Loss of Frataxin activates the iron/sphingolipid/PDK1/Mef2 pathway in mammals
Abstract
Friedreich’s ataxia (FRDA) is an autosomal recessive neurodegenerative disease caused by mutations in Frataxin (FXN). Loss of FXN causes impaired mitochondrial function and iron homeostasis. An elevated production of reactive oxygen species (ROS) was previously proposed to contribute to the pathogenesis of FRDA. We recently showed that loss of frataxin homolog (fh), a Drosophila homolog of FXN, causes a ROS independent neurodegeneration in flies (Chen et al., 2016). In fh mutants, iron accumulation in the nervous system enhances the synthesis of sphingolipids, which in turn activates 3-phosphoinositide dependent protein kinase-1 (Pdk1) and myocyte enhancer factor-2 (Mef2) to trigger neurodegeneration of adult photoreceptors. Here, we show that loss of Fxn in the nervous system in mice also activates an iron/sphingolipid/PDK1/Mef2 pathway, indicating that the mechanism is evolutionarily conserved. Furthermore, sphingolipid levels and PDK1 activity are also increased in hearts of FRDA patients, suggesting that a similar pathway is affected in FRDA.
https://doi.org/10.7554/eLife.20732.001Introduction
FRDA is the most prevalent form of recessive cerebellar ataxia characterized by neurodegeneration and cardiomyopathy. Patients with FRDA exhibit a progressive degeneration of the dorsal root ganglia, sensory peripheral nerves, and dentate nuclei of the cerebellum. Most individuals develop several neurological symptoms at a juvenile age, but individuals with adult or late onset are occasionally reported (Koeppen, 2011). Mutations in FXN were discovered as the primary cause of FRDA (Campuzano et al., 1996). Studies of the yeast frataxin homolog identified a role for FXN in iron-sulfur cluster biosynthesis and iron homeostasis (Babcock et al., 1997; Mühlenhoff et al., 2002; Rötig et al., 1997). Loss of FXN has been shown to cause mitochondrial dysfunction in several model organisms (Anderson et al., 2005; Puccio et al., 2001; Rötig et al., 1997). Yet, whether iron accumulates in the nervous system is still under debate, as it has been reported that iron levels are not altered in the nervous system of a conditional knockout mouse model and in FRDA patients (Puccio et al., 2001; Simon et al., 2004; Solbach et al., 2014). However, in FRDA patients, iron was shown to accumulate in the dentate nuclei or in glia cells of dorsal root ganglia (Boddaert et al., 2007; Koeppen et al., 2009). In sum, the iron deposition phenotype is still controversial in mouse models and FRDA patients, and the role of iron in the pathophysiology of FRDA has not yet been determined.
It has been proposed that ROS, generated by impaired mitochondrial electron transport chain complexes or iron deposition, mediate the pathogenesis in FRDA (Bayot et al., 2011; Santos et al., 2010). However, emerging evidence suggests that ROS are not elevated in several model organisms, and several clinical trials based on antioxidant therapy have shown no or limited benefit in FRDA patients (Babcock et al., 1997; Llorens et al., 2007; Macevilly and Muller, 1997; Santhera Pharmaceuticals, 2010; Seznec et al., 2005; Shidara and Hollenbeck, 2010). Hence, the pathogenesis of this disease is still poorly understood.
In a recent study, we described the first fly mutant allele of frataxin homolog (fh), the fly homolog of FXN (Chen et al., 2016). We showed that loss of fh in Drosophila leads to iron accumulation in the nervous system, which in turn induces sphingolipid synthesis and ectopically activates Pdk1 and Mef2. Mef2 activation triggers the aberrant transcription of downstream target genes that causes the degeneration of fly photoreceptors (Chen et al., 2016). However, whether this pathway is also affected in vertebrates upon loss of FXN is unknown. Here, we removed the endogenous mouse Fxn gene by using an adeno-associated virus (AAV) and CRISPR/Cas9 strategy (Swiech et al., 2014). We show that the iron/sphingolipid/PDK1/Mef2 pathway is activated in mice upon loss of Fxn. Furthermore, sphingolipids and PDK1 activity are also up-regulated in hearts of FRDA patients, suggesting that this pathway is also affected in FRDA. Hence, down-regulation of this pathway provides possible new targets to interfere with disease progression.
Results
To test if the iron/sphingolipid/PDK1/Mef2 pathway is activated in vertebrates in vivo upon loss of Fxn, we reduced Fxn levels in the mouse nervous system by using the AAV and CRISPR/Cas9 system (Swiech et al., 2014). We designed two different Fxn single guide RNAs (sgRNAs) and tested their efficiency. The Fxn-sgRNAs induce lesions in the Fxn locus and reduce the levels of FXN protein when compared to the LacZ-sgRNA control in Neuro-2a cells (Figure 1—figure supplement 1A and B). To deliver LacZ- or Fxn-sgRNA into the nervous system, we injected AAV that carried sgRNA into the ventricle of Rosa26-Cas9 knock-in newborn mouse brains (Platt et al., 2014), and the efficiency of AAV infection into the cortical neurons was examined. As shown in Figure 1—figure supplement 1C, the majority of cortical neurons are infected with AAV, and the mRNA levels of Fxn in Fxn-sgRNA mice are less than 40% of the LacZ-sgRNA control mice (Figure 1—figure supplement 1D).
At approximately four months of age the Fxn-sgRNA mice (mice with Fxn-sgRNA AAV injection) develop an abnormal appearance when compared to LacZ-sgRNA control mice. These include a smaller body size and a hunchback phenotype at P130 (Figure 1A). Interestingly, these features are similar to the neuronal conditional knockout mice that were previously reported (Puccio et al., 2001). In addition, the Fxn-sgRNA mice become much less mobile than their LacZ-sgRNA littermates (Videos 1 and 2). The Fxn-sgRNA mice also display an aberrant reflex when lifted by the tail as they fail to spread their hind limbs (Figure 1B). To evaluate the sensorimotor coordination, we performed a rotarod test at two different ages. At P50, we observe no difference between LacZ-sgRNA and Fxn-sgRNA mice. However, Fxn-sgRNA mice show a significant reduction of latency to fall from the rod at age of P130 (Figure 1C). Besides the deficit on the rotarod test, Fxn-sgRNA mice also show a severe impairment in the wire hang test at P130 (Figure 1D). In summary, a removal of Fxn in a substantial neuronal population in the newborn mouse brain causes a severe impairment of neuronal function.
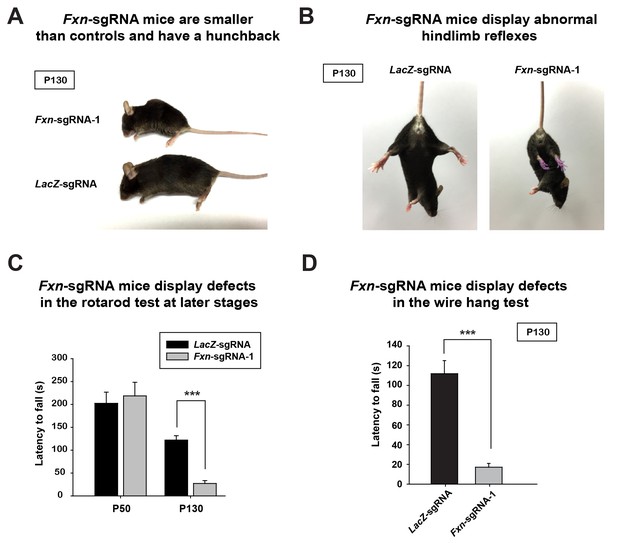
Phenotypic characterization of Fxn-sgRNA mice.
(A) Body size of LacZ- and Fxn-sgRNA mice at P130. Fxn-sgRNA mice exhibit a hunchback phenotype. (B) Hindlimb reflexes of LacZ- and Fxn-sgRNA mice at P130. (C) Rotarod test of LacZ- and Fxn-sgRNA mice at P50 (n = 5) and P130 (n = 6). (D) Wire hang test of LacZ- and Fxn-sgRNA mice at P130. n = 6. Data are presented as mean ± SEM. ***, p<0.001, Student’s t-test.
LacZ- and Fxn-sgRNA mice at P130.
https://doi.org/10.7554/eLife.20732.004Fxn-sgRNA mice at P130.
https://doi.org/10.7554/eLife.20732.005We further characterized the neuronal phenotype by examining brain sections at P130. We observed that the activating transcription factor 3 (ATF3), a marker that is typically associated with cellular stress response and neuronal damage (Tsujino et al., 2000), is up-regulated in cortical neurons of Fxn-sgRNA mice (Figure 2A). However, no ATF3 positive cells were observed in LacZ-sgRNA mice (Figure 2A). In addition, the axon initial segments, specialized membrane regions where Na+ channels cluster to initiate action potentials (Szu-Yu Ho and Rasband, 2011), are lost in cortical neurons of Fxn-sgRNA mice. As shown in Figure 2B, the scaffolding protein required for the formation and maintenance of the axon initial segment, ankyrin-G (AnkG), is severely reduced or lost in cortical neurons in Fxn-sgRNA mice, especially in ATF3 positive neurons (Figure 2B). These data suggest that loss of Fxn in cortical neurons leads to severe neuronal injury and damage.
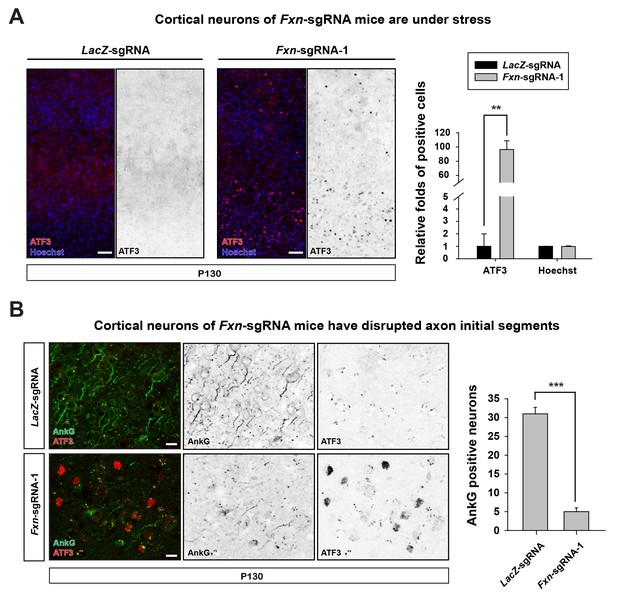
Loss of Fxn leads to neuronal insults.
(A) Immunostaining of cerebral cortex layer I (top) to VI (bottom) of LacZ- and Fxn-sgRNA mice at P130. ATF3 is labeled in red, and nuclei are marked by Hoechst in blue. n = 3. Quantification is on the right. Scale bar, 50 μm. (B) Immunostaining of cortical neurons of LacZ- and Fxn-sgRNA mice at P130. Axon initial segments are marked by anti-AnkG antibody (green), and ATF3 is marked in red. n = 3. Quantification is on the right. Scale bar, 10 μm. Data are presented as mean ± SEM. **, p<0.01, ***, p<0.001, Student’s t-test.
To assess if the iron/sphingolipid/PDK1/Mef2 pathway is activated in the brains of Fxn-sgRNA mice, we assessed if iron levels are increased upon loss of Fxn. To examine Fe3+ levels, we performed Perls’ staining and used 3,3'-diaminobenzidine (DAB) to enhance the signal. At P130 the DAB signal is strongly increased in brain sections of Fxn-sgRNA mice when compared to control mice (Figure 3A). To further characterize the iron distribution, we co-stain iron with neuronal and glia markers in the same brain sections. Since strong DAB signal interferes with the fluorescence, we chose the cortex region with relatively weak DAB deposits of Fxn-sgRNA mice. Interestingly, the majority of DAB signals are co-localized with a neuronal marker but not glial markers, suggesting that Fe3+ accumulates in neurons, although there are some weak diffuse signals distributed outside of the cell body (Figure 3 and Figure 3—figure supplement 1). These data show that Fe3+ accumulates in the nervous system upon loss of Fxn. The fact that we observed an obvious Fe3+ accumulation, whereas previous reports did not, is likely due to the use of DAB to enhance the Perls' Prussian blue staining, as we did not observe any signal with Perls' Prussian blue staining alone (Figure 3—figure supplement 2A).
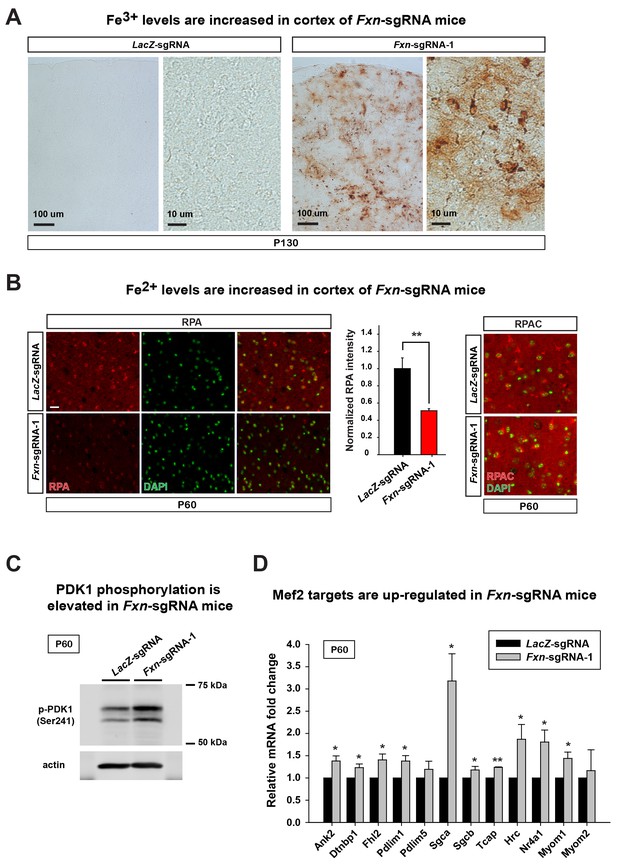
Loss of Fxn leads to an activation of the iron/sphingolipid/PDK1/Mef2 pathway.
(A) Perls’ blue staining with DAB enhancement of the cerebral cortex layer I (top) to VI (bottom) of LacZ- and Fxn-sgRNA mice at P130. n = 3. Scale bar, 100 and 10 μm. (B) RPA and RPAC staining of mouse cortical neurons of LacZ- and Fxn-sgRNA mice at P60. RPA and RPAC fluorescence is in red, and nuclei are labeled by DAPI in green. n = 3. Scale bar: 20 µm. (C) Immunoblot of PDK1 phosphorylation levels of brains of LacZ- and Fxn-sgRNA mice at P60. (D) mRNA levels of Mef2 downstream targets of LacZ- and Fxn-sgRNA mice at P60. n = 3. Data are presented as mean ± SEM. *, p<0.05. **, p<0.01, Student’s t-test.
We next assessed Fe2+ levels by using RPA (Petrat et al., 2002). RPA is a cell-permeable fluorescent dye that selectively accumulates in mitochondria, and its fluorescence is stoichiometrically quenched by Fe2+ (Petrat et al., 2002). Interestingly, we observed Fe2+ accumulation in the brain of Fxn-sgRNA mice at P60, a time point at which we did not observe behavioral defects in the Fxn-sgRNA mice. As shown in Figure 3B, the RPA fluorescence is quenched in cells of cortical layer IV and V of Fxn-sgRNA mice when compared to LacZ-sgRNA controls, indicating an accumulation of Fe2+ in the nervous system upon loss of Fxn. As a negative control, we used RPAC, a dye that is structurally very similar to RPA but is insensitive to Fe2+ mediated fluorescence quenching. RPAC is present at similar levels in both Fxn-sgRNA and LacZ-sgRNA brains (Figure 3B), suggesting that the decreased fluorescence of RPA in Fxn-sgRNA mice is not due to impaired dye uptake but due to Fe2+ quenching.
It was previously proposed that iron deposition triggers free radical production via Fenton’s reaction. We therefore measured oxidative stress by examining the levels of 4-hydroxynonenal (4-HNE), a major product of lipid peroxidation (Ayala et al., 2014). However, we did not observe an increase of 4-HNE in Fxn-sgRNA brain tissues (Figure 3—figure supplement 2B), consistent with previous observations in flies (Chen et al., 2016). We next determined if iron triggers the activation of the PDK1/Mef2 pathway in Fxn-sgRNA mice. Phosphorylation of S241 in the PDK1 activation loop is required for its activity (Casamayor et al., 1999). We observed an increase in phosphorylation levels of S241 in Fxn-sgRNA mice compared to the LacZ-sgRNA mice at P60, prior to the onset of symptoms (Figure 3C and Figure 3—figure supplement 2C). To assess if Mef2 is activated in Fxn-sgRNA mice, we determined the mRNA levels of several validated Mef2 downstream targets (Anderson et al., 2004; Ewen et al., 2011; Potthoff et al., 2007; Woronicz et al., 1995). As shown in Figure 3D, many, but not all, transcripts are up-regulated 1.5–3 fold in Fxn-sgRNA mice at P60. This is consistent with previous findings that only about half of Mef2 targets are up-regulated (to 2–3 fold) when Mef2A is overexpressed (Ewen et al., 2011). In summary, these results provide evidence that the PDK1/Mef2 pathway is activated in the vertebrate nervous system upon loss of Fxn prior to the onset of phenotypes that are observed about two months later.
Last, we tested if the same pathway is also activated in FRDA patients. As we were unable to obtain cerebella and dorsal root ganglia of patients, we used heart samples that were previously shown to accumulate Fe3+ (Bradley et al., 2000; Koeppen, 2011). We first investigated the sphingolipid levels in frozen heart tissues of five controls and six FRDA patients. As shown in Figure 4A, a substantial fraction of the sphingolipids are increased in FRDA patients when compared to controls, including short chain ceramides (C14-C18), different dihydroceramides, dihydrosphingosines, dihydrosphingosine-1-phosphate, and sphingosine. Finally, we tested if PDK1 activity is also increased in FRDA patients. The phosphorylation levels of S241 in PDK1 are elevated in FRDA patients when compared to controls (Figure 4B). Note that patient six showed no obvious increase in PDK1 phosphorylation when compared to the other patients. This patient had relatively mild symptoms and a later onset of disease (age 18) than other patients (ages 4 to 9). The age of death was also much later in patient 6 (age 67) compared to the other patients (age 25–37). Similarly, the increased levels of sphingolipids in patient six are also less pronounced than other patients. In sum, these data suggest that the iron/sphingolipid/PDK1/Mef2 pathway is activated in FRDA patients and that it may contribute to the pathogenesis of FRDA.
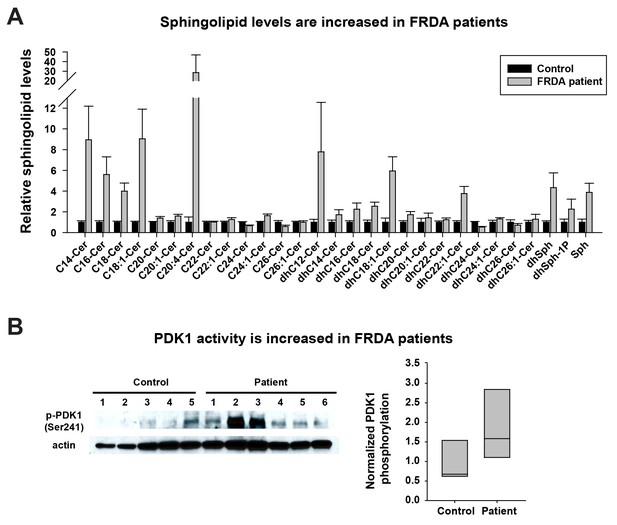
Sphingolipid levels and PDK1 activity are increased in heart tissues of FRDA patients.
(A) Sphingolipid profiling of heart tissues from controls (n = 5) and FRDA patients (n = 6). Data are presented as mean ± SEM. (B) Immunoblot of PDK1 phosphorylation levels of heart tissues from controls (n = 5) and FRDA patients (n = 6). Box plot of PDK1 phosphorylation is on the right.
Discussion
Here we show that removal of Fxn in the central nervous system using AAV and CRISPR/Cas9 causes behavioral and neurological phenotypes in mice. This new FXN mouse model exhibits several similar phenotypes to the previous neuronal conditional knockout mouse model, including smaller body size, hunchback phenotype, impaired locomotion, and shortened life span (Puccio et al., 2001). By using RPA and Perls'/DAB stainings, more sensitive iron staining assays than Perls’ Prussian blue staining alone, we found that Fe2+ and Fe3+ levels are increased in the cerebral cortex of Fxn-sgRNA mice, and the activities of PDK1 and Mef2 are up-regulated, suggesting that the iron/sphingolipid/PDK1/Mef2 pathway, previously identified in fly fh mutants, is also activated in Fxn-sgRNA mice. Importantly, sphingolipids and PDK1 activity are also up-regulated in the hearts of FRDA patients, indicating that a similar pathway is also activated and may contribute to the pathogenic mechanism of FRDA.
Iron toxicity has been long considered as a possible pathogenic contributor of FRDA. However, the iron accumulation phenotypes have never been reported in the nervous system in an inducible conditional knockout mouse model (Martelli and Puccio, 2014; Puccio et al., 2001; Simon et al., 2004). We provide the first direct evidence that both Fe2+ and Fe3+ accumulate in the brain upon loss of Fxn. In addition, we noticed that irons are not only deposited inside the neuronal cell body but also present in the extracellular space. For example, DAB staining showed a diffuse pattern in and surrounding the neuronal cell body (Figure 3A and Figure 3—figure supplement 1), and the RPA fluorescence is similarly reduced in the extracellular space (Figure 3B), suggesting that iron accumulation occurs both intracellularly and extracellularly.
It is worth noting that the iron/sphingolipid/PDK1/Mef2 pathway is conserved from yeast to fly (Chen et al., 2016; Lee et al., 2012), and that this pathway is also activated in Fxn-sgRNA mice and the hearts of FRDA patients. Interestingly, genetic variations at the 5’UTR of Mef2C that promote Mef2C transcription are associated with hypertrophic cardiomyopathy (Alonso-Montes et al., 2012). In addition, overexpression of Mef2A or Mef2C causes cell sarcomeric disorganization and elongation in cultured cardiomyocytes (Xu et al., 2006). These data suggest that an activation of iron/sphingolipid/PDK1/Mef2 pathway may play a role in the induction of cardiomyopathy in FRDA patients. Inhibition of this pathway may not only suppress neurodegeneration, but also prevent or delay cardiomyopathy, the major cause of lethality in FRDA. In sum, this pathway may provide us with therapeutic targets based on antisense oligonucleotide strategies (Ackermann et al., 2012), an option that should be explored.
Materials and methods
sgRNA design
Request a detailed protocolsgRNAs were designed using the CRISPR Design Tool (http://crispr.mit.edu). Control sgRNA sequence was designed to target lacZ gene from Escherichia coli (Swiech et al., 2014): TGCGAATACGCCCACGCGAT. Fxn-sgRNA sequences targeting the 5’ end of the Fxn gene locus were used: Fxn sgRNA-1, AGGGAACCGATCGTAACCTG and Fxn sgRNA-2, TTCGGAGGTCGCGCAGCCGT. sgRNAs DNA duplex were subcloned into the AAV-SpGuide vector (Addgene) (Swiech et al., 2014). To test sgRNA efficiency in genome editing, AAV-SpCas9 (Addgene) and AAV-sgRNA constructs were co-transfected into Neuro-2a cells. Cells were harvested 48 hr after transfection and the genomic DNA was extracted. A ~1 Kb fragment containing the Fxn sgRNA sequence was PCR amplified with the following primers, Fxn_F: 5’-CACCAGCGATCTTCAGAATCACC-3’ and Fxn-R: 5’-GGAGGCAGGAGGATCACAGG-3’. PCR products were then re-hybridized and cleaved by T7 endonuclease (NEB). Fragmented PCR products were then analyzed by 2% agarose gel. To test sgRNA efficiency in Fxn protein levels, AAV-SpCas9 (Addgene) and AAV-sgRNA constructs were co-transfected into Neuro-2a cells. As anti-FXN antibody cannot recognize the endogenous FXN proteins, we co-transfected Fxn-cDNA construct into Neuro-2a cells to allow FXN overexpression. Cells were then harvested and the FXN protein levels were analyzed by immunoblotting.
AAV production and purification
Request a detailed protocolAAV vectors were produced at the Gene Vector Core, Baylor College of Medicine. Dulbecco’s modified Eagle’s medium (DMEM) with high glucose (4.5 g/l) and antibiotic-antimycotic were purchased from GenDEPOT (Katy, TX), and fetal bovine serum (FBS) was purchased from Sigma (St. Louis, MO). HEK293T cells were grown in DMEM supplemented with 10% FBS and 1x antibiotic-antimycotic. DJ/8 Rep-Cap plasmid (Grimm et al., 2008) was purchased from Cell Biolabs (San Diego, CA). AAVs were packaged by three plasmids transfection (transfer vector, DJ/8, and helper plasmid) using iMFectin Poly DNA Transfection Reagent (GenDEPOT). In brief, 80–90% confluent HEK293T cells in a 15 cm dish were split into 6×15 cm dish one day before transfection. Next day, the media was removed and replaced with 9 ml of fresh DMEM/5% FBS. 24 µl of iMFectin reagent were diluted to 0.5 ml by DMEM and then added to 0.5 ml of DMEM containing 4 µg of helper plasmid, 2 µg of DJ/8 and 2 µg of transfer vector. The transfection mixture was vortexed for 10 s and incubated for 15 min at room temperature before overlay on HEK293T cells without removing media. After 4 hr, 10 ml of DMEM/5% FBS were added and cells were incubated in humidified CO2 incubator. AAV purification was performed by the method developed by Ayuso et al (Ayuso et al., 2010) with modifications. Three days after transfection, cells were collected by centrifugation at 2500 x g for 10 min. Cell culture supernatant was retained for subsequent precipitation. The cell pellet was re-suspended in 1 ml of 50 mM Tris pH8.0 containing 5 mM MgCl2 and 0.15 M NaCl per plate, lysed by adding 0.1 vol of 5% sodium deoxycholate at room temperature for 30 min and then incubated with 10 µg/ml of DNase I and RNase A for 1 hr at 37°C. Cell lysates were clarified by centrifugation at 5000 x g for 10 min at 4°C. Cell culture supernatant was incubated with 10 µg/ml of DNase I and RNase A for 1 hr at 37°C and then incubated at 4°C overnight after addition of a stock solution of 40% polyethylene glycol (PEG) 8000 containing 2.5 M NaCl to final concentration of 8% PEG. AAV in cell culture supernatant was collected by centrifugation at 2500 x g for 30 min. The pellets containing AAV were re-suspended in a minimal volume of HBS (50 mM HEPES, 0.15 M NaCl, 1% Sarcosyl, 20 mM EDTA, pH8.0). Cell associated and secreted AAVs were combined for the subsequent iodixanol density centrifugation (Zolotukhin et al., 1999). AAVs were dialyzed against phosphate buffered saline without Mg2+ and Ca2+ and the titer was determined by real time PCR using vector specific primers (Ljungberg et al., 2012).
AAV injection into the neonatal mouse brain
Request a detailed protocolRosa26-Cas9 knockin mice (Gt(ROSA)26Sortm1.1(CAG-cas9,-EGFP)Fez h/J, Stock No. 024858, the Jackson Laboratory, gift from Huda Zoghbi) were used for AAV injection. P1 neonates were cryo-anesthesized at 0°C for 3 min before injection. AAVDJ-8 carrying Fxn-sgRNA or control LacZ-sgRNA (2 × 1014 gc/ml) was injected into the ventricles using a pulled glass needle. After injection, pups were laid on a warm pad until recovery. The brains were dissected at P60 and P130 after virus injection. All mouse work and procedures were approved by the Animal Care and Use Committee at Baylor College of Medicine and were performed in accordance with the guidelines of the U.S. National Institutes of Health (animal protocol: an-4634).
Behavior test
Request a detailed protocolLacZ- and Fxn-sgRNA mice were subjected to accelerating rotarod and wire hang tests as previously described (Zhang et al., 2013).
Iron staining
Request a detailed protocolTo detect Fe2+ levels of AAV injected mouse brain, the brains were dissected and embedded into 2.5% low-melting agarose (Sigma-Aldrich). The 100 μm brain sections were acquired by a vibratome (1000 Classic; Warner Instruments) and transferred into 24 well plate with ice-cold artificial cerebrospinal fluid (ACSF) (125 mM NaCl, 2.5 mM KCl, 1 mM MgCl2 6H2O, 1.25 mM NaH2PO4, 2 mM CaCl2.2H2O, 25 mM NaHCO3, 25 mM Glucose, pH 7.4). The brain sections were then incubated with 10 µM RPA or RPAC (Squarix Biotechnology) in ACSF for 20 min at room temperature in the dark. The samples were washed subsequently three times by ACSF. To detect Fe3+ levels, brains were dissected and fixed in 4% paraformaldehyde for 1 hr on ice followed by immersion in 20% sucrose overnight at 4°C. Brains were then sectioned using a freezing stage Microtome (Microm KS 34, Thermo Scientific) and spread out on glass coverslips. Brain slices were then incubated with Perls’ solution (1% K4Fe(CN)6 and 1% HCl in 0.4% Triton-PBS) for 10 min. After three quick washes in 0.4% Triton-PBS, samples were incubated for 5 min with DAB solution (10 mg DAB with 0.07% H2O2 in 0.4% Triton-PBS) to enhance the signal. The brains were then quickly washed three times with 0.4% Triton-PBS and mounted. The images were obtained with an Axio-imager Z1 microscope (Carl Zeiss) fitted with an AxioCam digital camera (Carl Zeiss). The images were analyzed by ZEN 2012 (Carl Zeiss).
Immunofluorescence staining
Request a detailed protocolFor mouse brain staining, brains were dissected and fixed in 4% paraformaldehyde for 1 hr on ice followed by immersion in 20% sucrose overnight at 4°C. Brains were then sectioned using a freezing stage Microtome (Microm KS 34, Thermo Scientific) and spread out on glass coverslips for immunostaining. The antibodies were used at the following concentrations: mouse anti-AnkG (N106/36, N106/20) (UC Davis/NIH), 1:200; rabbit anti-ATF3 (Santa Cruz) (RRID:AB_2058590), 1:500; chicken anti-GFP (ab13970, abcam) (RRID:AB_300798), 1:1000; rabbit anti-Iba1 (019–19741, Wako), 1:250; chicken anti-GFAP (ab4674, abcam) (RRID:AB_304558), 1:500; NeuroTrace (Thermo Scientific), 1:200; Alexa 488-, Cy3-, or Cy5-conjugated secondary antibodies (Jackson ImmunoResearch), 1:250. Samples were then mounted in Vectashield (Vector Laboratories) before being analyzed under a confocal microscope. The images were obtained with an Axio-imager Z1 microscope (Carl Zeiss) fitted with an AxioCam digital camera (Carl Zeiss). The images were analyzed by ZEN 2012 (Carl Zeiss).
Immunoblot
Request a detailed protocolFor the Neuro-2a cells (RRID:CVCL_0470), cells were harvested and lysed in ice-cold RIPA buffer (50 mM Tris-HCl, 150 mM NaCl, 1% NP-40, 1% sodium deoxycholate, 0.1% SDS, 50 mM NaF, 1 mM Na3VO4, 10% Glycerol, protease inhibitor Cocktail (Roche)) 48 hr after transfection. The samples were then centrifuged at 16,100 ×g for 10 min to remove nuclei and debris. For the brain tissues, mouse brains were dissected, frozen on dry ice and homogenized as previously described (Ho et al., 2014). Briefly, brains were homogenized in ice-cold homogenization buffer (0.32 M sucrose, 5 mM sodium phosphate, pH 7.2, 1 mM NaF, 1 mM Na3VO4, and protease inhibitors). The homogenates were centrifuged at 700×g for 10 min at 4°C to remove nuclei and debris. The supernatant was then centrifuged at 27,200×g for 90 min at 4°C, and the pellet was resuspended in homogenization buffer (3 ml/g of brain). Protein concentrations were measured using a Bradford assay (Bio-Rad). The samples were resolved by SDS-PAGE, transferred to nitrocellulose membrane, and immunoblotted with the following primary antibodies: rabbit anti-PDK1 (Cel Signaling) (RRID:AB_2236832); rabbit anti-p-PDK1 (Cell Signaling) (RRID:AB_2161134); mouse anti-actin (ICN Biomedicals) (RRID:AB_2336056); rabbit anti-FXN (Santa Cruz) (RRID:AB_2110677); rabbit anti-4-HNE (abcam). Secondary antibodies were from Rockland Immunochemicals. Images were acquired and analyzed with Odyssey CLx Infrared Imaging System (LI-COR Biotechnology).
Mass spectrometry
Request a detailed protocolSphingolipid profiling of human heart tissues was performed by the Lipidomics Core at the Medical University of South Carolina as previously described (Chen et al., 2016).
Reverse transcription–quantitative polymerase chain reaction (PCR)
Request a detailed protocolTotal RNA from mouse brains were extracted by Trizol RNA Isolation Reagents (Thermo Fisher Scientific, Sugar Land, Texas) following the manufacturer’s instructions. The cDNA was synthesized by High-Capacity cDNA Reverse Transcription Kit (Applied Biosystems). Real-time PCR was performed using iQ SYBR Green Supermix (Bio-Rad) in a thermal cycler (iCycler; Bio-Rad Laboratories). The data were collected and analyzed using the optical module (iQ5; Bio-Rad Laboratories). The following primer pairs are used (5’to 3’): Gapdh forward (control primer), AGGTCGGTGTGAACGGATTTG; Gapdh reverse (control primer), TGTAGACCATGTAGTTGAGGTCA; Ank2 forward, AGATTACTGTGCAGCATAACAGG; Fxn forward, GCTGGAGGGAACCGATCGTA; Fxn reverse, TTCCTCAAATGCACCACGCAG; Ank2 reverse, TGGTTGTAAAGGAAACACACTCA; Dtnbp1 forward, TTAGCAGGTATGAGGATGCGT; Dtnbp1 reverse, GGTGCAGCAAATGGTTCTCTAC; Fhl2 forward, ATGACTGAACGCTTTGACTGC; Fhl2 reverse, CGATGGGTGTTCCACACTCC; Pdlim1 forward, TCGATGGGGAAGATACCAGCA; Pdlim1 reverse, TCTGTTCAGACCTGGATACTGTG; Pdlim5 forward, AGCAGCAAAATGGTAACCCTG; Pdlim5 reverse, CGTGAAGGGGTATCTTTTCCTTT; Sgca forward, GCAGCAGTAACTTGGATACCTC, Sgca reverse, AAAGGATGCACAAACACACGA; Sgcb forward, AGCACAACAGCAATTTCAAAGC, Sgcb reverse, AGGAGGACGATCACGCAGAT; Tcap forward, GATGCGCCTGGGTATCCTC; Tcap reverse, GATCGAGACAGGGTACGGC; Hrc forward, CAACCGATGTACCGAATGTGA; Hrc reverse, GGTAGCAGAATTGACAGTGCTG; Nr4a1 forward, TTGAGTTCGGCAAGCCTACC, Nr4a1 reverse, GTGTACCCGTCCATGAAGGTG; Myom1 forward, GCACGACCATGAGCCACTAC; Myom1 reverse, ACCCTTGAGAATGCCGGGA; Myom2 forward, AAAAGACACAAGCACTTTGACCA; Myom2 reverse, TGGGAGGATGACTGGGTGG.
Control and FRDA patient heart specimens
Request a detailed protocolFrozen heart specimens of FRDA patients were obtained from Dr. Arnulf Koeppen, Veterans Affairs (VA) Medical Center, Albany, NY, USA. Our receipt and analyses of these postmortem tissues was conducted with the approval of the Institutional Review Board at Baylor College of Medicine (protocol H-32191). Table 1 shows clinical and genetic data of six patients with FRDA. Five frozen normal control heart specimens were obtained from National Disease Research Interchange, Philadelphia, PA, USA.
Clinical and genetic data of 6 FRDA patients.
No. | Gender | Age of onset | Age of death | GAA1 | GAA2 |
---|---|---|---|---|---|
1 | M | 8 | 27 | 700 | 1070 |
2 | M | 4 | 37 | 674 | 674 |
3 | F | 5 | 25 | 800 | 1100 |
4 | F | 9 | 26 | 690 | 850 |
5 | M | 7 | 35 | 750 | 1100 |
6 | F | 18 | 67 | 621 | 766 |
-
The sizes for two GAA trinucleotide repeat expansions in the first intron of FXN in FRDA patients are shown with the smaller expansion designated GAA1 and the larger expansion designated GAA2.
References
-
New polymorphisms in human MEF2C gene as potential modifier of hypertrophic cardiomyopathyMolecular Biology Reports 39:8777–8785.https://doi.org/10.1007/s11033-012-1740-7
-
HRC is a direct transcriptional target of MEF2 during cardiac, skeletal, and arterial smooth muscle development in vivoMolecular and Cellular Biology 24:3757–3768.https://doi.org/10.1128/MCB.24.9.3757-3768.2004
-
RNAi-mediated suppression of the mitochondrial iron chaperone, frataxin, in DrosophilaHuman Molecular Genetics 14:3397–3405.https://doi.org/10.1093/hmg/ddi367
-
Lipid peroxidation: production, metabolism, and signaling mechanisms of Malondialdehyde and 4-hydroxy-2-nonenalOxidative Medicine and Cellular Longevity 2014:1–31.https://doi.org/10.1155/2014/360438
-
Clinical, biochemical and molecular genetic correlations in Friedreich's ataxiaHuman Molecular Genetics 9:275–282.https://doi.org/10.1093/hmg/9.2.275
-
The Mef2A transcription factor coordinately regulates a costamere gene program in cardiac muscleJournal of Biological Chemistry 286:29644–29653.https://doi.org/10.1074/jbc.M111.268094
-
A hierarchy of ankyrin-spectrin complexes clusters sodium channels at nodes of RanvierNature Neuroscience 17:1664–1672.https://doi.org/10.1038/nn.3859
-
The dorsal root ganglion in Friedreich's ataxiaActa Neuropathologica 118:763–776.https://doi.org/10.1007/s00401-009-0589-x
-
Friedreich's ataxia: pathology, pathogenesis, and molecular geneticsJournal of the Neurological Sciences 303:1–12.https://doi.org/10.1016/j.jns.2011.01.010
-
Sphingolipid signaling mediates iron toxicityCell Metabolism 16:90–96.https://doi.org/10.1016/j.cmet.2012.06.004
-
Oxidative stress does not appear to be involved in the aetiology of Friedreich's ataxiaRestorative Neurology and Neuroscience 11:131–137.https://doi.org/10.3233/RNN-1997-11303
-
The yeast frataxin homolog Yfh1p plays a specific role in the maturation of cellular Fe/S proteinsHuman Molecular Genetics 11:2025–2036.https://doi.org/10.1093/hmg/11.17.2025
-
Regulation of skeletal muscle sarcomere integrity and postnatal muscle function by Mef2cMolecular and Cellular Biology 27:8143–8151.https://doi.org/10.1128/MCB.01187-07
-
Friedreich ataxia: molecular mechanisms, redox considerations, and therapeutic opportunitiesAntioxidants & Redox Signaling 13:651–690.https://doi.org/10.1089/ars.2009.3015
-
Friedreich ataxia: the oxidative stress paradoxHuman Molecular Genetics 14:463–474.https://doi.org/10.1093/hmg/ddi042
-
In vivo interrogation of gene function in the mammalian brain using CRISPR-Cas9Nature Biotechnology 33:102–106.https://doi.org/10.1038/nbt.3055
-
Maintenance of neuronal polarityDevelopmental Neurobiology 71:474–482.https://doi.org/10.1002/dneu.20843
-
Activating transcription factor 3 (ATF3) induction by axotomy in sensory and motoneurons: A novel neuronal marker of nerve injuryMolecular and Cellular Neuroscience 15:170–182.https://doi.org/10.1006/mcne.1999.0814
-
Regulation of the Nur77 orphan steroid receptor in activation-induced apoptosisMolecular and Cellular Biology 15:6364–6376.https://doi.org/10.1128/MCB.15.11.6364
-
Myocyte enhancer factors 2A and 2C induce dilated cardiomyopathy in transgenic miceJournal of Biological Chemistry 281:9152–9162.https://doi.org/10.1074/jbc.M510217200
-
Membrane domain organization of myelinated axons requires βII spectrinJournal of Cell Biology 203:437–443.https://doi.org/10.1083/jcb.201308116
Article and author information
Author details
Funding
Friedreich's Ataxia Research Alliance
- Kuchuan Chen
- Guang Lin
- Hugo J Bellen
Robert A. and Renee E. Belfer Family Foundation
- Hugo J Bellen
Howard Hughes Medical Institute
- Hugo J Bellen
National Institutes of Health (1RC4GM096355)
- Hugo J Bellen
The Huffington Foundation
- Hugo J Bellen
Target ALS
- Hugo J Bellen
The funders had no role in study design, data collection and interpretation, or the decision to submit the work for publication.
Acknowledgements
We thank Karen Schulze for comments, Dr Huda Zoghbi for the Rosa26-Cas9 mice, and Dr Arnulf Koeppen for providing FRDA patient heart tissues. We thank National Disease Research Interchange for providing control heart tissues. We thank the Gene Vector Core at Baylor College of Medicine for the AAV production. We thank the Lipidomics Core at the Medical University of South Carolina for sphingolipid analysis. We thank the BCM Intellectual and Developmental Disabilities Research Center (IDDRC) confocal microscopy core, which is supported by the Eunice Kennedy Shriver National Institute of Child Health and Human Development (1U54 HD083092). We acknowledge support from the Friedreich's Ataxia Research Alliance, NIH (1RC4GM096355), the Robert A and Renee E Belfer Family Foundation, the Huffington Foundation, and Target ALS to HJB. HJB is an Investigator of the Howard Hughes Medical Institute.
Ethics
Human subjects: Postmortem cardiac tissue was provided by Dr. Arnulf Koeppen and collected at Veterans Affairs (VA) Medical Center, Albany, NY, USA. Our receipt and analyses of these postmortem tissues was conducted with the approval of the Institutional Review Board at Baylor College of Medicine (protocol H-32191).
Animal experimentation: All mouse work and procedures were approved by the Animal Care and Use Committee at Baylor College of Medicine and were performed in accordance with the guidelines of the U.S. National Institutes of Health (animal protocol: an-4634).
Copyright
© 2016, Chen et al.
This article is distributed under the terms of the Creative Commons Attribution License, which permits unrestricted use and redistribution provided that the original author and source are credited.
Metrics
-
- 1,982
- views
-
- 436
- downloads
-
- 51
- citations
Views, downloads and citations are aggregated across all versions of this paper published by eLife.
Citations by DOI
-
- 51
- citations for umbrella DOI https://doi.org/10.7554/eLife.20732