay
Request a detailed protocolE. coli OP50 was cultured at 37 °C shaking overnight and then centrifuged and concentrated to 100 x. 100 µL of bacteria was added to 9.9 mL of S-media to create a 1 x mixture. 100 µL of this bacteria/S-media mixture was added to the wells of a Falcon non tissue culture treated clear flat bottom 96 well plate (Falcon, 351172). Approximately 20 eggs per worm strain were added to the wells of the 96 well plate as previously described (Leiser et al., 2015). Three replicates per worm strain were added to the 96-well plate, for a total of 60 eggs per worm strain. Then either 0, 1, 2, or 5 µg/mL of tunicamycin (Sigma, T7765) diluted in dimethyl sulfoxide (DMSO) (Sigma, D2650) was added to the wells. Total volume per well was 100 µL. The plate was incubated covered at room temperature on an orbital shaker for 72 hr. After 72 hr, each well containing worms was rinsed with 100 µL M9 and then added to individual 35 mm NGM plates seeded with E. coli OP50 to sit covered at room temperature overnight. The following day, plates were assessed for live adults. % Alive Adults was calculated by determining how many of the three technical replicates of a given strain showed at least one live adult and then graphed in Microsoft Excel. Three replicate experiments were performed.
Thapsigargin stress assay
Request a detailed protocolTo assess development of worms during chronic ER calcium stress, NGM plates seeded with E. coli OP50 were spotted with 25 µL 1 mg/mL thapsigargin in DMSO (Sigma) or DMSO only directly on to the E. coli OP50 lawns and allowed to dry overnight. Then, 30 synchronized L1’s per worm strain were added directly to the spotted lawns (Burkewitz et al., 2020). Forty-eight hours later, worms were picked off these plates, added to a 2% agarose pad on a glass slide, anesthetized in 0.5 M sodium azide (Sigma), and imaged at 6.3 x magnification (brightfield) with the LASx software and Leica scope. Three replicates were performed. Each worm was recorded in ImageJ. Data were analyzed in GraphPad Prism using unpaired two-tailed t tests with Welch’s correction.
Thrashing assays
Request a detailed protocolAnimals were synchronized by placing 10 gravid adult worms on NGM plates seeded with E. coli OP50 and allowing them to lay eggs for 2 hr at 20 °C. The gravid adult worms were then removed, and the eggs were allowed to hatch and develop at 20 °C until day 1 adulthood. Worms were placed in a drop of M9 solution, as previously described (Leiser et al., 2015). The individual body bends were counted at maximum rate for 30 s. Thrashing was assayed on day 2 and day 10 of adulthood. The animals that were not used for the day 2 assay were transferred to fresh fed FUdR plates four times until they were ready to be assayed. Three replicates were performed. Data were analyzed in GraphPad Prism using unpaired two-tailed t tests with Welch’s correction as well as one-way ANOVA.
Lifespan assays
Request a detailed protocolGravid adults were placed on NGM plates containing 1 mM β-D-isothiogalactopyranoside (IPTG), 25 μg/ml carbenicillin, and the corresponding RNA interference (RNAi) clone from the Vidal or Ahringer RNAi library. 200 µL of HT115 bacteria expressing double stranded (ds) RNA of either the control empty vector (EV) or the RNAi of interest at optical density (OD) of 3.0 and concentration of 3 X was added to each plate. After 3 hr, the adults were removed, and the eggs were allowed to develop at 20 °C until they reached late L4/young adult stage. From here, 70 worms were placed on each RNAi plate and transferred to fresh RNAi +FUDR plates on day 1, day 2, day 4, and day 6 of adulthood. A minimum of two plates per strain per condition were used per replicate experiment. Experimental animals were scored every 2–3 d and considered dead when they did not move in response to prodding under a dissection microscope. Worms that crawled off the plate were not considered, but ruptured worms were considered as previously described (Leiser et al., 2015). A similar method was used for non-RNAi lifespan experiments, except the plates did not contain IPTG and worms were fed E. coli OP50. 200 µL of E. coli OP50 at OD of 3.0 and concentration of 3 X was added to each plate. A similar method was also used for carbachol (Thermo Fisher, L06674.14) and EDTA (Thermo Fisher, AM9260G) supplementation lifespan experiments, except 50 µM carbachol or 50 µM EDTA was added to the NGM plates without IPTG, and worms were fed E. coli OP50. 200 µL of E. coli OP50 at OD of 3.0 and concentration of 3 X was added to each plate. Optimal concentrations of carbachol and EDTA (50 µM) were determined by assessing survivability of worms exposed to a range of concentrations. Log-rank test was used to derive p-value for lifespan assays using p<0.05 cut-off threshold compared to EV or wild-type controls. Cox regression was also used to assess interactions between genotype and condition for lifespan assays sing p<0.01 cut-off threshold compared to controls.
Dietary restriction (sDR) lifespan assay
Request a detailed protocolGravid adults were placed on NGM plates seeded with 200 µL E. coli OP50. After 3 hr, the adults were removed, and the eggs were allowed to develop at 20 °C until they reached late L4/young adult stage. From here, 70 worms were transferred to fed FUdR plates seeded with 200 µL of E. coli OP50 at OD of 3.0 and concentrated 3 X on days 1 and 2 of adulthood. On day 3 of adulthood, DR conditions were transferred to plates with 109 seeded lawns and transferred every other day four times while the corresponding controls were transferred equally to fed ad-lib plates. This form of DR is termed solid DR (sDR; Greer and Brunet, 2009). 80 µL of 10 mM palmitic acid (Sigma-Aldrich) dissolved in 100% EtOH was added to the outer rim of the plate to prevent fleeing. A minimum of two plates per strain per condition were used per replicate experiment. Experimental animals were scored every 2–3 d and considered dead when they did not move in response to prodding under a dissection microscope. Worms that crawled off the plate were not considered, but ruptured worms were considered as previously described (Leiser et al., 2015).
Transcriptomic analysis
Request a detailed protocolApproximately 600 day 1 adult worms per biological replicate were washed with M9 buffer three times, frozen in liquid nitrogen, and then stored at –80 °C. RNA was extracted by adding 500 mL of Trizol reagent to the frozen worm pellets, followed by three freeze-thaw cycles with liquid nitrogen and water bath at 42 °C. Then, 500 µL of ethanol was added to the samples, and RNA was isolated using the Direct-Zol Miniprep Plus Kit (Cat#R2072). Purified RNA was sent to Novogene (Novogene Corporation Inc) for sequencing on the Illumina HWI-ST1276 instrument. Messenger RNA was purified from total RNA by using poly-T oligo-attached magnetic beads. After fragmentation, first strand cDNA was synthesized using random hexamer primers, followed by second strand cDNA. The library was constructed, which entailed end repair, A-tailing, adapter ligation, size selection, amplification, and purification, and then was sequenced on an Illumina device using paired-end sequencing. Gene ontology analysis was done using the National Institutes of Health DAVID Bioinformatics tool. The dataset has been submitted to GEO NCBI (GEO accession #GSE288007).
Gene expression assays
Request a detailed protocolRNA was isolated from day 1 adult worms (approximately 600 worms per strain) following three rounds of freeze-thaw in liquid nitrogen using Invitrogen’s Trizol extraction method, similar to the method described above. 1 µg of isolated and purified RNA was reverse transcribed to cDNA using SuperScript II Reverse Transcriptase (18064071, Invitrogen,). Gene expression levels were measured using 600 ng of cDNA and SYBR Green PCR Mastermix (A25742, Applied Biosystems) with primers at 10 μM concentration. mRNA levels were normalized using Y45F10D.4 as a reference gene (Taki and Zhang, 2013). List of qPCR primers used are in Supplementary file 6.
fmo-4 induction on RNAi
Request a detailed protocolGravid fmo-4p::mCherry transcriptional reporter adult animals were placed on NGM plates containing 1 mM β-D-isothiogalactopyranoside (IPTG), 25 μg/ml carbenicillin, and the corresponding RNAi clone from the Vidal or Ahringer RNAi library. After 3 hr, the adults were removed, and the eggs were allowed to develop at 20 °C until they reached late L4/young adult stage. Then 40–50 worms per plate were transferred to similar plates that contain FUdR for overnight. The following day, ~20 worms per condition were picked off these plates, added to a 2% agarose pad on a glass slide, anesthetized in 0.5 M sodium azide (Sigma), and imaged at 6.3 x magnification with the LASx software and Leica scope using the mCherry fluorescence channel (Miller et al., 2022). Three replicates were performed. Fluorescent intensity in the mCherry channel was measured in ImageJ. Brightness of images within a dataset was increased to the same level. Data were analyzed in GraphPad Prism using unpaired two-tailed t tests with Welch’s correction.
fmo-4 induction on carbachol
Request a detailed protocolGravid fmo-4p::mCherry transcriptional reporter adult animals were placed on NGM plates seeded with E. coli OP50. After 3 hr, the adults were removed, and the eggs were allowed to develop at 20 °C until they reached late L4/young adult stage. Then 30 worms were transferred to NGM plates seeded with E. coli OP50 also containing either 300 µM carbachol (Thermo Fisher, L06674.14) or water. An optimal concentration of carbachol was determined by first assessing survivability of worms exposed to a range of concentrations and then by assessing fluorescence of the fmo-4p::mCherry reporter worms exposed to a range of concentrations. The worms were incubated for 24 hr at 20 °C. After 24 hr, ~20 worms per condition were then picked off these plates and added to unseeded NGM plates, anesthetized in 0.5 M sodium azide (Sigma), and imaged at 6.3 x magnification with the LASx software and Leica scope using the mCherry fluorescence channel (Miller et al., 2022). Three replicates were performed. Each worm was measured for fluorescence in ImageJ. Data were analyzed in GraphPad Prism using t tests.
fmo-4 induction assay on EDTA
Request a detailed protocolGravid fmo-4p::mCherry transcriptional reporter adult animals were placed on NGM plates seeded with E. coli OP50. After 3 hr, the adults were removed, and the eggs were allowed to develop at 20 °C until they reached late L4/young adult stage. Then 30 worms were transferred to NGM plates seeded with E. coli OP50 topically spotted with 150 µL of 0.5 M ethylenediaminetetraacetic acid (EDTA) (ThermoFisher, AM9260G) or 150 µL of water. An optimal concentration of EDTA was determined by first assessing survivability of worms exposed to a range of concentrations and then by assessing the fluorescence of the fmo-4p::mCherry reporter worms exposed to a range of concentrations. The worms were incubated for 24 hr at 20 °C. After 24 hr, ~20 worms per condition were then picked off these plates and added to unseeded NGM plates, anesthetized in 0.5 M sodium azide (Sigma), and imaged at 6.3 x magnification with the LASx software and Leica scope using the mCherry fluorescence channel (Miller et al., 2022). Three replicates were performed. Each worm was recorded in ImageJ. Data were analyzed in GraphPad Prism using t tests.
GCaMP7f induction assay on carbachol and EDTA
Request a detailed protocolGravid GCaMP7f (SWF702; Dag et al., 2023) adult worms were placed on NGM plates seeded with E. coli OP50. After 3 hr, the adults were removed, and the eggs were allowed to develop at 20 °C until they reached late L4/young adult stage. Then 30 worms were transferred to NGM plates seeded with E. coli OP50 topically spotted with 150 µL of 0.5 M EDTA(Thermo Fisher, AM9260G), 300 µM carbachol (Thermo Fisher, L06674.14), or water. The worms were incubated for 24 hr at 20 °C. After 24 hr, ~20 worms per condition were then picked off these plates and added to unseeded NGM plates, anesthetized in 0.5 M sodium azide (Sigma), and imaged at 6.3 x magnification with the LASx software and Leice scope using the GFP fluorescence channel. Three replicates were performed. Each worm was recorded in ImageJ, with focus on the head region to assess neuronal expression. Data were analyzed in GraphPad Prism using t tests. For time course assays, gravid GCaMP7f adult worms were placed on unseeded NGM plates, anesthetized in 0.5 M sodium azide (Sigma), and imaged at 6.3 x magnification with the LASx software and Leica scope using the GFP fluorescence channel. Then 2 µL of either 300 µM carbachol or 10 mM EDTA were added to the worms and they were imaged using the GFP fluorescence channel at 0.5 min, 5 min, 10 min, 15 min, and 20 min. Three replicates were performed. Each worm was recorded in ImageJ, with focus on the head region to assess neuronal expression. Data were analyzed in GraphPad Prism using t tests.
Statistical analyses
Request a detailed protocolLog-rank test was used to derive p-value for lifespan and stress resistance survival assays using p<0.05 cut-off threshold compared to EV or wild-type controls. Cox regression was also used to assess interactions between genotype and condition for lifespans and stress resistance survival assays using p<0.01 cut-off threshold compared to controls. Source data 3 provides the results of the Log-rank test and Cox regression analysis, which were run in RStudio.
Data availability
Replicate data for all figures and figure supplements can be found in the source data files. Additionally, the RNA-sequencing dataset has been submitted to GEO NCBI (GEO accession #GSE288007).
-
NCBI Gene Expression OmnibusID GSE288007. fmo-4 promotes longevity and stress resistance via ER to mitochondria calcium regulation in C. elegans.
References
-
Mortality and morbidity in laboratory-maintained rhesus monkeys and effects of long-term dietary restrictionThe Journals of Gerontology. Series A, Biological Sciences and Medical Sciences 58:212–219.https://doi.org/10.1093/gerona/58.3.b212
-
large-scale screening for targeted knockouts in the Caenorhabditis elegans genomeG3: Genes, Genomes, Genetics 2:1415–1425.https://doi.org/10.1534/g3.112.003830
-
Mitochondria and calcium: from cell signalling to cell deathThe Journal of Physiology 529 Pt 1:57–68.https://doi.org/10.1111/j.1469-7793.2000.00057.x
-
Calcium and ROS: a mutual interplayRedox Biology 6:260–271.https://doi.org/10.1016/j.redox.2015.08.010
-
Calcium signaling and endoplasmic reticulum stressInternational Review of Cell and Molecular Biology 363:1–20.https://doi.org/10.1016/bs.ircmb.2021.03.003
-
Flavin-containing monooxygenases are conserved regulators of stress resistance and metabolismFrontiers in Cell and Developmental Biology 9:630188.https://doi.org/10.3389/fcell.2021.630188
-
Dietary restriction and lifespan: lessons from invertebrate modelsAgeing Research Reviews 39:3–14.https://doi.org/10.1016/j.arr.2016.12.005
-
Pull-down assay to characterize caMethods in Molecular Biology 1621:151–159.https://doi.org/10.1007/978-1-4939-7063-6_15
-
Life-span extension from hypoxia in Caenorhabditis elegans requires both HIF-1 and DAF-16 and is antagonized by SKN-1The Journals of Gerontology. Series A, Biological Sciences and Medical Sciences 68:1135–1144.https://doi.org/10.1093/gerona/glt016
-
Does EDTA-infusion affect calcium homeostatis leading to increased resistance to challenge?Acta Veterinaria Scandinavica Supplementum 97:29–34.
-
Calreticulin, a calcium-binding molecular chaperone, is required for stress response and fertility in Caenorhabditis elegansMolecular Biology of the Cell 12:2835–2845.https://doi.org/10.1091/mbc.12.9.2835
-
Flavin-containing monooxygenases in aging and disease: emerging roles for ancient enzymesThe Journal of Biological Chemistry 292:11138–11146.https://doi.org/10.1074/jbc.R117.779678
-
Roles of the HIF-1 hypoxia-inducible factor during hypoxia response in Caenorhabditis elegansJournal of Biological Chemistry 280:20580–20588.https://doi.org/10.1074/jbc.M501894200
-
Activation of genes involved in xenobiotic metabolism is a shared signature of mouse models with extended lifespanAmerican Journal of Physiology. Endocrinology and Metabolism 303:E488–E495.https://doi.org/10.1152/ajpendo.00110.2012
Article and author information
Author details
Funding
National Institutes of Health (R01AG075061)
- Scott F Leiser
National Institutes of Health (T32AG000114)
- Angela M Tuckowski
Glenn Foundation for Medical Research
- Scott F Leiser
University of Michigan (Rackham Research Grant)
- Angela M Tuckowski
The funders had no role in study design, data collection and interpretation, or the decision to submit the work for publication.
Acknowledgements
This work was supported by grants from NIH. AMT was supported by NIH T32AG000114 and the University of Michigan Rackham Research Grant. SFL was supported by R01AG075061 and the Glenn Foundation for Medical Research.
Version history
- Sent for peer review:
- Preprint posted:
- Reviewed Preprint version 1:
- Reviewed Preprint version 2:
- Version of Record published:
Cite all versions
You can cite all versions using the DOI https://doi.org/10.7554/eLife.99971. This DOI represents all versions, and will always resolve to the latest one.
Copyright
© 2024, Tuckowski et al.
This article is distributed under the terms of the Creative Commons Attribution License, which permits unrestricted use and redistribution provided that the original author and source are credited.
Metrics
-
- 896
- views
-
- 84
- downloads
-
- 0
- citations
Views, downloads and citations are aggregated across all versions of this paper published by eLife.