The neuropeptide F/nitric oxide pathway is essential for shaping locomotor plasticity underlying locust phase transition
Abstract
Behavioral plasticity is widespread in swarming animals, but little is known about its underlying neural and molecular mechanisms. Here, we report that a neuropeptide F (NPF)/nitric oxide (NO) pathway plays a critical role in the locomotor plasticity of swarming migratory locusts. The transcripts encoding two related neuropeptides, NPF1a and NPF2, show reduced levels during crowding, and the transcript levels of NPF1a and NPF2 receptors significantly increase during locust isolation. Both NPF1a and NPF2 have suppressive effects on phase-related locomotor activity. A key downstream mediator for both NPFs is nitric oxide synthase (NOS), which regulates phase-related locomotor activity by controlling NO synthesis in the locust brain. Mechanistically, NPF1a and NPF2 modify NOS activity by separately suppressing its phosphorylation and by lowering its transcript level, effects that are mediated by their respective receptors. Our results uncover a hierarchical neurochemical mechanism underlying behavioral plasticity in the swarming locust and provide insights into the NPF/NO axis.
https://doi.org/10.7554/eLife.22526.001eLife digest
Migratory locusts are widespread throughout the Eastern Hemisphere, especially in Asia, Australia and Africa. Although usually solitary insects, locusts can also form swarms made up of millions of individuals, which can devastate crops. Swarming can be studied on a smaller scale in the laboratory by forcing locusts to crowd together. This causes the locusts to enter a so-called gregarious state in which they are more active and sociable, which in turn promotes swarming. Isolating individual locusts has the opposite effect, causing the insects to enter a solitary state in which they are less active.
Chemicals in the locust brain called neuropeptides control phase transitions between solitary and gregarious behavior. Neuropeptides bind to specific proteins called receptors in the outer membranes of neurons and initiate unique signaling cascades inside cells. However, exactly how neuropeptides regulate the changes in locust behavior that affect swarming was not clear.
Hou et al. now reveal the role that two related neuropeptides, NPF1a and NPF2, play in this process. Crowding causes the levels of NPF1a and NPF2 in the locust brain to decrease, whereas isolating individual locusts causes the levels of two NPF receptors to increase. Both neuropeptides reduce levels of a molecule called nitric oxide in the brain. NPF1a does this by reducing the activity of the enzyme that produces nitric oxide, whereas NPF2 reduces the production of this enzyme. The reduction in nitric oxide in turn makes the locusts less active.
Similar NPF neuropeptides had previously been shown to affect activity levels in other invertebrates, such as roundworms and fruit flies. This, combined with the results now presented by Hou et al., suggests that the NPF/nitric oxide pathway may regulate activity in insects in general. Future work should investigate this possibility, as well as whether the NPF/nitric oxide pathway controls changes in other insect behaviors such as feeding and mating.
https://doi.org/10.7554/eLife.22526.002Introduction
Swarming occurs in a wide variety of animal taxa, including insects, fish, birds, and mammals. Individuals benefit from swarming in many aspects, including food searching, territory selection, and defense (Okubo, 1986; Weaver et al., 1989). Typically, to maintain the required fission–fusion dynamics, swarming animals exhibit striking behavioral plasticity of different types (Snell-Rood, 2006; Szyf, 2010). Biochemical changes in the levels of neuromodulators, such as monoamines, neuropeptides, and neurohormones, are able to induce behavioral variation thus mediate behavioral plasticity (Freudenberg et al., 2015; Godwin et al., 2015; Zupanc and Lamprecht, 2000). Nevertheless, the molecular basis by which neural factors orchestrate behavioral plasticity in swarming animals is poorly understood in detail.
Neuropeptides, a group of chemically diverse neural modulators, affect a broad range of physiological and behavioral activities (Lieberwirth and Wang, 2014; Nässel, 2002). Accumulating evidence shows that neuropeptides serve as conserved neuronal signals that modulate animal behaviors in social contexts (Lieberwirth and Wang, 2014; Nilsen et al., 2011). These peptides exert their actions by binding to specific membrane receptors, most of which are G-protein-coupled receptors (Quartara and Maggi, 1997). The binding initiates a second-message cascade unique for each receptor and results in a distinct molecular response (Hökfelt et al., 2003). It has been revealed that neuropeptides can induce plasticity in a series of behavioral processes, including sensory detection (Shankar et al., 2015), signal integration (Grammatopoulos, 2012), and behavioral responsiveness (Ruzza et al., 2015) by acting either individually or in concert with other neuromodulators (Dölen et al., 2011; Flores et al., 2015; Maroun and Wagner, 2016). Therefore, neuropeptides and their downstream components may act as vital parts of the regulatory network underlying behavioral plasticity in swarming animals.
The migratory locust, Locusta migratoria, exhibits two interconvertible phases, the solitarious phase (S-phase) and the gregarious phase (G-phase), the latter of which is characterized by swarming behavior (Ariel and Ayali, 2015). Locust behaviors in the two phases significantly differ, most notably in the interaction among individuals and in locomotor activity (Uvarov, 1977). S-phase locusts are sedentary and repel their conspecifics, whereas G-phase individuals are highly active and attract their conspecifics (Simpson et al., 1999). The behavioral transition between two phases is promoted by either isolating G-phase locusts (that is, solitarization) or, in the opposite direction, by forced crowding of S-phase locusts (that is, gregarization), the key step in seeding locust swarming (Pener and Simpson, 2009). Behavioral solitarization occurs faster than behavioral gregarization in the migratory locust. The attraction index and locomotor activity of locusts continuously decrease within 16 hr after isolation. By contrast, these behaviors do not increase until 32 hr after crowding, but are far below the level of gregarious controls even after crowding for 64 hr (Guo et al., 2011).
The locust brain undergoes strong neurochemical reconfiguration during behavioral phase transition; for instance, the contents of several neurotransmitters that mediate synaptic plasticity show significant change (Rogers et al., 2004; Ma et al., 2011, 2015). Recently, we have found that several neuropeptide genes are differentially expressed between the central nervous systems of G-phase and S-phase locusts (Hou et al., 2015), suggesting possible modulatory roles for these neuropeptides in the behavioral phase transition.
Here, we show that two related neuropeptides, NPF1a and NPF2, act as crucial neural modulators in the phase-related locomotor plasticity of the migratory locust. We uncover a potentially important connection between the atypical neurotransmitter NO and the two NPFs, a connection mediated by NOS. We therefore suggest that the actions of NPFs (or their homolog NPY) may be mediated, partly through NOS and NO, in other organisms.
Results
Two related neuropeptides, NPF1a and NPF2, affect phase-related locomotor activity
We have previously shown that 15 neuropeptide-encoding genes are differentially expressed in the brains of G-phase and S-phase locusts (Hou et al., 2015). Here, we extend our work to explore which of these neuropeptides are closely tied to the behavioral phase transition. qPCR analysis (Figure 1 and Figure 1—figure supplement 1) revealed that the mRNA levels of four neuropeptide encoding genes, namely, AKH/Corazonin related peptide (ACP), Insulin-like peptide (ILP), NPF1a, and NPF2, significantly changed in the phase transition, that is, during solitarization or gregarization or both. During gregarization, the mRNA levels of ACP and ILP steadily increased, whereas those of NPF1a and NPF2 rapidly decreased. During solitarization, the transcript levels of ILP and NPF1a significantly changed compared to those of ACP and NPF2.
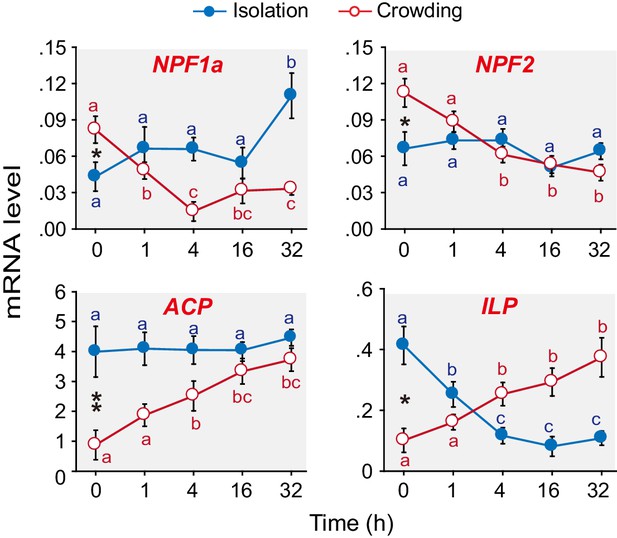
Levels of transcripts encoding the neuropeptides NPF1a, NPF2, ACP and ILP change during the G/S phase transition in the migratory locust.
qPCR was performed to determine the transcript levels of 15 neuropeptide-encoding genes in locust brains in the time course of the isolation of gregarious (G-phase) locusts or the crowding of solitarious (S-phase) locusts. Four neuropeptide genes displayed clear expression changes during isolation or crowding or both (in the case of NPF1a and ILP). Raw data measuring the mRNA levels of the four neuropeptide genes are shown in Figure 1—source data 1. For the transcript levels of the other 11 neuropeptide genes, see Figure 1—figure supplement 1. The data are presented as mean ± s.e.m. Significant differences at different times are denoted by letters (n = 4 samples per timepoint, 8 animals/sample, one-way ANOVA, p<0.05). *indicates a significant difference between typical G-phase (0 hr after isolation) and typical S-phase (0 hr after crowding) locust brains (Student’s t-test, *p<0.05, **p<0.01).
-
Figure 1—source data 1
mRNA levels of the four neuropeptide-encoding genes during isolation and crowding processes.
- https://doi.org/10.7554/eLife.22526.004
To assess whether these four neuropeptides are involved in the behavioral phase transition, we performed a behavioral screen in the G-phase locusts using transcript knockdown or peptide injection. The behavioral phase state was then assessed in an arena assay and measured by Pgreg, which is calculated using a binary logistic regression model that retains three variables: attraction index, total distance moved, and total duration of movement (Guo et al., 2011). Pgreg varies between 0 (in the fully S-phase behavioral state) and 1 (in the fully G-phase behavioral state). We performed RNAi-mediated transcript knockdown to reduce the levels of ACP and ILP, which show higher transcript levels in G-phase locust brains (Figure 1, lower). We found that knockdown of either ACP or ILP transcript did not significantly change the Pgreg values of G-phase locusts (Figure 2—figure supplement 1). On the other hand, we injected synthetic peptides to increase the concentrations of NPF1a and NPF2, which display lower transcript levels in G-phase locust brains (Figure 1, upper). G-phase locusts that were injected with NPF1a or NPF2 peptide behaved in a way that became considerably more solitarious, in a dose-dependent manner, when compared to control locusts (Figure 2A and Figure 2—figure supplement 2A). Co-injection of both NPF1a and NPF2 peptides into G-phase locusts enhanced the reduction of Pgreg compared to that seen following the injection of either NPF peptide alone (Figure 2A). Moreover, injection of NPF1a peptide provoked a faster inhibitory effect on the Pgreg values of locusts than that caused by NPF2 peptide injection (Figure 2B and Figure 2—figure supplement 2B). However, G-phase locusts that were injected with either dsNPF1a or dsNPF2 or with a mixture of these constructs did not show significant behavioral changes relative to control locusts (Figure 2—figure supplement 3, left).
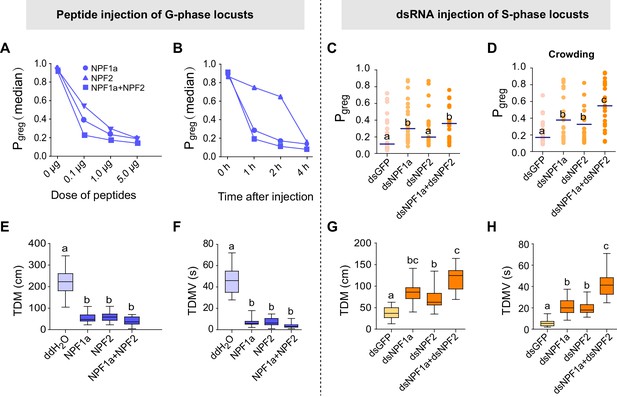
Perturbations of NPF1a or NPF2 peptide levels or of their transcript levels leads to changes in locomotor activity related to the G/S phase transition.
Locust behaviors are measured by the term Pgreg, which is a combined assessment of movement and inter-insect attraction (indicated as attraction index, see Figure 2—figure supplement 2). Pgreg = 0 represents a fully S-phase behavioral state; Pgreg = 1 represents a fully G-phase behavioral state. (A) and (B) Dose- and time-dependent changes in the median Pgreg of G-phase locusts after injection of NPF1a and NPF2 peptides, separately and together. For detailed Pgreg distributions and statistics, see Figure 2—figure supplement 2 (n ≥ 18 locusts, Mann–Whitney U test, p<0.05). (C) Pgreg in S-phase locusts 48 hr after transcript knockdown of NPF1a, or NPF2, or both (n ≥ 20 locusts, Mann–Whitney U test, p=0.020, 0.064 and 0.017, respectively). Lines indicate median Pgreg. Significant differences are denoted by letters. (D) Pgreg in crowded S-phase locusts after transcript knockdown of NPF1a, or NPF2, or both (n ≥ 20 locusts, Mann–Whitney U test, p=0.024, 0.039 and 0.037, respectively). Locusts were forced into a crowd 32 hr after dsRNA injection, and their behaviors were measured after 16 hr of crowding (that is 48 hr after dsRNA injection). (E) and (F) Total distance moved (TDM) and total duration of movement (TDMV) 4 hr after injection of NPF1a or NPF2 or both peptides in G-phase locusts (5 μg/individual). The data are presented as mean ± s.e.m. Significant differences are denoted by letters (n ≥ 18 locusts, one-way ANOVA, p<0.05). (G) and (H) Total distance moved (TDM) and total duration of movement (TDMV) 48 hr after transcript knockdown of NPF1a or NPF2 or both genes in S-phase locusts (n ≥ 20 locusts).
We validated the roles of two NPFs in the behavioral change in S-phase locusts by transcript knockdown of NPF1a and NPF2 individually or together. S-phase locusts that were injected with dsNPF1a displayed a significant behavioral change in the direction of G-phase, whereas injection of dsNPF2 did not significantly change the Pgreg values (Figure 2C and Figure 2—figure supplement 4). However, the S-phase locusts that were injected with either dsNPF1a or dsNPF2 were more gregarious than the controls in response to crowding stimuli, and these effects were strengthened by the dual-knockdown of the NPF1a and NPF2 transcripts (Figure 2D). Furthermore, peptide injection of NPF1a or NPF2 or their mixture in S-phase locusts did not affect their behavioral phase states (Figure 2—figure supplement 3, right).
Behavioral parameter analysis demonstrated that locust locomotor activity, including total duration of movement and total distance moved, were strongly suppressed by the treatments that increased the levels of NPF1a or NPF2 peptide in G-phase locusts, but enhanced by dsNPF1a or dsNPF2 injection in S-phase locusts (Figure 2E–H), while the attraction index was not significantly changed by these treatments (Figure 2—figure supplement 5). Thus, NPF1a and NPF2 play important roles in the locust behavioral phase transition by modulating locomotor activity.
Two NPF receptors, NPFR and NPYR, are essential for changes in locomotor activity related to the phase transition
Bioinformatically, we obtained two locust sequences with high similarity to the Drosophila NPFR gene (Supplementary file 1). They were named LomNPFR and LomNPYR, based on their phylogenetic relationship with homologs in other species (Figure 3—figure supplement 1B). Competitive binding experiments indicated that NPF1a peptide displayed much higher affinity to HEK 293 T cells expressing NPFR protein (IC50 = 24 nM) than did NPF2 peptide (IC50 = 355 nM) (Figure 3A and Figure 3—figure supplement 2), whereas NPF2 displayed much higher affinity to NPYR-expressing cells (IC50 = 64.5 nM) than did NPF1a (IC50 = 380 nM) (Figure 3B).
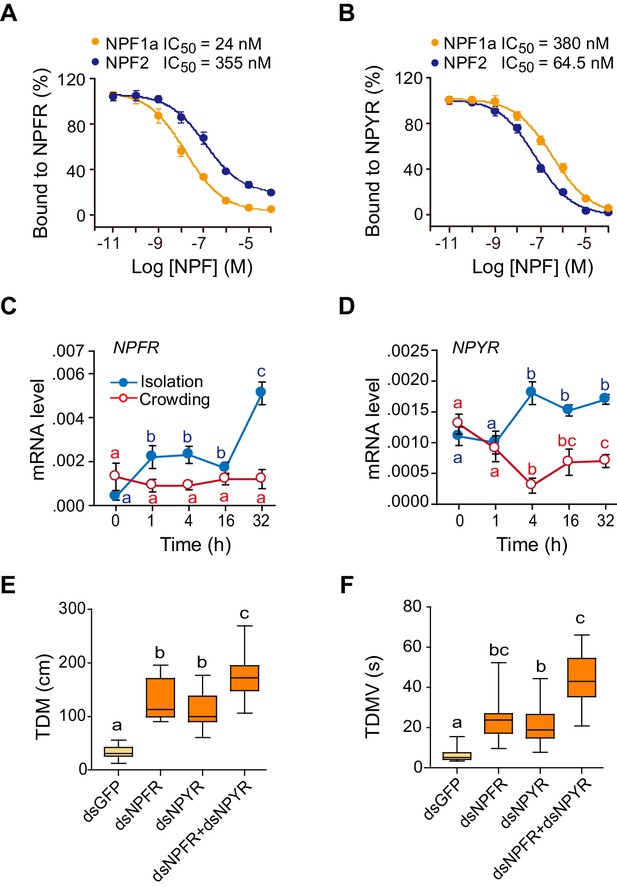
Receptors for NPF1a and NPF2 are involved in transmitting the effects of these neuropeptides on locomotor activity.
(A) Competitive inhibition of TAMRA-NPF1a binding to HEK 293 T cells transfected with pcDNA3.1-NPFR vector (n = 6). (B) Competitive inhibition of TAMRA-NPF2 binding to HEK 293 T cells transfected with pcDNA3.1-NPYR vector (n = 6). (C) and (D) Time course patterns of NPFR and NPYR transcript levels during the G/S locust phase transition (isolation, shown in blue; crowding, shown in red). The data are presented as mean ± s.e.m (n = 4 samples per timepoint, 8 locusts/sample, one-way ANOVA, p<0.05). Detailed expression levels of the two NPF receptors are shown in Figure 3—source data 1. (E) and (F) Total distance moved (TDM) and total duration of movement (TDMV) 48 hr after transcript knockdown of NPFR or NPYR or both genes in S-phase locusts. Significant differences are denoted by letters (n ≥ 19 locusts, one-way ANOVA, p<0.05).
-
Figure 3—source data 1
Transcript levels of NPFR and NPYR during the G/S locust phase transition.
- https://doi.org/10.7554/eLife.22526.013
The mRNA level of NPFR increased greatly within 1 hr after isolation of G-phase locusts, whereas it showed no change during locust crowding (Figure 3C). By contrast, the transcript level of NPYR responded to both isolation and crowding, with an obvious increase during isolation and a significant reduction during crowding (Figure 3D). Transcript knockdown of either NPFR or NPYR facilitated the transition from S-phase traits towards G-phase traits by influencing the locomotor activity of locusts (Figure 3E,F and Figure 3—figure supplement 3). Moreover, the dual-knockdown of NPFR and NPYR significantly strengthened the enhancement of both total distance moved and total duration of movement caused by knockdown of either transcript individually (Figure 3E,F). These results suggest that these two NPF receptors are essential for the regulation of phase-related locomotor activity.
NO signaling is a downstream component under the regulation of NPF1a and NPF2
To explore how NPF1a and NPF2 regulate locomotor plasticity during the G/S phase transition, we analyzed RNAseq-based transcriptomic differences in three comparisons: G-phase and S-phase locusts (comparison 1: C1); co-injection of NPF1a and NPF2 peptides in G-phase locusts with control injection (comparison 2: C2); co-injection of dsNPF1a and dsNPF2 in S-phase locusts with control injection (comparison 3: C3). We identified a total of 221, 317, and 313 differentially expressed genes in the three comparisons, respectively (Figure 4—figure supplement 1A), and 32% of these genes were annotated (Figure 4—figure supplement 1B). Numerous differentially expressed genes encoding catalytic and binding activities were clearly enriched in each treatment (Figure 4—figure supplement 1C).
A number of genes displayed altered transcription patterns (Figure 4A) that are consistent with locust behavioral change caused by the manipulation of NPF1a and NPF2 levels, as shown in Figure 2. The transcript levels of these genes were different between the typical G-phase and S-phase locusts (C1). Moreover, their transcript levels changed oppositely in the two treatments: co-injection of NPF1a and NPF2 peptides in G-phase locusts (C2) and dual-knockdown of NPF1a and NPF2 transcripts in S-phase locusts (C3). Among these genes, we found that several genes encode important signaling molecules. Using qPCR, the expression patterns of two genes, adenylate cyclase (AC2) and NOS, were confirmed in all three comparisons (Figure 4B and Figure 4—figure supplement 1D). The two genes showed high transcript levels in the brains of G-phase locusts. Moreover, their transcript levels were significantly lower after the co-injection of NPF1a and NPF2 peptides in G-phase locusts, and were increased by dual-knockdown of NPF1a and NPF2 transcripts in S-phase locusts (Figure 4B and Figure 4—figure supplement 1D).
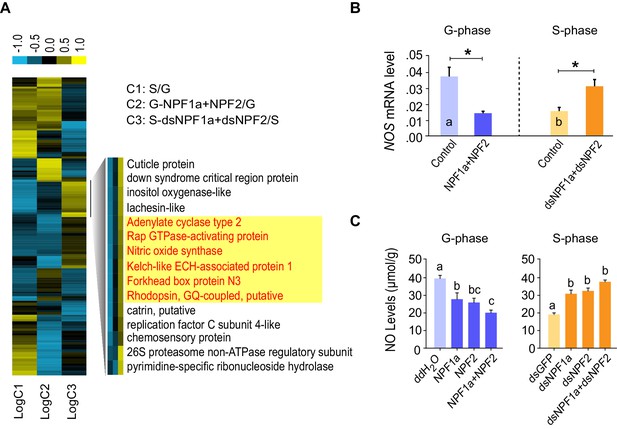
Cluster analysis of RNA-Seq data leads to the identification of nitric oxide synthase (NOS) as a downstream component of the NPF1a and NPF2 pathway.
(A) Cluster analysis of differentially expressed genes in the transcriptome. Several important genes (highlighted in yellow) involved in signaling in other organisms display expression patterns that correlate with behavioral change after the manipulation of NPF1a and NPF2 peptides or transcript levels. Logarithmic fold alteration of treatment versus control is shown in the heat map. Yellow and blue colors indicate up- and downregulation, respectively (n = 3 samples per treatment, 10 animals/sample). For detailed gene-expression data, please see Figure 4—source data 1. (B) Transcript levels of NOS in the brains after co-injection of NPF1a and NPF2 peptides in G-phase locusts or transcript knockdown of both NPF1a and NPF2 in S-phase locusts (n = 5 samples, 8 locusts/sample, Student’s t-test, *p<0.05, different letters labeled in columns indicate a significant difference). (C) NO levels after injection of NPF1a and NPF2 peptides, separately and together, in G-phase locusts, or after transcript knockdown of NPF1a and NPF2, separately and together, in S-phase locusts. The data are presented as mean ± s.e.m. Significant differences are denoted by letters (n = 4 samples, 16 locusts/sample, one-way ANOVA, p<0.05).
-
Figure 4—source data 1
The effects of NPF1a and NPF2 on the expression of annotated genes in the brains of fourth-instar locusts.
- https://doi.org/10.7554/eLife.22526.018
AC2 catalyzes cAMP production and might activate the PKA pathway, whereas NOS catalyzes NO production resulting in the activation of NO signaling (Mete and Connolly, 2003; Watts and Neve, 1997). We therefore examined whether cAMP and NO levels could be influenced by the manipulation of NPF1a and NPF2 levels. NO concentration in brains decreased dramatically within 4 hr after injection of NPF1a or NPF2 or of the peptide mixture into G-phase locusts, and significantly increased after knockdown of NPF1a or NPF2 or both NPF transcripts in S-phase locusts (Figure 4C). By contrast, there was no change in cAMP level 4 hr after manipulation of either NPF1a or NPF2 level (Figure 4—figure supplement 2). These data suggest that NO signaling may serve as a downstream pathway for both NPFs in the locust.
NO signaling acts as vital stimulator of locomotor activity in the G/S phase transition
The mRNA and protein levels of NOS were considerably higher in G-phase than in S-phase locust brains (Figure 5A,B), and significantly changed during the G/S phase transition (Figure 5C–E). Interestingly, NOS was present in both phosphorylated and non-phosphorylated forms (Figure 5—figure supplement 1A–C). Phosphorylated NOS was more abundant in the brains of G-phase locusts than in those of S-phase locusts (Figure 5B). Dephosphorylation of NOS by λ-phosphatase significantly reduced NOS activity and the NO level (Figure 5—figure supplement 1D,E). During the G/S phase transition, the level of NOS phosphorylation decreased or increased within 4 hr after solitarization or gregarization, respectively (Figure 5D,E). These changes occurred much faster than the alterations in NOS mRNA level, which did not change until 16 hr after solitarization or gregarization (Figure 5C). In addition, NO levels in the locust brains continuously decreased during solitarization, but sharply increased 32 hr after gregarization (Figure 5F). The changes in NO levels are tightly linked to the G/S behavioral phase transition.
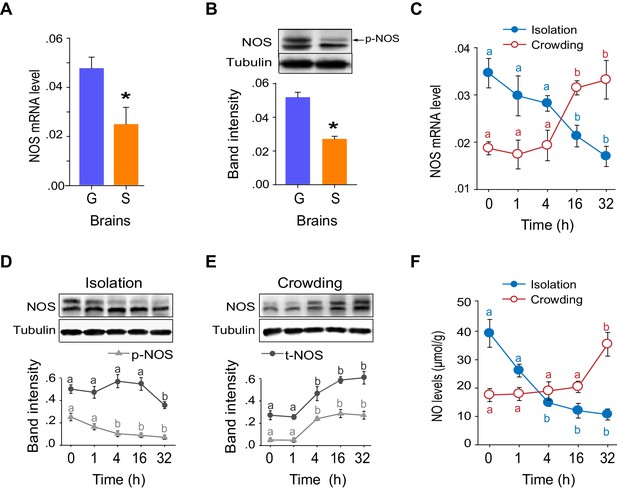
NOS transcript levels and phosphorylation states and NO levels differ in G-phase and S-phase locust brains.
(A) NOS mRNA levels in the brains of G-phase and S-phase locusts (n = 4 samples, 8 locusts/sample, Student’s t-test, *p<0.05). (B) NOS protein levels in the brains of G-phase and S-phase locusts. The upper band detected by anti-uNOS indicates phosphorylated NOS (p-NOS, see Figure 5—figure supplement 1) (n = 3 samples, 12 locusts/sample, Student’s t-test, *p<0.05). (C) Time course of NOS mRNA levels during the G/S phase transition (n = 4 samples/timepoint, 8 locusts/sample, one-way ANOVA, p<0.05, isolation shown in blue; crowding shown in red). (D) and (E) Time course of NOS protein levels during the G/S phase transition (n = 3 samples, 10–12 locusts/sample, phosphorylated NOS data are represented by triangles; total NOS data are represented by dots). The protein level is referenced to β-tubulin. (F) Time course of NO levels during the G/S phase transition. All data are presented as mean ± s.e.m. Significant differences are denoted by letters (n = 4 samples, 16 locusts/sample, one-way ANOVA, p<0.05). Raw data showing the changes in NOS mRNA level, NOS protein level and NO level are shown in Figure 5—source data 1.
-
Figure 5—source data 1
Time-course changes in NOS mRNA level, NOS protein level and NO level during the G/S phase transition.
- https://doi.org/10.7554/eLife.22526.022
We then conducted a series of molecular, pharmacological and behavioral experiments to investigate the function of NO signaling in the G/S locust phase transition. Knockdown of the NOS transcript or injection of the NOS inhibitor N-Nitro-L-arginine Methyl Ester (L-NAME) into G-phase locusts strongly suppressed the total duration of movement and total distance moved (Figure 6A–D), thus resulting in S-phase-like behavior (Figure 6—figure supplement 1A,C). By contrast, injection of S-phase locusts with the NO donor S-nitroso-N-acetyl-penicillamine (SNAP) enhanced the total duration of movement and total distance moved (Figure 6E,F), and pushed locust behavioral change from S-phase to G-phase state (Figure 6—figure supplement 1E). All manipulations (including NOS transcript knockdown and injections of the two chemicals) did not change the attraction index of tested locusts (Figure 6—figure supplement 1B,D,F). Furthermore, both transcript knockdown and L-NAME injection significantly reduced NOS activity and NO levels in G-phase locust brains, whereas SNAP injection increased NO levels in S-phase locust brains without affecting NOS activity (Figure 6—figure supplement 2).
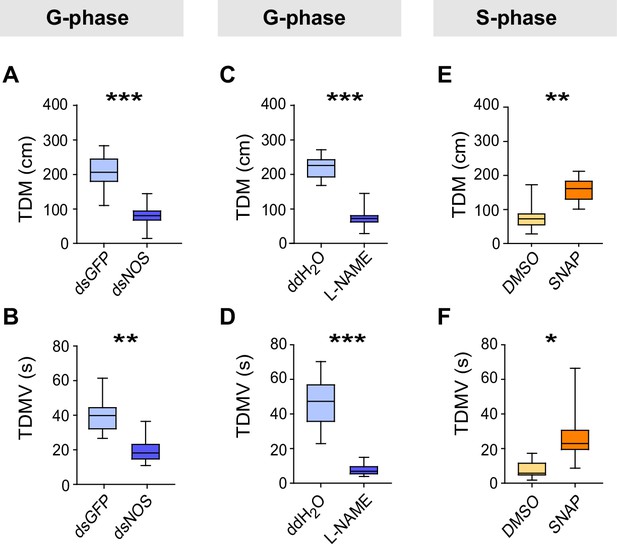
Perturbations of NO levels by transcript knockdown and drug injection dramatically change G-phase and S-phase locust behaviors.
(A) and (B) Total distance moved (TDM) and total duration of movement (TDMV) of G-phase locusts 48 hr after knockdown of the NOS transcript. All data are presented as mean ± s.e.m. (n ≥ 23 locusts, Student’s t-test, *p<0.05, **p<0.01, ***p<0.001). (C) and (D) Total distance moved (TDM) and total duration of movement (TDMV) of G-phase locusts 2 hr after injection of NOS inhibitor (L-NAME). (E) and (F) Total distance moved (TDM) and total duration of movement (TDMV) of S-phase locusts 2 hr after injection of NO donor (SNAP).
NPF1a and NPF2 sequentially suppress NO signaling at the phosphorylation and transcription levels
We have shown that NO levels were decreased by injection of either NPF1a or NPF2 and increased by knockdown of NPF1a or NPF2 transcripts (Figure 4C). Next we asked whether the two NPFs suppress the NO signaling pathway. The mRNA and protein levels of NOS significantly decreased 4 hr after injection of NPF2 peptide into G-phase locusts (Figure 7B,E). On the other hand, the mRNA and protein levels of NOS increased after knockdown of the NPF2 transcript in S-phase locusts (Figure 7C,F). By contrast, no change in NOS mRNA level was observed in any treatments involving NPF1a (Figure 7A,C). However, the level of phosphorylated NOS significantly decreased 1 hr after injection of NPF1a peptide into G-phase locusts (Figure 7D) and increased after knockdown of the NPF1a transcript in S-phase locusts (Figure 7F). Injection of NPF1a or NPF2 peptide into G-phase locusts significantly decreased NOS activity and NO levels in a time-dependent manner, with NPF1a exhibiting an earlier inhibitory effect on NO signaling than NPF2 (Figure 7G,H). Conversely, knockdown of NPF1a or NPF2 enhanced NOS activity in S-phase locusts (Figure 7I), which is consistent with the changing patterns of NO levels in the same treatments (Figure 4C). These data further verify the effects of NPF1a and NPF2 on NOS/NO signaling.
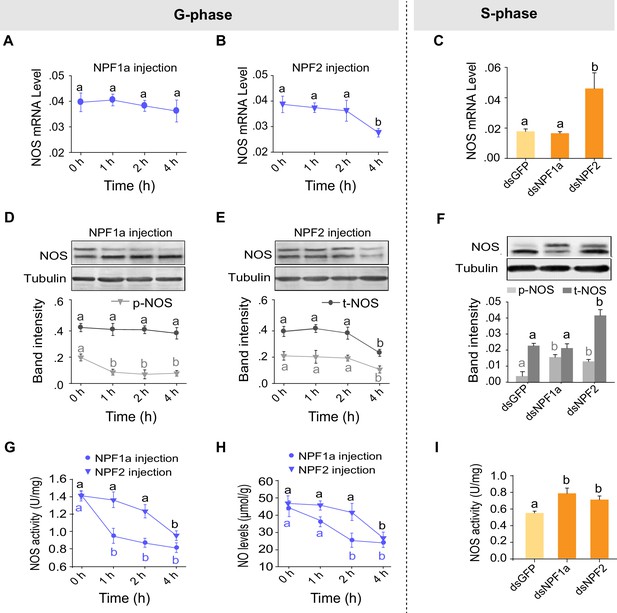
Manipulations of NPF1a and NPF2 levels alter NOS activity and phosphorylation states in the brains of G-phase and S-phase locusts.
(A) and (B) NOS mRNA levels after injection of NPF1a or NPF2 peptide into G-phase locusts. The data are presented as mean ± s.e.m. Significant differences are denoted by letters (n = 4 samples, 8 locusts/sample, one-way ANOVA, p<0.05). (C) NOS mRNA levels 48 hr after transcript knockdown of NPF1a or NPF2 in S-phase locusts (n = 4 samples, one-way ANOVA, p<0.05). (D) and (E) NOS protein levels after injection of NPF1a or NPF2 peptide into G-phase locusts (n = 3 samples, 10–12 locusts/sample, one-way ANOVA, p<0.05). (F) NOS protein levels 48 hr after transcript knockdown of NPF1a or NPF2 in S-phase locusts (n = 3 samples, one-way ANOVA, p<0.05). (G) NOS activity after injection of NPF1a or NPF2 peptide into G-phase locusts (n = 4 samples, 12–16 locusts/sample, one-way ANOVA, p<0.05). (H) NO levels after injection of NPF1a or NPF2 peptide into G-phase locusts (n = 4 samples, 12–16 locusts/sample, one-way ANOVA, p<0.05). (I) NOS activity 48 hr after transcript knockdown of NPF1a or NPF2 in S-phase locusts (n = 4 samples, one-way ANOVA, p<0.05).
NPF1a and NPF2 co-localize with NOS in the pars intercerebralis
To understand the neural basis for the interactions between two NPFs and NO signaling in the regulation of phase-related locomotion, we localized NOS and the two NPF peptides in the locust brain by double immunofluorescence staining. NOS was extensively expressed in the cell bodies of neurons in the pars intercerebralis and in the Kenyon cells anterior to the calyces of mushroom bodies in each brain hemisphere (Figure 8 and Figure 8—figure supplement 1). The distribution of NPF1a peptide was similar to that of NOS. NPF1a and NOS were co-localized in two regions, namely, the pars intercerebralis (Figure 8, upper) and the pars lateralis anterior to the calyces of mushroom bodies (Figure 8—figure supplement 1). However, NPF2 showed co-localization with NOS only in the cell body of neurons in the pars intercerebralis (Figure 8, lower). The co-localization of NPF1a and NPF2 with NOS in the pars intercerebralis of locust brain supports their linked action in phase-related behavioral changes.
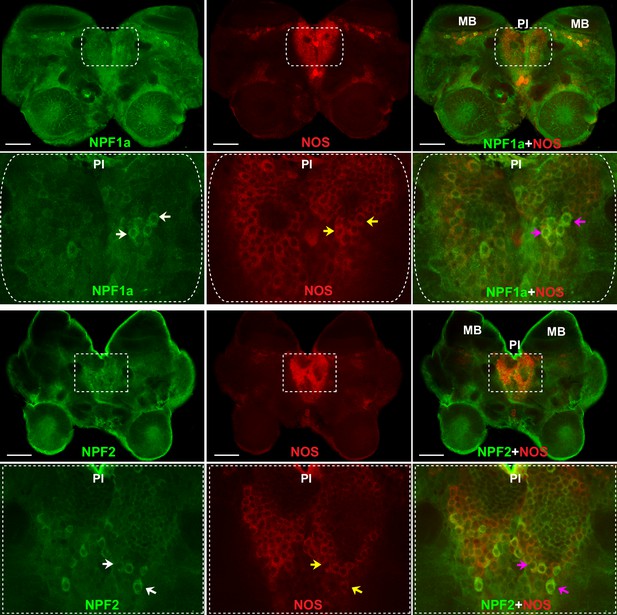
NOS and the two neuropeptides NPF1a and NPF2 co-localize in the pars intercerebralis of the locust brain.
NPF1a and NOS also co-localize in the neurons of pars lateralis anterior to the calyces of mushroom in each hemisphere in the locust brain (see Figure 8—figure supplement 1). White arrows indicateNPF1a or NPF2 staining, yellow arrows show NOS staining, pink arrows indicate merged signal of NOS and NPF1a or NOS and NPF2. Scale bars represent 100 μm.
NPFR and NPYR separately mediate distinct regulatory mechanisms involving NPF1a and NPF2 on NOS phosphorylation and transcription
On the basis of the different binding activities between each NPF and the two receptors, we speculated that the two NPF receptors, NPFR and NPYR, are responsible for the distinct effects on NOS induced by NPF1a and NPF2 (phosphorylated NOS levels were decreased by NPF1a injection whereas NOS transcript levels were reduced by NPF2 injection, as shown in Figure 7B,D). Knockdown of the NPFR transcript in S-phase locusts increased NOS phosphorylation level without affecting NOS transcript level (Figure 9A,B), similar to the effect caused by NPF1a knockdown (Figure 7A,D). By contrast, knockdown of the NPYR transcript led to increased NOS mRNA and NOS protein levels (Figure 9C,D). Furthermore, we investigated whether NPF1a and NPF2 could affect NOS phosphorylation or transcript level in G-phase locusts in which the transcripts of NPFR or NPYR had been knocked down. We found that knockdown of the NPFR transcript relieved the inhibition of NOS phosphorylation caused by NPF1a administration (Figure 9E,F), whereas knockdown of the NPYR transcript blocked NPF2-induced reduction in NOS mRNA and NOS protein levels in G-phase locusts (Figure 9G,H). These data indicate that NPFR and NPYR mediate distinct effects of NPF1a and NPF2 on NOS phosphorylation and transcription, respectively, in the locust brain.
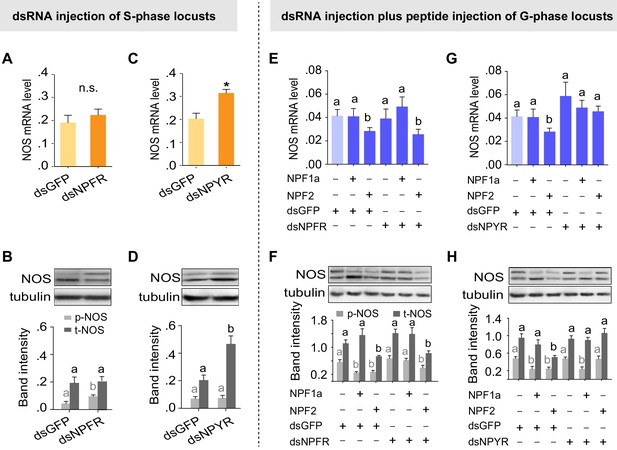
Two receptors mediate distinct effects of NPF1a and NPF2 neuropeptides on NOS phosphorylation and on NOS transcript levels, respectively.
(A) and (B) NOS mRNA levels (n = 5 samples, 6–8 locusts/sample) and NOS protein levels (n = 3 samples, 10–12 locusts/sample) 48 hr after transcript knockdown of NPFR in S-phase locusts. The data are presented as mean ± s.e.m. Significant differences are denoted by letters. n.s. means not significant (Student’s t-test, *p<0.05). (C) and (D) NOS mRNA levels (n = 5 samples) and NOS protein levels (n = 3 samples) 48 hr after transcript knockdown of NPYR in S-phase locusts. (E) and (F) NOS mRNA levels (n = 4 samples) and NOS protein levels (n = 3 samples) 4 hr after injection of NPF1a or NPF2 peptide in G-phase locusts pre-injected with dsNPFR. (G) and (H) NOS mRNA levels (n = 4 samples) and NOS protein levels (n = 3 samples) 4 hr after injection of NPF1a or NPF2 peptide in G-phase locusts pre-injected with dsNPYR. Detailed data describing NOS expression after injection of NPF1a or NPF2 peptide in G-phase locusts pre-injected with dsNPFR or dsNPYR are shown in Figure 9—source data 1.
-
Figure 9—source data 1
NOS mRNA levels and NOS protein levels after injection of NPF1a or NPF2 peptide into G-phase locusts pre-injected with dsNPFR or dsNPYR.
- https://doi.org/10.7554/eLife.22526.031
NO levels mediate the effects of NPF1a/NPFR and NPF2/NPYR on locomotor behavior related to phase transition
To determine whether the NPF-induced NO reduction directly regulates phase-related locomotor plasticity, we conducted rescue experiments by administrating SNAP to enhance NO concentration in G-phase locusts pre-treated with NPF1a or NPF2 peptide. SNAP administration resulted in robust recovery of the Pgreg values, total duration of movement, and total distance moved for G-phase locusts in which Pgreg values had been reduced by injection of either NPF1a or NPF2 peptide (Figure 10A).
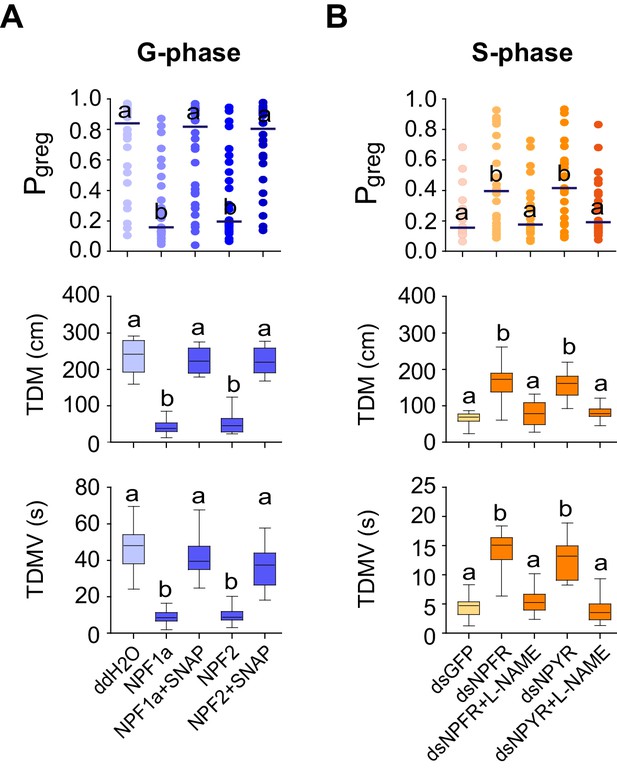
NPF1a, NPF2 and compounds that affect NO levels mediate effects on the locomotor behavior of G-phase and S-phase locusts.
(A) Behavioral test after administration of NO donor (SNAP) to G-phase locusts pre-injected with NPF1a or NPF2 peptide. Significant differences are denoted by letters. For Pgreg analysis, lines indicate median value (n ≥ 24 locusts; Mann–Whitney U test, p=0.0003 and 0.0001 for Pgreg NPF1a&SNAPvs. Pgreg NPF1a and Pgreg NPF2&SNAPvs. Pgreg NPF2, respectively). For TDM and TDMV analysis, the data are presented as mean ± s.e.m. (n ≥ 24 locusts, Student’s t-test, p<0.05). (B) Behavioral test after administration of NOS inhibitor (L-NAME) in S-phase locusts pre-injected with dsNPFR or dsNPYR (n ≥ 16 locusts, Mann–Whitney U test, p=0.022 and 0.042 for Pgreg dsNPFR&L-NAMEvs. Pgreg dsNPFR and Pgreg dsNPYR&L-NAMEvs. Pgreg dsNPYR, respectively). For TDM and TDMV analysis, the data are presented as mean ± s.e.m. (n ≥ 16 locusts, Student’s t-test, p<0.05).
We then tested the effects of the NOS inhibitor L-NAME in S-phase locusts that had been pre-treated with dsNPFR or dsNPYR. Transcript knockdown of either NPFR or NPYR enhanced phase-related locomotor activity and thus promoted the behavioral shift from S-phase state towards G-phase state (Figure 10B). However, L-NAME administration robustly abolished the increase in Pgreg values, total duration of movement, and total distance moved for test locusts induced by NPFR or NPYR transcript knockdown. These data suggest that NO signaling is an essential mediator for the effects of two NPFs and their receptors on phase-related locomotor plasticity in locusts.
Discussion
The current study reveals the inhibitory roles of two related neuropeptides, NPF1a and NPF2, and their receptors, NPFR and NPYR, in the locomotor activity related to locust phase transition. We provide evidence that NOS/NO signaling is a major mediator that transmits the effects of two NPF systems on phase-related locomotion. We establish a causation — the transcriptional changes in two NPF systems and the resulting converse alteration in NO levels in the locust brains contribute to variable locomotor activity during the G/S locust phase transition. Remarkably, NPF1a/NPFR and NPF2/NPYR suppress NOS activity and NO concentration at the levels of post-translational modification and transcription, respectively (see model in Figure 11).
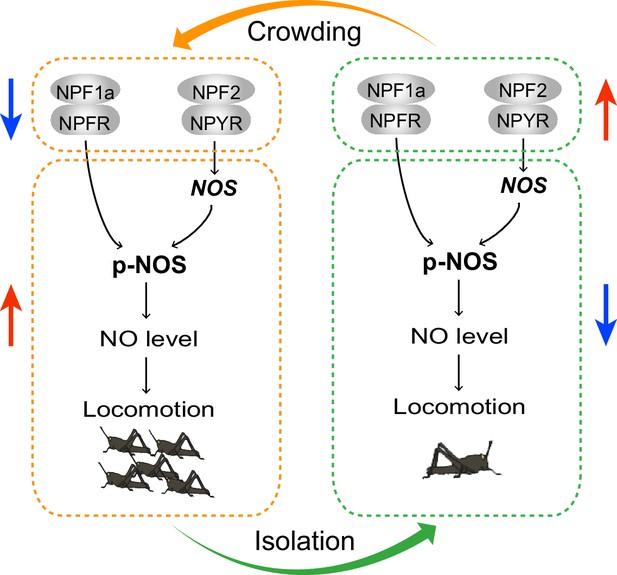
A model for the effects of neuropeptides NPF1a and NPF2 on locomotor activity related to phase transition of the migratory locust through NO signaling.
During the G/S phase transition, changes in the expression of two NPFs and their receptors act in concert to regulate the NO level, thus shaping phase-related locomotor plasticity. During crowding, the levels of two NPF systems decrease and promote NO level, resulting in higher locomotor activity. During isolation, the levels of two NPF systems increase and reduce NO level, thus leading to lower locomotor activity. Arrows indicate increased or decreased levels or activity.
The NPF/NO signaling pathway plays an essential role in phase-related locomotor plasticity in locusts
We show that manipulating the levels of two NPFs by peptide injection or transcript knockdown significantly affects phase-related behaviors among four neuropeptides that had differential levels during locust phase transition. These changes in locomotor behavior can be fully overcome by pharmacological administration of compounds that affect NO levels. Notably, NO signaling displays marked effects on locomotor activity; and the time-course changes in NO levels coincide well with locust behavioral transitions during both isolation and crowding (Guo et al., 2011), indicating NO is a decisive molecule for phase-related locomotion. The increased NO concentration may serve as a proximate cause of high locomotor activity in G-phase locusts, and decreased NO levels may lead to low locomotor activity in S-phase locusts. These data clearly suggest that the NPF/NO pathway plays a vital role in the modulation of phase-related locomotor plasticity. Our studies do not, however, preclude the regulatory roles of two other neuropeptides, ACP and ILP, in other phase-related characteristics or in long-term behavioral effects (Pener and Simpson, 2009).
Numerous studies have suggested that NPF signaling can influence a broad range of physiological and behavioral activities in insects, for instance feeding, reproduction, learning, circadian activity and stress responses (Nässel and Wegener, 2011; Lee et al., 2006; Krashes et al., 2009). Similar functional roles of the NPF (or NPFY) system in locomotion have been observed in two model invertebrate species, Caenorhabditis elegans (de Bono and Bargmann, 1998) and Drosophila melanogaster (Wu et al., 2003). These findings, in conjunction with ours, raise the possibility that the NPF system might serve as a common neural signaling pathway that shapes locomotor plasticity in invertebrates. In addition, recent studies have reported that NPF (referred to as NPF1a here) can mediate food intake, body weight and male-specific reproduction processes in the adults of another locust species, Schistocerca gregaria (Van Wielendaele et al., 2013a, 2013b), indicating that NPF plays multiple roles in locust biology.
Previous studies have suggested that phase differences in food choice are related to cryptic and aposematic strategies. Gregarious nymphs of S. gregaria are prone to consume more nutritionally imbalanced food and to accept food containing toxic chemicals more readily than do solitarious nymphs (Simpson et al., 2002). This is partly due to the relative gustatory insensitivity to low-quality food in gregarious locusts (Despland and Simpson, 2005). A link between NPF signaling and food choice has been suggested in Drosophila (Shen and Cai, 2001). Thus, a possible involvement of the NPF system could be speculated in phase-related behavioral choices of food quality in the locust nymphs. Further functional analysis is required to confirm this possibility and to elucidate the mechanisms through which NPF regulates multiple phase-related behavioral characteristics.
Our results indicate that NO signaling has a stimulatory role in locomotor activity in locusts. Several studies have suggested the significance of NO signaling in locomotor activity and behavioral plasticity in various animal species (Del Bel et al., 2005; Kyriakatos et al., 2009). For example, NO-initiating signaling has been shown to suppress aggression by promoting the tendency to flee in crickets (Stevenson PA, 2016) and to increase oviposition digging rhythm so as to control egg-laying movements in desert locusts (Newland and Yates, 2007). The cGMP/protein kinase G (PKG) pathway (the main downstream target of NO signaling) is involved in the control of foraging and locomotor behavior in Drosophila (Osborne et al., 1997), as well as in the regulation of labor division in honey bees (Ben-Shahar, 2005) and ants (Ingram et al., 2005). However, although there is a phase-dependent regulation of NO synthesis (Rogers et al., 2004) and a higher PKG activity in the anterior midline of brains of insects in the gregarious phase (Lucas et al., 2010), significant effects of PKG on behavioral phase state could not be observed in the desert locust S. gregaria (Ott et al., 2012). A reasonable explanation could be that the regulatory mechanisms of NO signaling in phase transition are species-specific, as is true of the roles played by several other neurotransmitters (Ma et al., 2011; Anstey et al., 2009). Another possibility is that NO regulates behavioral phase transition via a PKG-independent pathway in locusts (Newland and Yates, 2008).
NPF1a and NPF2, their receptors and NOS act in concert to regulate phase-related locomotion through NO signaling
We provide clear evidence that two NPFs acts as brakes that sequentially modify NO levels to control locomotor plasticity. The regulatory role of the NPF-NO pathway in locomotor behavior is further supported by the overlap immunostaining of two NPFs and NOS in the pars intercerebralis, which is linked to the regulation of locomotor rhythm in insects (Matsui et al., 2008). NO levels may reflect distinct physiological states and affect a wide variety of behaviors across species (Collmann et al., 2004; Davies, 2000; Del Bel et al. 2005; Cayre et al., 2005), yet how this molecule’s level responds to varied internal or external conditions remains unclear. To the best of our knowledge, this study is the first to show the link between NPF and NO signaling in shaping behavioral plasticity.
We show that the sequential inhibitory effects of NPF1a and NPF2 on NO levels are attributed to their regulation of NOS phosphorylation and NOS gene transcription, respectively, indicating that these two NPF members are not redundant in regulating phase-related locomotion. Phosphorylation is known to be an important form of post-translational modification (PTM) for a broad range of proteins, including receptors, transcriptional factors and vital enzymes (Kasuga et al., 1982; Matsuzaki et al., 2003; Bertorello et al., 1991). The phosphorylated proteins usually display changed spatial structures, subcellular locations and catalytic activity, and thus play key roles in rapid cellular signaling (Aguirre et al., 2002; Ho et al., 2011; Hurley et al., 1990). Studies in mammals have shown that NOS activity is tightly regulated by phosphorylation. For instance, the phosphorylation of Ser1412 stimulates NOS activity whereas Ser847 phosphorylation inhibits enzyme activity (Watts et al., 2013; Komeima et al., 2000).
NOS has also been suggested to be modified post-translationally in the locust embryo (Stern et al., 2010). Here, we show that NOS is modified by phosphorylation in the locust brains. Even if the total NOS protein level were not influenced by the activities of NPFs, simply reducing NOS phosphorylation leads to significantly decreased NOS activity and thus results in lower NO level, suggesting that NOS activity in the locust largely depends on its modification by phosphorylation. Therefore, NPF1a may lower the NO level by directly reducing NOS phosphorylation, whereas NPF2 may lower the NO level by reducing NOS substrate for phosphorylation. Our results show that NPF1a-regulated NOS phosphorylation cycles quickly, whereas NOS expression may respond more slowly to NPF2 regulation. Thus, the distinct modes of changing NO levels that are regulated by the two NPF systems not only explain the more rapid behavioral effect of NPF1a when compared to that of NPF2, but also emphasizes that downregulation of the NPF2 system is necessary in the G-phase to increase locomotion.
We show that two NPF peptides and their receptors may play synergistic roles in regulating the dynamic changes in NO levels during the two time-course processes of phase transition. The continuous reduction of NO levels during isolation is tightly controlled by the decreased NOS phosphorylation that results from the upregulation of NPFR and NPYR. By contrast, the reduction of two NPFs contributes mainly to the overall enhancement of NOS phosphorylation and NO levels during crowding. Although phosphorylated NOS shows greater activity than the unphosphorylated protein in promoting NO production, as shown previously, the enhancement of NO levels upon crowding seems to be delayed relative to that of NOS phosphorylation, implying that the stimulation of NO levels during crowding is a complex process that might involve additional regulators beyond the enzyme activity. NO level is dependent upon the balance between its production and degradation (Sansbury and Hill, 2014). NO generation not only depends on NOS expression and its post-translational modification but also relies on the availability of the corresponding substrate (e.g., L-Arginine) and cofactor (e.g., BH4, FAD or FMN) (Li and Poulos, 2005), whereas NO degradation may result directly from its reaction with reactive species (e.g., superoxide) (Channon, 2012). Given this, modulations of the availability of these factors may responsible for the sluggish increase of NO level during locust crowding.
Specific effects of different neuromodulators are essential for orchestrating phase-related behavioral traits
Locomotor activity is a major phase-related behavior that changes in response to population density (Wang and Kang, 2014). The high locomotor activity of G-phase locusts is potentially beneficial for rapid aggregation, synchronous movement, and avoidance of predators or conspecific cannibalism during locust swarming (Simpson et al., 1999). Therefore, the sequential modifications of NO levels resulting from NPF1a and NPF2 should allow dynamic locomotor adaptation to maintain locust swarming. Our previous studies have indicated that several other regulators, such as dopamine, serotonin and carnitines, are also involved in the modulation of phase-related locomotion in the migratory locust (Wu, et al., 2012; Ma et al., 2015). In addition, protein kinase A, a possible downstream factor of serotonin and dopamine, can regulate behavioral phase transition in the desert locust (Ott et al., 2012). It has been shown that the NPF/NPFR pathway has a dominant suppressive effect on PKA-sensitized sugar aversion in Drosophila (Xu et al., 2010). In our study, the expression level of AC2, one of the enzymes catalyzing cAMP production and activating PKA, is also affected by alteration of NPF levels in locusts. Studies in mammals have shown that both dopaminergic transmission and PKA could enhance NO levels thus leading to distinct biological actions (Wang and Lau, 2001; Yang et al., 2011). On the basis of these findings, we hypothesize that the two NPF systems may cooperate with the dopamine pathway to modulate locomotor activity during locust phase transition.
We show that the NPF/NO pathway is not involved in the modulation of another major phase-related behavioral characteristic, conspecific attraction induced by odors, in the migratory locust. This finding is superficially inconsistent with previous results on the roles of NPFs or NO in fine-tuning of food odor-induced behavior and olfactory learning in mice and the fruit fly (Rohwedder et al., 2015; Sung et al., 2014). One possible explanation is that pheromone-induced olfactory behaviors that are related to the locust phase change may involve regulatory mechanisms that are different from those involved in food-odor-induced olfactory responses in locusts. And, the locust phase transition is a continuous process involving changes of various characteristics including behaviors, metabolism, immunity and body color (Wang and Kang, 2014). In addition to its significance in behavioral modulation, NO signaling is also able to affect a variety of physiological and pathological processes (Bogdan, 2015; Calabrese et al., 2004; Sansbury and Hill, 2014). Thus, uncovering the long-term effects of the NPF/NO pathway on phase-related characteristics, such as disease resistance, energy metabolism and aging, will provide a more comprehensive understanding of the phase transitions that underlie locust swarming.
Materials and methods
Rearing of locusts
Request a detailed protocolG-phase locusts were maintained in large well-ventilated cages (40 cm × 40 cm × 40 cm) at a density of 500–1000 locusts per cage. S-phase locusts were reared individually in boxes (10 cm × 10 cm × 25 cm) supplied with charcoal-filtered compressed air. Both colonies were maintained at 30 ± 2°C and under 14:10 light/dark photocycle regime. The locusts were fed with fresh wheat seedling and bran (Guo et al., 2011).
Experimental samples for time-course analysis of gene expression during phase transition
Request a detailed protocolFor solitarization, fourth-instar G-phase nymphs were separately raised under solitarious conditions as described above. After 0, 1, 4, 16, or 32 hr of isolation, locust brains were collected and snap frozen. For gregarization, two fourth-instar S-phase nymphs were reared in small cage (10 cm × 10 cm × 10 cm) containing 20 G-phase locusts of the same developmental stage. After 0, 1, 4, 16, or 32 hr of crowding, locust brains were dissected and frozen in liquid nitrogen. All samples were stored at −80°C. Each sample contained a total of eight insects, including four male and four female insects. Four independent biological replicates were prepared for further experiments.
RNA preparation and qPCR
Request a detailed protocolTotal RNA was extracted using the RNeasy Mini Kit (Qiagen) according to the manufacturer’s protocol. cDNA was reverse-transcribed from 2 μg of total RNA using M-MLV reverse transcriptase (Promega). Gene-specific mRNA levels were assessed by qPCR using the SYBR Green kit on a LightCycler 480 instrument (Roche). RP49 was used as internal reference. The primers used are shown in Supplementary file 2.
Transcript knockdown via RNAi
Request a detailed protocolThe dsRNAs of target genes were prepared using the T7 RiboMAX Express RNAi system (Premega). dsRNA was microinjected into the brains of test insects (69 ng/locust for NPF1a, NPF2, ACP, ILP, and NOS, 1 μg/locust for NPFR and NPYR). dsGFP-RNA was used as control in all RNAi experiments. The behaviors of test locusts were measured 48 hr after injection as described below.
Peptide injection and drug treatments
Request a detailed protocolThe concentrations of peptides and drugs that were used were determined according to described methods (Newland and Yates, 2007; Van Wielendaele et al., 2013b). The commercially synthesized peptides (BGI, NPF1a peptide — YSQVARPRF-NH2; and NPF2 peptide — RPERPPMFTSPEELRNYLTQLSDFYASLGRPRF-NH2) were dissolved in ddH2O as stock solution (20 μg/μl). Working solutions of different concentrations (0.05, 0.5 and 2.5 μg/μl) were injected into the hemolymph in the heads of fourth-instar locusts using a microinjector (2 μl/locust). The arena behavioral assay was conducted 1 hr, 2 hr, and 4 hr following injection. L-NAME was dissolved with ddH2O to make a 1 mM stock solution. Working solution (100 μM) was microinjected into the heads of G-phase locusts (2 μl/locust). SNAP was dissolved with DMSO to prepare 100 mM stock solution. Working solution (200 μM) was microinjected into the heads of S-phase locusts (2 μl/locust). Locust behaviors were tested 2 hr after drug injection.
Behavioral arena assay
Request a detailed protocolThe behavioral assay was performed in a rectangular Perspex arena (40 cm × 30 cm× 10 cm) containing three chambers. The left chamber (7.5 cm × 30 cm × 10 cm) contained 30 fourth-instar G-phase locusts as a stimulus group, and the right chamber was empty (7.5 cm × 30 cm ×10 cm). Locusts behaviors were recorded for 300 s by an EthoVision video tracking system and analyzed according to the binary logistic regression model constructed in our previous work (Guo et al., 2011). Details are as follow: Pgreg = eη/ (1+eη); η = −2.11 + 0.005 × AI (attraction index) + 0.012 × total distance moved +0.015 × total duration of movement; AI = total duration in stimulus area − total duration in the opposite of stimulus area; this parameter represents the extent to which the tested animals are attracted by the stimulus group. TDMV (total duration of movement) and TDM (total distance moved) indicate the locomotor activity levels. Pgreg indicates the probability that a locust is considered gregarious. Pgreg = 1 represents a fully gregarious behavior, whereas Pgreg = 0 represents a fully solitarious behavior. In the behavioral assay, 16–35 locusts were tested for each treatment according to the sample size reported in previous studies (Ott et al., 2012; Ma et al., 2011). Locusts that did not move during behavioral testing were excluded.
Characterization of NPF receptors in locusts
Request a detailed protocolThe amino acid sequence of the Drosophila NPF receptor was used to search for NPF homologs in the locust genome database utilizing the tblastn algorithm. The phylogenetic relationship of NPFR and NPYR of insects and human was analyzed using MEGA software.
HEK 293 T cells (RRID: CVCL-0063) were purchased from the American Type Culture Collection (ATCC, CRL-3216, the identity has been authenticated using STR profiling) and cultured in low glucose DMEM (Life Technology) supplemented with 10% fetal bovine serum. Cells were routinely tested for mycoplasma every 6 months. For the competition binding assays, HEK 293 T cells transiently transfected with pcDNA3.1-NPFR or pcDNA3.1-NPYR (with a Flag-tag encoding sequence following target gene) were washed with 1 X PBS and added into 96-well plates (2 × 105 cells/well) coated with poly-L-lysine (0.1 mg/mL). Cells were then incubated with 25 μL TAMRA-NPF1a or TAMRA-NPF2 (10 nM) in the presence of increasing concentrations of unlabeled ligands in a final volume of 100 μL of binding buffer (PBS containing 0.1% bovine serum albumin). Nonspecific binding was determined by the addition of 25 μL unlabeled ligand. Mixtures were incubated at 30°C for 2 hr. Fluorescence intensity was measured using a fluorimeter (Molecular Devices) after washing twice with binding buffer. The HEK 293 T cells transfected with pcDNA3.1 were used as a control. The binding displacement curves were analyzed using the non-linear logistic regression method. Western blotting was carried out to validate the protein expressions of NPFR and NPYR in HEK 293 T cells using the mouse monoclonal antibody against Flag (CoWin, 1:5000).
RNA-seq and data processing
Request a detailed protocolThe brains of fourth-instar G-phase locusts were collected 4 hr after injection of the mixture of NPF1a and NPF2 peptides or ddH2O (a total of 5 μg). Similarly, the brains of fourth-instar S-phase locusts were collected 48 hr after injection of the mixture of dsNPF1a and dsNPF2 or dsGFP. Each sample contained 10 brains (5 males and 5 females). Three independent replicates were performed for each treatment. Total RNA was isolated as previously described, and RNA quality was confirmed by agarose gel. cDNA libraries were prepared according to Illumina’s protocols. Raw data were filtered, corrected, and mapped to locust genome sequence using Tophat software. The number of total reads was normalized by multiple normalization factors. Transcript levels were calculated using the reads per kb million mapped reads criteria. The difference sbetween the test and control groups were represented by P values. Differentially expressed genes with significance levels at p<0.05 in each comparison were enriched. In addition, unsupervised hierarchical clustering was performed using Clustal 3.0, which employs uncentered Pearson correlation and average linkage; results are presented by Java Treeview software. The RNA-seq data have been deposited in the Sequence Read Archive database of the National Center for Biotechnology Information (NCBI) (accession no. SRP092214).
Western blot analysis
Request a detailed protocolLocust brains (10–12 individuals/sample) were collected and homogenized in 1 X PBS buffer (0.1 M phosphate buffer, 0.15 M NaCl, pH 7.4) containing the phosphatase inhibitor PhosSTOP (Roche) and a proteinase inhibitor (CoWin). Total protein content was examined using the BicinChoninic Acid (BCA) Protein Assay Kit (Thermo). The extracts (100 μg) were reduced, denatured, and electrophoresed on 8% SDS-PAGE gel and then transferred to polyvinylidene difluoride membrane (Millipore). The membrane was then cut to two pieces and incubated separately with a specific antibody against the target protein of ~130 KD or a reference protein of ~55 KD overnight at 4°C (affinity-purified polyclonal rabbit antibody against uNOS, Sigma-Aldrich, 1:200; Rabbit polyclonal antibody against tubulin, CoWin, 1:5000). Goat anti-rabbit IgG was used as secondary antibody (CoWin, 1:10000). Protein bands were detected by chemiluminescence (ECL kit, CoWin). The band intensity of the Western blot was quantified using densitometry in Quantity One software.
For determination of NOS phosphorylation, 200 μg brain extracts were incubated with λ phosphatase and 1 X NEB buffer supplemented with 1 mM Mncl2 for 1 hr at 30°C (NEB). Control protein was treated under the same conditions without λ phosphatase. Western blot analysis was performed to confirm NOS phosphorylation in the locust brains.
Bioassays
Request a detailed protocolAn enzyme-linked immunosorbent assay (ELISA) kit (R and D Systems, Inc.) was used to detect the relative cAMP level in locust brains. For NO content determination, Total Nitric Oxide Assay Kit (Beyotime) was used. Because NO molecules are unstable, the total NO levels in all test groups were assessed by detecting the content of nitrate and nitrite. The NOS Kit (Nanjing Jiancheng Bioengineering Institute) was used to detect the total NOS activity in locust brains. Protein concentrations were measured using the BicinChoninic Acid (BCA) Protein Assay Kit (Thermo). All of these three measurements were performed according to the manufacturer’s instructions. Each measurement was from at least four biological replicates (12–16 locusts/replicate). Data were normalized to the protein concentration.
Immunohistochemistry
Request a detailed protocolWhole-mount double immunohistochemistry of locust brains was performed using affinity-purified polyclonal rabbit antibody against NPF1a or NPF2 (AbMAX, China, 1:100) and monoclonal mouse antibody against uNOS (Thermo, 1:200, RRID: AB_325476). Alexa Fluor-488 goat anti-rabbit IgG (Cat. A-11008, 1:500; Life Technologies) and Alexa Fluor-568 goat anti-mouse IgG (Cat. A-11019, 1:1000; Life Technologies) were used as secondary antibodies for NPFs and NOS staining, respectively. Fluorescence was detected using an LSM 710 confocal laser-scanning microscope (Zeiss). Photos for both positive staining and negative controls were imaged under the same conditions.
Determination of the molecular effects on NOS expression caused by NPFR and NPYR
Request a detailed protocolTo validate the involvement of NPFR and NPYR in the regulation of NOS expression and phosphorylation by two NPF peptides, the brains of fourth-instar S-phase locusts were microinjected with dsNPFR or dsNPYR, and collected 48 hr after injection. For gregaria, the brains of fourth-instar locusts were microinjected with dsNPFR, dsNPYR or dsGFP followed by NPF1a or NPF2 treatment 4 hr before sample collection. Total RNA and protein in each treatment were extracted according to the Invitrogen TRIzol RNA and protein extraction protocol. qPCR and Western blot analysis were performed to examine the influence of NPFR and NPYR on NOS expression and phosphorylation.
Behavioral rescue experiments in vivo
Request a detailed protocolFor G-phase locusts, synthesized NPF1a or NPF2 peptide (2.5 μg/μl) was microinjected into the fourth-instar insects. Two hours later, SNAP (200 μM, 2 μl/locust) was injected into the heads of the experimental insects. Control insects were treated with an equal amount of saline. The injected locusts were then raised under the gregarious condition and subjected to behavioral analysis 2 hr after injection of SNAP.
For S-phase locusts, dsNPFR or dsNPYR was microinjected into the brains of fourth-instar S-phase insects. Forty-six hours after injection, the NOS inhibitor L-NAME was microinjected into the locusts pre-treated with dsNPFR or dsNPYR. The insects treated with dsGFP were used as a control. Tested insects were thus raised under the solitarious condition and subjected to behavioral analysis 2 hr after injection of L-NAME.
Statistical analyses
Request a detailed protocolFor gene expression and enzyme activity analysis, we knew from the previous studies that a sample size of 6 animals per treatment was enough to detect significant differences among treatments (Ott et al., 2012; Yang et al., 2014). Therefore, 8–16 animals were examined in each experimental treatment. For behavioral measurement, we knew that 15 individuals per group was sufficient to detect reproducible differences between groups (Ma et al., 2011). All of the experiments were performed with at least three independent biological replicates.
Student’s t-test was used for two-group comparison. One-way ANOVO followed by Turkey’s post-hoc test was used for multi-group comparisons. Data that do not meet normal distribution were excluded in these statistics. Behavioral phase state analysis was performed using the Mann–Whitney U test because of its non-normal distribution feature. Differences were considered statistically significant at p<0.05. Data were analyzed using SPSS 20 software and presented as mean ± s.e.m. except for the Pgreg values, which are shown as median values.
Data availability
-
Locusta migratoria transcriptomePublicly available at the NCBI Sequence Read Archive (accession no: SRP092214).
References
-
Phosphorylation of Ser307 in insulin receptor substrate-1 blocks interactions with the insulin receptor and inhibits insulin actionJournal of Biological Chemistry 277:1531–1537.https://doi.org/10.1074/jbc.M101521200
-
Locust collective motion and its modelingPLoS Computational Biology 11:e1004522.https://doi.org/10.1371/journal.pcbi.1004522
-
The foraging gene, behavioral plasticity, and honeybee division of laborJournal of Comparative Physiology A 191:987–994.https://doi.org/10.1007/s00359-005-0025-1
-
Nitric oxide synthase in innate and adaptive immunity: an updateTrends in Immunology 36:161.https://doi.org/10.1016/j.it.2015.01.003
-
Nitric oxide and cellular stress response in brain aging and neurodegenerative disorders: the role of vitagenesIn Vivo 18:245–267.
-
A role for nitric oxide in sensory-induced neurogenesis in an adult insect brainEuropean Journal of Neuroscience 21:2893–2902.https://doi.org/10.1111/j.1460-9568.2005.04153.x
-
Tetrahydrobiopterin: a vascular redox target to improve endothelial functionCurrent Vascular Pharmacology 10:705–708.https://doi.org/10.2174/157016112803520819
-
Odorant-evoked nitric oxide signals in the antennal lobe of Manduca sextaJournal of Neuroscience 24:6070–6077.https://doi.org/10.1523/JNEUROSCI.0710-04.2004
-
Nitric oxide signalling in insectsInsect Biochemistry and Molecular Biology 30:1123–1138.https://doi.org/10.1016/S0965-1748(00)00118-1
-
Role of nitric oxide on motor behaviorCellular and Molecular Neurobiology 25:371–392.https://doi.org/10.1007/s10571-005-3065-8
-
Orexins and fear: implications for the treatment of anxiety disordersTrends in Neurosciences 38:550–559.https://doi.org/10.1016/j.tins.2015.06.005
-
Aggression in non-human vertebrates: genetic mechanisms and molecular pathwaysAmerican Journal of Medical Genetics Part B: Neuropsychiatric Genetics 171:603–640.https://doi.org/10.1002/ajmg.b.32358
-
Insights into mechanisms of corticotropin-releasing hormone receptor signal transductionBritish Journal of Pharmacology 166:85–97.https://doi.org/10.1111/j.1476-5381.2011.01631.x
-
Phosphorylation of FOXO3a on Ser-7 by p38 promotes its nuclear localization in response to doxorubicinThe Journal of Biological Chemistry 287:1545–1555.https://doi.org/10.1074/jbc.M111.284224
-
Molecular characterization and expression profiles of neuropeptide precursors in the migratory locustInsect Biochemistry and Molecular Biology 63:63–71.https://doi.org/10.1016/j.ibmb.2015.05.014
-
Neuropeptides: opportunities for drug discoveryThe Lancet Neurology 2:463–472.https://doi.org/10.1016/S1474-4422(03)00482-4
-
Task-specific expression of the foraging gene in harvester antsMolecular Ecology 14:813–818.https://doi.org/10.1111/j.1365-294X.2005.02450.x
-
Inhibition of neuronal nitric-oxide synthase by calcium/ calmodulin-dependent protein kinase IIalpha through Ser847 phosphorylation in NG108-15 neuronal cellsThe Journal of Biological Chemistry 275:28139–28143.https://doi.org/10.1074/jbc.M003198200
-
Nitric oxide potentiation of locomotor activity in the spinal cord of the lampreyJournal of Neuroscience 29:13283–13291.https://doi.org/10.1523/JNEUROSCI.3069-09.2009
-
The need for speed: neuroendocrine regulation of Socially-controlled sex changeIntegrative and Comparative Biology 55:307–322.https://doi.org/10.1093/icb/icv041
-
Structure-function studies on nitric oxide synthasesJournal of Inorganic Biochemistry 99:293–305.https://doi.org/10.1016/j.jinorgbio.2004.10.016
-
Social bonding: regulation by neuropeptidesFrontiers in Neuroscience 8:171.https://doi.org/10.3389/fnins.2014.00171
-
The locust foraging geneArchives of Insect Biochemistry and Physiology 74:52–66.https://doi.org/10.1002/arch.20363
-
Inhibitors of the NOS enzymes: a patent reviewIDrugs : The Investigational Drugs Journal 6:57–65.
-
Nitrergic modulation of an oviposition digging rhythm in locustsJournal of Experimental Biology 210:4448–4456.https://doi.org/10.1242/jeb.010009
-
Insulin-like peptide genes in honey bee fat body respond differently to manipulation of social behavioral physiologyJournal of Experimental Biology 214:1488–1497.https://doi.org/10.1242/jeb.050393
-
Dynamical aspects of animal grouping: swarms, schools, flocks, and herdsAdvances in Biophysics 22:1–94.https://doi.org/10.1016/0065-227X(86)90003-1
-
Locust phase polyphenism: an updateAdvances in Insect Physiology 36:1–272.https://doi.org/10.1016/s0065-2806(08)36001-9
-
Substantial changes in central nervous system neurotransmitters and neuromodulators accompany phase change in the locustJournal of Experimental Biology 207:3603–3617.https://doi.org/10.1242/jeb.01183
-
Neuropeptide F neurons modulate sugar reward during associative olfactory learning of Drosophila larvaeJournal of Comparative Neurology 523:2637–2664.https://doi.org/10.1002/cne.23873
-
Regulation of obesity and insulin resistance by nitric oxideFree Radical Biology and Medicine 73:383–399.https://doi.org/10.1016/j.freeradbiomed.2014.05.016
-
A behavioural analysis of phase change in the desert locustBiological Reviews of the Cambridge Philosophical Society 74:461–480.https://doi.org/10.1017/S000632319900540X
-
A comparison of nutritional regulation in Solitarious- and gregarious-phase nymphs of the desert locust Schistocerca gregariaThe Journal of Experimental Biology 205:121–129.
-
Phenotypic plasticity and the origin of novel traits: butterflies modify behavior and morphology in response to a novel environmentIntegrative and Comparative Biology 46:E133.
-
Development of nitrergic neurons in the nervous system of the locust embryoThe Journal of Comparative Neurology 518:spc1–1175.https://doi.org/10.1002/cne.22344
-
Behavior and epigenetics: long-term plasticity of the epigenome?Journal of Perinatal Medicine 38:S1.https://doi.org/10.1515/jpm.2010.191
-
Centre for Overseas Pest ResearchGrasshoppers and Locusts, Centre for Overseas Pest Research, London, UK.
-
Regulation of feeding by neuropeptide F in the desert Locust, Schistocerca gregariaInsect Biochemistry and Molecular Biology 43:102–114.https://doi.org/10.1016/j.ibmb.2012.10.002
-
Neuropeptide F regulates male reproductive processes in the desert Locust, schistocerca gregariaInsect Biochemistry and Molecular Biology 43:252–259.https://doi.org/10.1016/j.ibmb.2012.12.004
-
Molecular mechanisms of phase change in locustsAnnual Review of Entomology 59:225–244.https://doi.org/10.1146/annurev-ento-011613-162019
-
Activation of type II adenylate cyclase by D2 and D4 but not D3 dopamine receptorsMolecular Pharmacology 52:181–186.
-
Anti-hypertrophic and anti-oxidant effect of beta3-adrenergic stimulation in myocytes requires differential neuronal NOS phosphorylationJournal of Molecular and Cellular Cardiology 62:8–17.https://doi.org/10.1016/j.yjmcc.2013.04.025
-
Akt- and PKA-mediated endothelial nitric oxide synthase activation triggers early ischemic preconditioning in isolated rat heartsFASEB Journal : Official Publication of the Federation of American Societies for Experimental Biology 25:931–948.
Article and author information
Author details
Funding
National Natural Science Foundation of China (Youth fund (Grant NO. 31601875))
- Li Hou
National Natural Science Foundation of China (Grant NO. 31472047)
- Xianhui Wang
Chinese Academy of Sciences (Strategic Priority Research Program (Grant NO. XDB11010000))
- Xianhui Wang
- Le Kang
The funders had no role in study design, data collection and interpretation, or the decision to submit the work for publication.
Acknowledgements
We thank Gerald Reeck (Kansas State University and Institute of Zoology, Chinese Academy of Sciences) for constructive comments during the revision of this manuscript. We are grateful to Prof. Minmin Luo (National Institute of Biological Sciences, Beijing Institute), Prof. Chuan Zhou (Institute of Zoology, Chinese Academy of Sciences) for their insightful suggestions on this project. This work was supported by the Strategic Priority Research Program of CAS (Grant NO. XDB11010000) and the National Natural Science Foundation of China (Grant NO. 31601875 and 31472047).
Copyright
© 2017, Hou et al.
This article is distributed under the terms of the Creative Commons Attribution License, which permits unrestricted use and redistribution provided that the original author and source are credited.
Metrics
-
- 12,529
- views
-
- 544
- downloads
-
- 41
- citations
Views, downloads and citations are aggregated across all versions of this paper published by eLife.
Citations by DOI
-
- 41
- citations for umbrella DOI https://doi.org/10.7554/eLife.22526