Potassium dependent rescue of a myopathy with core-like structures in mouse
Abstract
Myopathies decrease muscle functionality. Mutations in ryanodine receptor 1 (RyR1) are often associated with myopathies with microscopic core-like structures in the muscle fiber. In this study, we identify a mouse RyR1 model in which heterozygous animals display clinical and pathological hallmarks of myopathy with core-like structures. The RyR1 mutation decreases sensitivity to activated calcium release and myoplasmic calcium levels, subsequently affecting mitochondrial calcium and ATP production. Mutant muscle shows a persistent potassium leak and disrupted expression of regulators of potassium homeostasis. Inhibition of KATP channels or increasing interstitial potassium by diet or FDA-approved drugs can reverse the muscle weakness, fatigue-like physiology and pathology. We identify regulators of potassium homeostasis as biomarkers of disease that may reveal therapeutic targets in human patients with myopathy of central core disease (CCD). Altogether, our results suggest that amelioration of potassium leaks through potassium homeostasis mechanisms may minimize muscle damage of myopathies due to certain RyR1 mutations.
https://doi.org/10.7554/eLife.02923.001eLife digest
Skeletal muscle covers our skeleton and allows us to move around. One disorder that leads to weakness in skeletal muscle—known as central core disease—can leave affected infants ‘floppy’ and delay the development of motor skills such as sitting, crawling, and walking. While no cure or treatment currently exists for the disease, researchers have found that most cases are connected to a mutation in the gene that makes a protein called ryanodine receptor type 1 (RyR1).
RyR1 belongs to a family of proteins that create channels for the controlled release of calcium ions from stores within cells. For muscle cells to contract, calcium ions must be released from these internal stores at the same time as potassium ions leave the cells. To relax the muscle cells, calcium ions are pumped back into the internal stores and potassium ions are taken back into the cell. Previous studies have established a role for RyR1 in the contraction of skeletal muscle, but the precise molecular details are not known.
Here, Hanson et al. studied mice that had symptoms of central core disease due to a mutation in the gene that makes RyR1. The muscle weakness in these mice was caused by defects that hindered the release of calcium ions from internal stores and leakage of potassium ions from the muscle cells.
The experiments reveal that a high-potassium diet alleviates the symptoms of disease in the mice by increasing the amount of potassium surrounding the muscle cells. Treatment with an existing drug called glibenclamide also reversed the disease symptoms by reducing the leakage of potassium ions from the cells.
Hanson et al. also found several genes involved in controlling potassium ion levels in cells that could act as indicators of the presence of the disease. These findings suggest that therapies targeting the control of potassium ion levels in muscle cells could minimize muscle damage in patients with central core disease.
https://doi.org/10.7554/eLife.02923.002Introduction
Myopathies due to mutations in RyR1 are inherited and currently incurable. Congenital myopathies are characterized by hypotonia and delay of motor development with weakness in skeletal muscles. In normal muscle, nuclei are located at the periphery of the myofibers and the mitochondria reside throughout the myofiber. Muscle biopsies of patients with myopathies and mutations in ryanodine receptor type 1 (RyR1) show centralized nuclei as well as disorganized areas in the center of the myofiber, called cores, that lack mitochondria and are devoid of metabolic activity, reflective of cellular damage. The most common RyR1-associated myopathy is central core disease (CCD) (Wu et al., 2006; Jungbluth, 2007). In addition, RyR1 mutations in muscle cause diseases such as multi-mini core disease (MMD), hypokalemic periodic paralysis, and malignant hyperthermia (MH), although the phenotype/genotype relationship is unclear (Fujii et al., 1991; Marchant et al., 2004; Treves et al., 2008; Wilmshurst et al., 2010).
Muscle movement is produced through excitation–contraction (E–C) coupling elicited by t-tubule conductance of the muscle action potential. Depolarization stimulates the dihydropyridine receptor (DHPR, CaV1.1) to mechanically open RyR1 channels in the sarcoplasmic reticulum (SR), which release intracellular calcium stores to flood the myofiber with calcium and initiate muscle contraction (Numa et al., 1990). During contractions, muscle cells influx Na+ and efflux K+, leading to depolarization of the membrane, a rise in extracellular K+, and loss of excitability. The myoplasmic loss of potassium is stabilized via increased potassium conductance from the opening of voltage-gated potassium channels (Lindinger et al., 2001; Nielsen et al., 2004) and the activation of sodium–potassium ATPase (Na+, K+-ATPase) transport (Clausen, 2013b).
During muscle contractions, the enzymes Na+/K+-ATPase, Ca2+-ATPase, and myosin ATPase consume most of the stored ATP. Myosin ATPase is considered to account for the majority of ATP consumption at 60–70% (Smith et al., 2005). Ca2+-ATPase is assumed to account for the majority of remaining ATP, while Na+–K+-ATPase can consume up to 10% of available ATP (Homsher, 1987; Clausen et al., 1991; Barclay et al., 2007). As ATP levels decrease during repeated muscle contractions and fatigue, ATP-sensitive KATP6.2 channels open causing potassium efflux, membrane depolarization, and inhibition of the action potential (Gong et al., 2003).
Thus, potassium homeostasis mechanisms preserve E–C coupled release of internal calcium stores, protect against muscle fatigue, and prevent excessive K+ loss (Clausen, 1986; Cifelli et al., 2008) and may protect the muscle during ATP loss (Gong et al., 2003).
In this study, we explore the function of RyR1 as it pertains to mechanisms of muscle weakness and potassium homeostasis. Through a forward genetic screen, we identified a mutation in Ryr1 that causes muscle weakness and myopathic pathological hallmarks in heterozygous mice. Furthermore, we examined the potential of this animal model for clinical and therapeutic studies. Mutant Ryr1 muscle showed decreased concentrations of Ca2+ and K+ in the myoplasm and an increased permeability for K+ in muscles at rest. In vitro, the potassium leak can be rectified through the application of higher concentrations of K+ or inhibition of KATP channels. In vivo, the muscle weakness and myopathic pathology can be reversed through increased interstitial potassium and pharmacological inhibition of KATP channels either by diet or FDA-approved drugs, suggesting defects in K+ homeostasis mechanisms as possible myopathological hallmarks due to mutations in RyR1. KATP6.2 channels and other regulators of potassium transport are misexpressed in mutant muscle and may partly underlie the disease phenotype in this myopathic animal model. Finally, we find that human patients with CCD show dysregulation of genes involved in the control of potassium homeostasis and suggest that these may serve as important disease biomarkers.
Results
Identification of RyR1 mutation that induces a myopathy with core-like structures in mice
Through a forward genetic screen in mice, we identified a mutation in which heterozygous mutant mice display muscle weakness. Sequencing identified an E4242G change in exon 93 of Ryr1 (Figure 1A) outside of the channel region of RyR1. This allele is named RyR1m1Nisw, but here we will refer to it as Ryr1AG. Gene identification was confirmed by a lack of complementation in a genetic cross between RyR1AG and RyR1tm1TAlle allele (see ‘Materials and methods’). RyR1 mutations in humans are associated with several congenital myopathies including CCD, multi-mini core disease, hypokalemic periodic paralysis, and malignant hyperthermia (MH) (Fujii et al., 1991; Marchant et al., 2004; Jungbluth, 2007; Treves et al., 2008; Wilmshurst et al., 2010). 1-month, 2-month, and 1-year old Ryr1AG/+ mice showed a significant decrease in grip strength and considerable deficits on a wire hanging task compared to Ryr1+/+ mice (Figure 1B,C). Malignant hyperthermia test was performed by placing Ryr1+/+ and Ryr1AG/+ mice in a 41°C humidified incubator for 30 min. There was no sign of muscle rigidity or spasticity or seizure activity for any of the animals. To test for sensitivity to halogenated anesthetics, anesthetic isoflurane was administered to Ryr1AG/+ mice at ∼5.5 × 10−5 ml/cm3 for a maximum of 30 min exposure. Ryr1AG/+ mice showed no MH response after exposure to isoflurane. The rectal temperature in Ryr1AG/+ mice after isoflurane exposure was similar to wild-type littermates [Ryr1AG/+ was 35.6 ± 0.9°C at 2 months (n = 5) and 35.9 ± 0.6°C at 6 months (n = 5) compared with Ryr1+/+ 35.4 ± 0.8°C and 35.8 ± 0.7°C at 2 and 6 months respectively (n = 5 for both)]. Thus, heterozygous mutant mice show muscle weakness, the clinical definition of a myopathy.
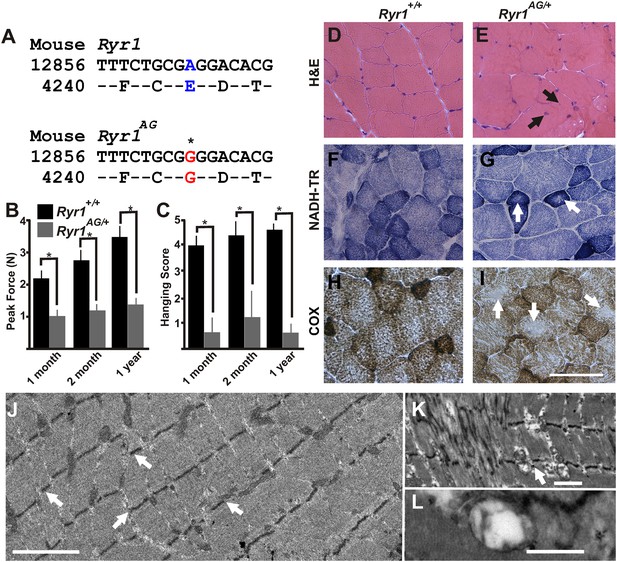
ENU-induced Ryr1AG mutation mimics clinical and pathological features of CCD in heterozygous mice.
(A) Missense mutation in exon 93 of Ryr1 changes A to G, resulting in substitution of glutamic acid with glycine (E4242G). (B) Average grip strength assayed using vertical digital push–pull strain gauge on Ryr1+/+ and Ryr1AG/+ mice at different ages raised on control 0.6% potassium diet. (C) In vivo hanging task determination of upper-body strength of Ryr1+/+ and Ryr1AG/+ mice at different ages (B, C, 10 trials/mouse, n = 5 per set). (D, E) H&E staining indicate central nuclei (arrows) in Ryr1AG/+ (E) but not Ryr1+/+ (D) vastus lateralis muscle from 1-year old mice raised on control 0.6% potassium diet. (F, G) NADH-TR staining indicates cores (white arrows) in 1-year old vastus lateralis of RyR1AG/+ but not Ryr1+/+. (H, I) Cytochrome oxidase (COX) staining denotes a decrease in mitochondrial function (white arrows) in vastus lateralis muscle of Ryr1AG/+ (I) compared to Ryr1+/+ (H). Scale bar = 50 μm. (J–L) Transmission electron microscopy of 2-month old Ryr1AG/+ soleus muscle. (J, K) Regions of Z line streaming and associated sarcoplasmic disruption and cores (white arrows) of myofibrils. (L) Enlarged T-tubules are present in type I fibers (scale bars: J = 1 μm; K = 2 μm; L = 0.2 μm ).
In typical muscle, nuclei are located at the periphery of the myofiber and mitochondria are present throughout. Muscle biopsies of CCD patients show internalized, centrally located nuclei, disorganized cores that lack mitochondria, and reduced metabolic activity (visualized with cytochrome oxidase (COX) and nicotinamide adenine dinucleotide hydride-tetrazolium reductase (NADH-TR) staining) (Wu et al., 2006). However, the number of cores present in muscle biopsies is not reflective of the degree of muscle weakness (Wu et al., 2006). Because proximal muscles of limbs are typically taken for muscle biopsies in patients, we examined the vastus lateralis and adductor magnus. Additionally, we examined the soleus muscle as it is commonly used in vitro studies of fatigue and RyR1-associated myopathy in mouse models. Analysis of these three muscles in 1-year old Ryr1AG/+ mice showed centrally located nuclei (13.2 ± 3.8 per 100 myofibers vs none detected in wild-type), core-like structures (Table 1), and decreased COX and NADH-TR staining, whereas no cores were observed in Ryr1+/+ muscle (Figure 1D–I). Focusing on type 1 fibers, core-like structures appear in 8.9 ± 1.8 per 100 NADH-TR stained type 1 fibers in 12-month old Ryr1AG/+ soleus muscle, which is comparable to the 12 cores per 100 NADH-TR stained fibers of 18-month old Ryr1IT (RyR1I4895T) soleus muscle, which has a congenital myopathy with cores (Zvaritch et al., 2009). In 2-month old Ryr1AG/+ muscle, we observed ultrastructural hallmarks of RyR1-associated myopathies (Boncompagni et al., 2009, 2010), including z-line streaming (white arrows in Figure 1J: Table 2), sarcomeric degeneration, irregularly shaped mitochondria, core-like structures (white arrow in Figure 1K), and swelling of the t-tubules (Figure 1L) as well as internalized nuclei (9.8 ± 4.9 per 100 myofibers vs none detected in wild-type). The onset and extent of the pathological and histological changes in multiple muscle types are similar to a mouse CCD model, RyR1I4895T (Boncompagni et al., 2009; Zvaritch et al., 2009; Boncompagni et al., 2010), altogether suggesting Ryr1AG mice as an additional model for myopathies with core-like structures.
Core-like structures in type 1 muscle fibers of 1-year old RyR1AG/+ mice as revealed by NADH-TR and COX labeling
NADH-TR negative cores | COX negative cores | |
---|---|---|
Vastus lateralis | 6.2 ± 2.4 | 7.8 ± 2.1 |
Adductor magnus muscle | 9.3 ± 2.9 | 9.7 ± 2.2 |
Soleus muscle | 8.9 ± 1.8 | 8.1 ± 1.4 |
-
Average values from 100 fibers with 10 slices per muscle and three muscles per group.
Number of Z line streaming sites within 100 μm2 grid in 2-month old wild-type and RyR1AG/+ soleus muscle
Genotype | 12-month old | 2-month old | |||
---|---|---|---|---|---|
0.6% Diet | 5.2% Diet | Enalapril | Glibenclamide | ||
Ryr1+/+ | 0.48 ± 0.21 | 0.04 ± 0.01 | 0.03 ± 0.01 | 0.05 ± 0.03 | 0.09 ± 0.08 |
Ryr1AG/+ | 10.67 ± 0.82** | 3.75 ± 0.05** | 0.34 ± 0.16* | 1.13 ± 0.14** | 1.12 ± 0.02** |
-
Average values from five grids per mice and three mice per group. Asterisks indicate significant value *<0.05, **<0.005.
RyR1AG mutation causes a defect in calcium release
Ryr1 functions as a muscle isotype of ryanodine receptor that is mechanically activated by the dihydropyridine receptor to allow Ca2+ release from the sarcoplasmic reticulum, thus evoking E–C coupling (Fill and Copello, 2002). To determine whether RyR1AG mutant protein affects calcium release, we examined the effects of treatment with 4-chloro-m-cresol (4-CmC), a potent activator of RyR1 in skeletal muscle and RyR2 in cardiac muscle (Zorzato et al., 1993; Choisy et al., 1999). The domain, which is activated by 4-CmC, is located between residues 4007 and 4180 of RyR1 (Fessenden et al., 2003), near the Ryr1AG mutation (E4242G). An increased sensitivity to 4-CmC has been linked to patients with malignant hyperthermia (Herrmann-Frank et al., 1996a; Baur et al., 2000) and ratiometric calcium imaging has been used to show hypersensitivity of 4-CmC in muscle cells from a patient with a mutation in RyR1 associated with malignant hyperthermia susceptibility (Roesl et al., 2014). Thus, we performed ratiometric Ca2+ imaging using the calcium indicator, Fura-2 AM, and recorded the ratio of 340/380 nm fluorescence over a range of 4-CmC concentrations up to 1000 μM (Zorzato et al., 1993; Herrmann-Frank et al., 1996b). 4-CmC was applied to explanted soleus muscle from 2-month old Ryr1AG/+ and littermate controls and ratiometric data of individual muscle fibers were normalized to the fluorescent ratiometric intensity at 1000 μM 4-CmC for each group. In wild-type mice, application of 200 μM 4-CmC induced measurable changes in Fura-2 signal (Figure 2A; 20 individual fibers from 4 mice). However, in littermate Ryr1AG/+ muscle fibers, measurable changes in Fura-2 signal were not observed until the application of 600 μM 4-CmC (Figure 2A; 27 individual fibers from 5 mice). These data suggest that the Ryr1AG/+ mutation results in decreased sensitivity to 4-CmC.
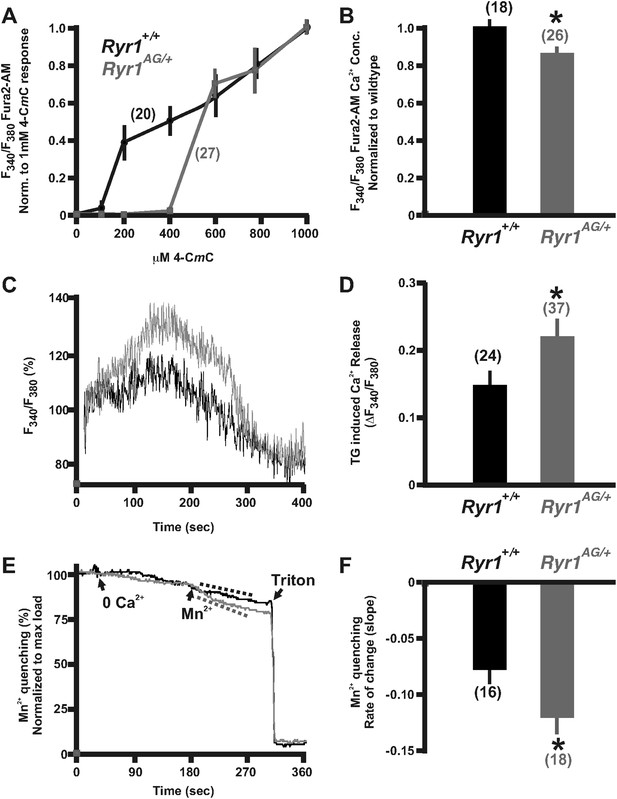
Calcium homeostasis is disrupted in Ryr1AG/+ muscle.
Fura-2 ratiometric imaging of myoplasmic Ca2+ in 2-month old muscle. (A) Ratiometric analysis of 4-CmC sensitivity in soleus muscles of wild-type (black) and Ryr1AG/+ littermates (grey). (B) Average ratiometric analysis of myoplasmic Fura-2 signal normalized to wild-type signal (n above bar represents number of analyzed fibers). (C) Representative fura-2 ratiometric signal of muscle fibers showing percent change after addition of 20 μM thapsigargin (TG) from wild-type (black) and Ryr1AG/+ (grey) littermate. (D) TG induced maximum response in muscle fibers (n above bar represents number of analyzed fibers). (E, F) Representation and quantification of Mn2+ quenching of Fura-2 fluorescence ratiometric signal illustrating increased influx of store operated calcium entry in soleus muscle fibers of Ryr1AG/+ (grey) muscle fibers compared to wild-type (black) muscle fibers (dash lines represent slope). In (E), arrows indicate when media was introduced with 0 Ca2+ followed by 0.5 mM Mn2+.
The reduced sensitivity of Ryr1AG to 4-CmC may suggest that less Ca2+ is released into the myoplasm upon RyR1 activation. In myotubes expressing malignant hyperthermia RyR1 cDNAs, myoplasmic Ca2+ is elevated due to a significant passive Ca2+ leak from the SR (Yang et al., 2007). However, in Ryr1AG/+ muscle fibers, we found that myoplasmic Ca2+ levels were significantly decreased compared to wild-type muscle fibers (Figure 2B; 85.3 ± 2.2% in Ryr1AG/+ muscle fibers normalized to wild-type concentration). This suggests that a Ca2+ leak that significantly elevates resting Ca2+ is not present in Ryr1AG/+ muscle fibers. In addition, the reduced sensitivity of Ryr1AG/+ muscle fibers to 4-CmC may suggest an increase in SR Ca2+. To examine SR calcium levels, 20 μM thapsigargin (TG), which inhibits SR Ca2+ ATPases, was applied to Fura-2 labeled muscle fibers. Ratiometric analysis of Ca2+ intensities provide evidence that Ryr1AG/+ muscle fibers have an increase in TG-dependent SR Ca2+ release (Figure 2C,D; 0.23 ± 0.05; 37 muscle fibers from 6 mice; p < 0.001) compared to wild-type muscle fibers (0.15 ± 0.03; 24 muscle fibers from 4 mice). This suggests increased levels of Ca2+ in the SR of Ryr1AG/+ muscle fibers and is consistent with the lack of a Ca2+ leak and decreased RyR1-mediated Ca2+ release from the SR in the mutant myofibers.
For muscle to function properly throughout the process of E–C coupling, the muscle fibers must maintain functional levels of Ca2+ within the SR. In response to reduced myoplasmic Ca2+, external Ca2+ enters the myofiber to replenish internal stores through store-operated calcium entry (SOCE). To measure unidirectional ion flux through SOCE, we used Mn2+ quenching of Fura-2 fluorescence in muscle fibers from Ryr1AG/+ and wild-type mice as Mn2+ has an increased affinity to Fura-2 compared to Ca2+, thus decreasing Ca2+-dependent myoplasmic fluorescence. To examine Mn2+ quenching, Fura-2 fluorescence was measured in muscle fibers first washed in 0 mM Ca2+, followed by Mn2+, and finally Triton-X 100 with EGTA to provide a baseline measurement. Ryr1AG/+ muscle fibers showed a substantially increased Mn2+ entry rate (−0.08 ± 0.02 rate in wild-type; n = 16 muscle fibers in 4 mice) compared to that of wild-type (Figure 2E,F; −0.2 ± 0.02 rate in wild-type; n = 18 muscle fibers in 4 mice). These data suggest that Ca2+ homeostasis is disrupted in Ryr1AG/+ muscle.
RyR1AG skeletal muscles show mitochondrial dysfunction
During excitation–contraction coupling, RyR1 floods Ca2+ into the myoplasm for ATP-dependent contraction of muscle fibers. Immediately following contraction, Ca2+ is pumped back into the SR and taken up by adjacent mitochondria for the purpose of ATP production. The decrease in myoplasmic calcium could be due to decreased release of Ca2+ from the SR or increased uptake of Ca2+ by the mitochondria, which prompted us to evaluate mitochondrial function. Local calcium release mechanisms or calcium ‘sparks’ communicate with adjacent mitochondria to spatially confine Ca2+ release (Rizzuto et al., 1993; Hajnoczky et al., 1995; Rizzuto et al., 1998). In skeletal myotubes, RyR1 and RyR3 generate calcium sparks (Ward et al., 2001; Weisleder et al., 2012), with RyR1 being the predominant ryanodine receptor expressed in limb skeletal muscles (Marks et al., 1989; Takeshima et al., 1989; Otsu et al., 1990; Zorzato et al., 1990). In cardiac muscle, the in vivo depletion of RyR2 is sufficient to reduce mitochondrial Ca2+ influx, oxidative metabolism, and ATP levels (Bround et al., 2013). Moreover, the activation of cytosolic Ca2+ sparks can lead to the influx of mitochondrial calcium termed Rhod-2 labeled calcium ‘marks’ (Pacher et al., 2002). Rhod-2 distributes to mitochondria when applied at low temperature and can be used as a measure of changes in fluorescence intensity (Trollinger et al., 1997). Influxes in mitochondrial calcium induce increased fluorescence intensities in Rhod-2 labeled mitochondria. In Ringer's solution, the number of mitochondria with changes in fluorescence intensity over 10 min of imaging were significantly decreased in Ryr1AG/+ compared to Ryr1+/+ littermate isolated muscle fibers (6.1 ± 1.1 compared to 10.2 ± 1.4 per 1000 μm2; Figure 3D), but the Rhod-2 intensity changes in mitochondria were increased after the application of 4-CmC to the bath in both Ryr1AG/+ and Ryr1+/+ isolated fibers (Figure 3A–D). Recent evidence in a mouse model with an induced mutation in RyR1 illustrates that leakage of SR Ca2+ induces ROS activation and mitochondrial dysfunction (Andersson et al., 2011). However, no difference in MitoSOX, an indicator of superoxides, was detected in Ryr1AG/+ compared to Ryr1+/+ littermate 2-month old soleus muscle fibers (Figure 3E). This is consistent with our data showing that Ryr1AG/+ muscle does not have an abnormal Ca2+ leak.
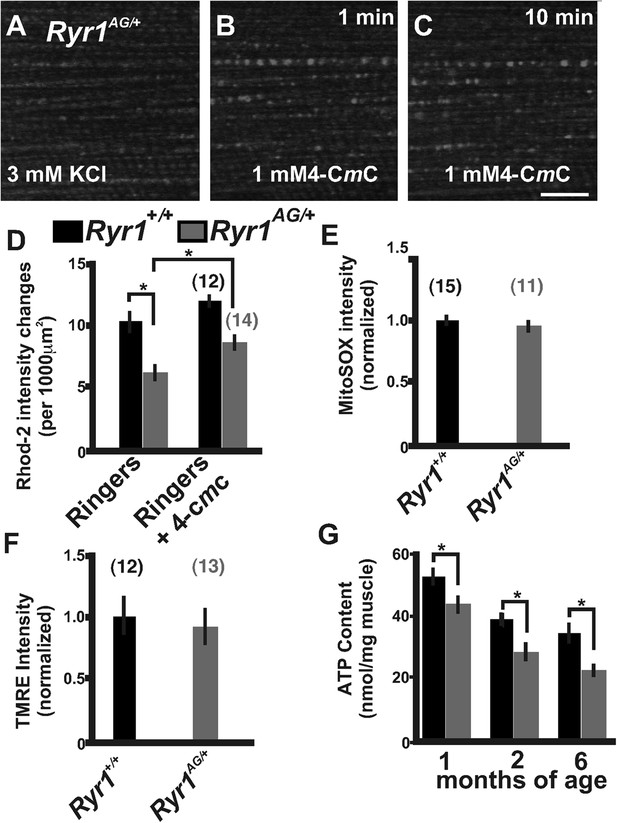
Defects in mitochondrial function in Ryr1AG/+ mice.
(A–C) Representative isolated fiber with Rhod2 fluorescence of 2-month old Ryr1AG/+ in 3 mM KCl (A) and 1 min (B) and 10 min (C) after application of 1 mM 4-CmC (punctate fluorescence are calcium marks). (D) Quantification of mitochondrial calcium marks visualized with Rhod2-AM from isolated soleus muscle fibers from Ryr1+/+ (black bars) and Ryr1AG/+ (gray bars) mice. (E) Superoxide labeling of isolated muscle fibers from Ryr1+/+ and Ryr1AG/+ mice. (F) Intensity of TMRE labeling of isolated soleus muscle fibers from Ryr1+/+ and Ryr1AG/+ littermates with saponin. The numbers on top of bars in graphs represent number of fibers examined. (G) ATP content from Ryr1+/+ and Ryr1AG/+ isolated soleus muscle from age-matched mice (n = 4 for each age and group). Scale bar = 5 μm.
The decrease in myoplasmic Ca2+ and Rhod-2 labeled mitochondria Ca2+ intensities, and the hyposensitivity in RyR1AG/+ channels may lead to a reduction in mitochondrial generation of ATP. Mitochondrial membrane potential drives the generation of ATP. To analyze mitochondrial membrane potential, we used the mitochondrial membrane potential indicator TMRE along with the amphipathic glycoside, saponin, to avoid the potentially confounding influence of variations in plasma membrane potential. Ryr1AG/+ isolated muscle fibers showed no significant difference relative to Ryr1+/+ in overall TMRE labeling of mitochondria (Figure 3F). Therefore, muscle was examined for total muscle ATP generation. ATP determination showed ∼30% less ATP in Ryr1AG/+ soleus muscle compared to age-matched Ryr1+/+ (1, 2, and 6-month old mice; n = 4 mice per group; Figure 3G). In human subjects that undergo fatigue, muscle fiber ATP levels can be reduced by up to 20% (Sahlin et al., 1997; Karatzaferi et al., 2001a, 2001b; Jones et al., 2009), suggesting that the reduced ATP levels in Ryr1AG/+ muscle might impair ATP-dependent enzyme activity during and after contraction (Homsher, 1987; Clausen et al., 1991; Smith et al., 2005; Barclay et al., 2007; Clausen, 2013b). These data suggest that dysfunctional Ca2+ release in RyR1AG muscle may influence mitochondrial function and ATP production. Together the SR Ca2+ release deficiencies and decreased mitochondrial ATP production may be sufficient to induce the myopathic phenotype in RyR1AG/+ mice.
Disruption of potassium homeostasis in Ryr1AG/+ muscle
The significant depletion of ATP in RyR1AG/+ muscle could consequently affect ATP-dependent mechanisms that normally act within the muscle, such as the influx of potassium to enhance membrane excitability. To determine whether potassium homeostasis is disrupted in Ryr1AG/+ muscle, we used the potassium indicator PBFI-AM (see ‘Materials and methods’) in combination with ratiometric potassium imaging of muscle fibers. Experiments using wild-type soleus in normal Ringer's solution (3 mM K+) showed that intracellular potassium concentration was 137 ± 7 mM (n = 23 fibers from 4 mice; Figure 4A,C). However, Ryr1AG/+ muscle showed lower intracellular potassium concentrations of 106 ± 8 mM (n = 29 fibers from 4 mice; p < 0.005; Figure 4B,C), a similar decline as that observed for wild-type muscles undergoing fatigue (McKenna et al., 2008). Moreover, Ryr1AG/+ muscle fibers showed a slow decrease in potassium fluorescent ratio intensity over the course of a minute suggesting an increase in K+ ion permeability (Figure 4D,E; n = 23 fibers in 4 Ryr1AG/+ mice and n = 29 fibers in 4 wild-type mice), which may be indicative of a potassium leak. We hypothesized that if Ryr1AG/+ muscles have a defect in potassium homeostasis due to an increase in K+ ion permeability, it might be possible to decrease the semi-permeability of the K+ ion with the addition of external K+. However, we did not observe a significant difference in serum K+ levels in 2-month old Ryr1AG/+ mice, but there was a 12 ± 3% increase in serum K+ in 6-month old Ryr1AG/+ mice relative to wild-type (Figure 4—figure supplement 1, n = 6 samples per group per age for Ryr1+/+ and Ryr1AG/+). The acute addition of 7 mM KCl to the muscle increased PBFI-AM fluorescence intensity, suggesting inhibition of a potassium leak, while 0 mM KCl decreased PBFI-AM fluorescence intensity (Figure 4D,E; n = 23 fibers in 4 Ryr1AG/+ mice and n = 29 fibers in 4 wild-type mice). Glibenclamide (2 μM) selectively binds to and inhibits SUR1/2 (Porat et al., 2011), subunits of the KATP6.1 and KATP6.2 channels in muscle (Pedersen et al., 2009). In wild-type adult mouse skeletal muscle, glibenclamide protects against fatigue caused by tetanic force (Duty and Allen, 1995). Additionally, in chick muscle fibers, glibenclamide increases the twitch and tetanus tension induced by application of caffeine, an agonist of RyR1 (Andrade et al., 2011). The acute addition of 2 μM glibenclamide to the 3 mM KCl bath resulted in increased intracellular K+ in wild-type (n = 17 fibers in 4 mice) and Ryr1AG/+ (n = 16 fibers in 4 mice) soleus muscle fibers (Figure 4D,F). Upon bath application of 7 mM KCl for 1.5 hr to examine a continuous counterbalancing of the K+ leak, the intracellular potassium concentration in the mutant soleus increased to 132 ± 8 mM, similar to wild-type (Figure 4G, n = 25 fibers in 4 Ryr1AG/+ mice and n = 26 fibers in 4 wild-type mice). Upon bath application of 2 μM glibenclamide for 1.5 hr, the intracellular potassium concentration in the mutant soleus increased to 138 ±12 mM, similar to wild-type (Figure 4H, n = 28 fibers in 4 Ryr1AG/+ mice and n = 28 fibers in 4 wild-type mice). The decline in intracellular K+ fluorescence in Ryr1AG/+ muscle compared to wild-type muscle (Figure 4A–E) and the increase in intracellular K+ upon KATP channel inhibition (Figure 4G,H) suggest an increase in KATP channel activity in Ryr1AG/+ muscle. These data suggest that increased extracellular K+ ion and inhibition of KATP channels might prevent the excessive K+ leak and protect the mutant muscle from decreased intracellular concentrations of K+.
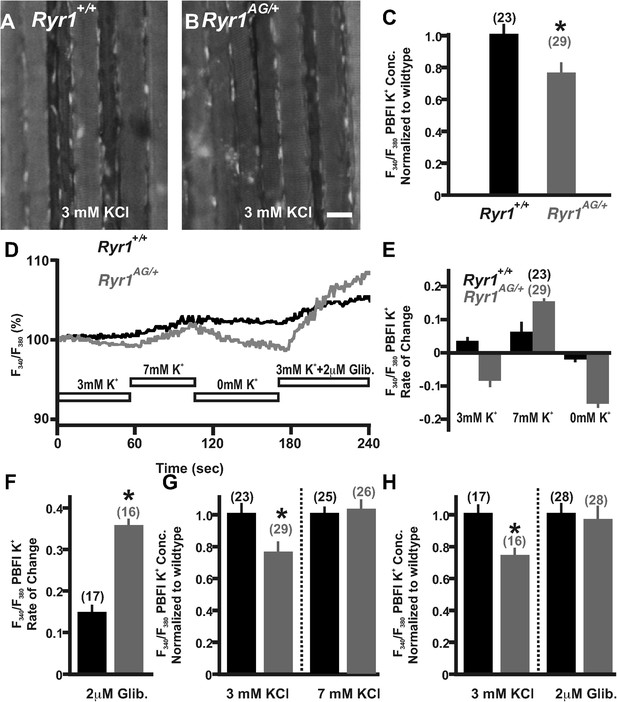
Detection and compensation of an internal potassium leak in RyR1AG/+ muscle.
Fluorescence imaging of PBFI at 340 nm in Ryr1+/+ (A) and Ryr1AG/+ (B) soleus muscle in 3 mM Ringer's solutions. (C) Ratiometric potassium imaging obtained at 340 and 380 nm wavelengths provided a ratio of fluorescence in Ryr1+/+ (black) and Ryr1AG/+ (grey) soleus muscle (normalized to Ryr1+/+). (D) Representation of the ratiometric imaging experimental paradigms used in Figure 4 showing bath applications of 3 mM KCl, 7 mM KCl, 0 mM KCl, and 3 mM KCl with 2 μM glibenclamide in Ringer's solutions. (E) Slope of intracellular K+ fluorescence intensities in experimental conditions. (F, H) Normalized intracellular K+ concentration in Ryr1+/+ (black) and Ryr1AG/+ (grey) soleus muscle in 3 mM KCL (F, H) compared to soleus from contralateral limb in 7 mM KCl Ringer's solutions (F) or 3 mM KCL plus 2 μM Glibenclamide (H) (muscle was bathed in solutions for 1.5 hr before imaging, n is the number of fibers examined from four mice). (G) Rate of change in PBFI fluorescence after acute bath application of 2 μM Glibenclamide. Scale bar = 20 μm.
Altered expression of genes and proteins involved in potassium homeostasis in Ryr1AG/+ muscle
The decreased ATP concentrations and ratiometric potassium data in Ryr1AG/+ muscle suggest that there may be an aberrant activation of mechanisms that regulate potassium efflux. Therefore, we examined several proteins involved in K+ homeostasis in muscle of 2-month old Ryr1AG/+ mice. As mitochondrial dysfunction may confound the analysis of whole muscle, we prepared membrane-enriched lysates in which the plasma membrane and t-tubules were isolated from the fraction containing mitochondria, peroxisomes, and lysosomes (see ‘Materials and methods’). The membrane-enriched preparations showed a 33 ± 6% increase in KATP6.2 protein in 2-month old Ryr1AG/+ soleus muscle compared to Ryr1+/+ littermates (Figure 5A,B; p < 0.005, the RNA encoding KATP6.2 (Kcnj11) was not altered in expression, Table 3, 0.6% diet), but no significant change in the protein expression of KATP6.1 or KIR2.1. KATP6.2 channels localize to skeletal muscle membranes and t-tubules (Banas et al., 2011), suggesting that KATP6.2 channel function may be increased, leading to increased potassium efflux and disrupted potassium homeostasis, and contributing to the weakness in Ryr1AG/+ muscle.
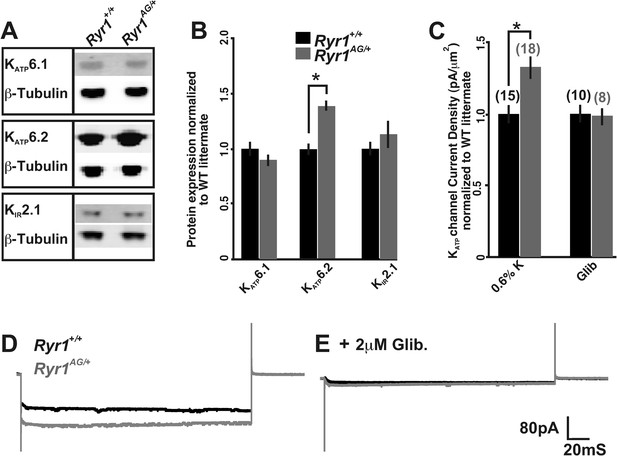
Altered activity of KATP channels involved in potassium transport.
(A) Western blots of membrane enriched lysate (that lacks mitochondria, peroxisomes, and lysosomes) from 2-month old wild-type and heterozygous Ryr1AG/+ soleus muscle analyzed for KATP6.1, KATP6.2, and KIR2.1. (B) Quantification of protein levels from Western blots of membrane enriched lysates. Each sample was first normalized to its own loading control and then the values from mutant and wild-type on the same blot were compared. Statistical analyses were determined from a minimum of 3 blots and at least two independent samples (n = 4 for each Western). (C) KATP current densities of isolated soleus muscle fibers from 2-month old wild-type and Ryr1AG/+ littermates with and without glibenclamide. Numbers on top of bars are number of fiber recordings. (D–E) Representative current density recordings with the same tip resistance from Ryr1+/+ and Ryr1AG/+ soleus muscle fibers (E) with and (D) without 2 μM glibenclamide.
Quantitative RT-PCR of muscles from 2-month old wild-type and heterozygous Ryr1AG/+ mice fed for 4 weeks on the indicated diets
Gene | 0.6% K Diet | 5.2% K Diet | ||
---|---|---|---|---|
Ryr1+/+ | Ryr1AG/+ | Ryr1+/+ | Ryr1AG/+ | |
Abcc8 (SUR1) | ||||
Vastus lateralis | 1.00 ± 0.07 | 1.30 ± 0.21** | 1.05 ± 0.30 | 1.51 ± 0.33* |
Tibialis anterior | 1.00 ± 0.04 | 1.20 ± 0.17*** | 1.09 ± 0.23 | 1.31 ± 0.27* |
Adductor magnus | 1.00 ± 0.12 | 1.28 ± 0.22* | 0.98 ± 0.23 | 1.61 ± 0.42* |
Abcc9 (SUR2) | ||||
Vastus lateralis | 1.00 ± 0.19 | 0.81 ± 0.24 | 1.03 ± 0.20 | 1.09 ± 0.18 |
Tibialis anterior | 1.00 ± 0.23 | 0.86 ± 0.21 | 0.99 ± 0.13 | 1.18 ± 0.20 |
Adductor magnus | 1.00 ± 0.18 | 0.88 ± 0.19 | 1.01 ± 0.19 | 1.11 ± 0.11 |
Atpa1 (NKAα1) | ||||
Vastus lateralis | 1.00 ± 0.15 | 1.23 ± 0.21* | 0.94 ± 0.32 | 0.18 ± 0.06*** |
Tibialis anterior | 1.00 ± 0.21 | 1.22 ± 0.13* | 0.75 ± 0.39 | 0.23 ± 0.06*** |
Adductor magnus | 1.00 ± 0.26 | 1.25 ± 0.17* | 0.93 ± 0.29 | 0.24 ± 0.07*** |
Clc1 (CLC1) | ||||
Vastus lateralis | 1.00 ± 0.09 | 1.05 ± 0.19 | 0.90 ± 0.21 | 0.84 ± 0.25 |
Tibialis anterior | 1.00 ± 0.10 | 1.10 ± 0.16 | 0.93 ± 0.11 | 0.82 ± 0.27 |
Adductor magnus | 1.00 ± 0.12 | 1.03 ± 0.21 | 0.89 ± 0.15 | 0.88 ± 0.17 |
Kcnj2 (KIR2.1) | ||||
Vastus lateralis | 1.00 ± 0.09 | 0.77 ± 0.12*** | 1.75 ± 0.17*** | 1.62 ± 0.13*** |
Tibialis anterior | 1.00 ± 0.17 | 0.68 ± 0.17*** | 1.70 ± 0.26*** | 1.55 ± 0.15*** |
Adductor magnus | 1.00 ± 0.18 | 0.59 ± 0.13*** | 1.67 ± 0.33** | 1.52 ± 0.18*** |
Kcnj8 (KATP6.1) | ||||
Vastus lateralis | 1.00 ± 0.20 | 0.56 ± 0.12*** | 0.97 ± 0.20 | 0.97 ± 0.23 |
Tibialis anterior | 1.00 ± 0.13 | 0.74 ± 0.19*** | 0.87 ± 0.11 | 0.91 ± 0.12 |
Adductor magnus | 1.00 ± 0.24 | 0.69 ± 0.16** | 1.16 ± 0.20 | 1.08 ± 0.17 |
Kcnj11 (KATP6.2) | ||||
Vastus lateralis | 1.00 ± 0.15 | 0.96 ± 0.17 | 1.10 ± 0.13 | 1.42 ± 0.14*** |
Tibialis anterior | 1.00 ± 0.18 | 1.01 ± 0.16 | 1.05 ± 0.08 | 1.34 ± 0.09*** |
Adductor magnus | 1.00 ± 0.17 | 0.94 ± 0.09 | 1.0 9± 0.06 | 1.38 ± 0.21*** |
Prkaa1 (AMPK) | ||||
Vastus lateralis | 1.00 ± 0.14 | 1.23 ± 0.15** | 1.11 ± 0.10 | 1.07 ± 0.14 |
Tibialis anterior | 1.00 ± 0.04 | 1.11 ± 0.06** | 1.08 ± 0.05 | 1.07 ± 0.10 |
Adductor magnus | 1.00 ± 0.11 | 1.20 ± 0.11*** | 1.01 ± 0.11 | 1.10 ± 0.06 |
-
Values normalized to GAPDH before normalization to Ryr1+/+ control on 0.6% diet. Asterisks indicates significant value * = 0.05, ** = 0.001, *** = 0.005.
An increased number of KATP channels in the membrane (or a change in activity) would be expected to alter current densities. In rat skeletal muscle, KATP6.2 pores have a single-channel conductance of around 80 pS, although the current densities (pA) of KATP channels can vary between muscles (Tricarico et al., 2006). To examine current densities of KATP channels in soleus muscle fibers, inside-out patch excision in ATP-free solutions was performed. Currents from excised patches of 2-month old wild-type soleus muscle fibers were −106 ± 12 pA with an average pipette resistance of 2.2 ± 0.4 MΩ after −60 mV voltage injection (n = 10 fibers from 3 mice). In 2-month old Ryr1AG/+ soleus muscle fibers, the currents increased to −142 ± 24 pA with an average pipette resistance of 2.3 ± 0.3 MΩ (n = 10 fibers from 3 mice). Thus, Ryr1AG/+ soleus muscle fibers showed a 34 ± 9% increase in normalized current densities compared to wild-type littermates (Figure 5C,D). Glibenclamide addition removed the current differences between Ryr1+/+ and Ryr1AG/+ muscle, suggesting that the channels opened in the inside-out patch were KATP channels (Figure 5C,E, n = 8 fibers from three mice for both Ryr1+/+ and Ryr1AG/+). These data indicate an increase in KATP channel activity in the membrane of Ryr1AG/+ muscle and this could explain the increased potassium efflux in spite of the decreased ATP levels in mutant muscle.
Potassium transport activity of KATP channels is positively regulated by the AMP-activated protein kinase AMPK (Prkkaa1) (Wang et al., 2005), and this transcript was upregulated in 2-month old Ryr1AG/+ muscles (Table 3, 0.6% diet). The sulfonylurea receptors (SUR) are ATP-binding cassette (ABC) transporters and subunits of KATP channels. SUR1 (Abcc8), which binds to KATP6.2, was significantly upregulated in Ryr1AG/+ muscles at the RNA level (Table 3). Thus, the increased expression of genes that encode proteins upstream of KATP6.2 channels or that interact with KATP6.2 channels might contribute to the increased activity of KATP6.2 channels in membrane and t-tubules. ClC-1-dependent chloride conductance is increased during muscle fatigue (Pedersen et al., 2009), yet ClC-1 transcripts were not significantly altered in Ryr1AG/+ muscle compared to Ryr1+/+ littermates. Ryr1AG/+ muscle showed a rapid increase in intracellular potassium when KATP channels were blocked (Figure 4C,D,F), suggesting that mechanisms of K+ transport into the cell are active in these muscles but may not be able to overcome the potassium leak and compensate for decreased intracellular K+. Na+, K+-ATPase activity maintains Na+ and K+ equilibrium at the membrane in the muscle (Kristensen and Juel, 2010). Transcripts of the pump subunit Na+, K+-ATPase α1 were increased in Ryr1AG/+ muscle (Table 3). Taken together, these data suggest that expression levels of genes and proteins in the potassium transport pathway are dysregulated when RyR1-dependent calcium release is disrupted.
Amelioration of myopathic phenotype through potassium supplementation
As our in vitro studies showed that increased extracellular K+ leads to a normalization of intracellular K+ in Ryr1AG/+ muscles, we then asked whether increased serum K+ could alter the in vivo phenotype of Ryr1AG/+ mice. Serum potassium levels can be increased through diet (Christensen et al., 2010) or with the angiotensin-converting enzyme (ACE) inhibitor, enalapril (Cleland et al., 1985). Therefore, we asked whether these treatments might decrease K+ ion permeability in muscle and whether this could ameliorate the physiological and pathological symptoms of the myopathy in Ryr1AG/+ mice. To test these hypotheses, Ryr1AG/+ and Ryr1+/+ mice were weaned onto 0.6% K+ (level of potassium in standard mouse chow), and four weeks later, when muscle weakness is already observed (Figure 1B,C), they were given either 5.2% K+ diet or enalapril (0.02 mg/ml in drinking water) for an additional four weeks (Figure 6—figure supplement 1, for blood pressure readings). Examination of soleus muscle fibers from these mice showed that internal potassium concentrations were increased in Ryr1AG/+ soleus fibers, similar to wild-type, following 5.2% K+ diet (145 ± 22 mM compared to 147 ± 20 mM; Figure 6A) or with enalapril (147 ± 18 mM compared to 148 ± 25 mM; Figure 6A). As noted in Figure 1, weakness in 2-month old Ryr1AG/+ mice on 0.6% K+ diets was clearly evident with an ∼50% reduction in grip strength and inability to hang onto the wire, but in line with our hypothesis, Ryr1AG/+ mice treated with enalapril showed a significant improvement in the wire hanging task (Figure 6B,C). Most dramatically, the 5.2% K+ diet rescued muscle strength in Ryr1AG/+ mice (Figure 6B,C). Thus, potassium supplementation and an FDA-approved drug can rescue muscle weakness in mice carrying the Ryr1AG mutation.
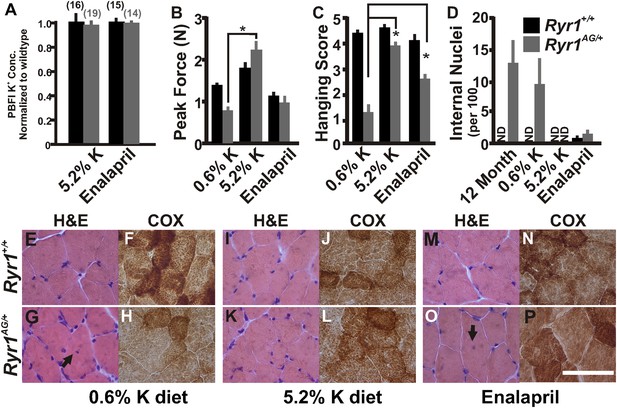
Increased potassium diet can rescue muscle strength and reverse the CCD histology and myopathy.
(A) Normalized internal potassium concentrations of soleus muscle from 2-month old Ryr1+/+ and Ryr1AG/+ mice fed 0.6% K+ diet for 4 weeks, then placed on 5.2% K+ diet or 0.6% diet + enalapril for 4 weeks, and then bath exposed to different extracellular potassium concentrations. (B, C) Average grip strength (B, five trials/mouse and 5 mice/set; p values <0.001) and in vivo hanging task (C, 10 trials/mouse, n = 5 per set; p values <0.001) assayed from 2-month old Ryr1+/+ (black bar) and Ryr1AG/+ (grey bar) mice maintained for 4 weeks on control 0.6% K+ diet, 5.2% K+ diet, or 0.6% K+ diet supplemented with enalapril. (D) Quantification of number of internalized nuclei per 100 myofibers in 12-month old mice or in 2-month old mice maintained for 4 weeks on control 0.6% K+ diet, 5.2% K+ diet, or 0.6% K+ diet supplemented with enalapril (n = 10 per muscle, n = 3 per set of muscles for total of n = 30; p values <0.001). (E–P) Vastus lateralis myofibers from 2-month old Ryr1+/+ and Ryr1AG/+ mice maintained for 4 weeks on control 0.6% K+ diet, 5.2% K+ diet, or 0.6% K+ diet supplemented with enalapril. Cross-sections stained with H&E (left panels) and COX (right panels). Ryr1AG/+ mice on 5.2% K diet show increased COX staining and no internalized nuclei, similar to Ryr1+/+. These pathological features are still observed in Ryr1AG/+ mice on 0.6% K+ diets. Enalapril increases COX staining but some internalized nuclei are observed, even in Ryr1+/+. Scale bar = 50 μm; error bars as standard error of the mean (SEM).
Histological examination is the hallmark diagnosis of RyR1-related myopathies. On control 0.6% K+ diets, internally located nuclei and decreased mitochondrial activity were detected in Ryr1AG/+ muscle (Figure 6D,E–H). Strikingly, muscle from Ryr1AG/+ mice on high-potassium diet resembled RyR1+/+ muscle (Figure 6D,I–L), while enalapril treatment showed a less dramatic improvement in muscle histology (Figure 6D,M–P). The number of Z line streaming areas was also significantly reduced by these therapies (Table 2). Thus, even after observable muscle dysfunction, myopathic pathology can be rescued with increased extracellular potassium.
Chronic treatment of Ryr1AG/+ animals for 1 month with a 5.2% potassium diet returned KATP6.2 protein expression to levels similar to those seen in diet-treated Ryr1+/+ littermates (Figure 7A). We also noted that protein levels of the inwardly rectifying potassium channel KIR2.1 were increased by 64 ± 23% in Ryr1AG/+ muscle following 5.2% K+ diet (Figure 7A, p < 0.01), suggesting that increased KIR2.1 channel activity may also assist in rectifying the K+ ion leak. This physiological rescue and the normalization of KATP expression suggest a decrease in KATP channel activity. KATP current density recordings in Ryr1AG/+ soleus muscle fibers following 5.2% potassium diet were returned to levels similar to wild-type on normal diet (−107 ± 16 pA in 2.0 ± 0.3 MΩ), whereas currents in Ryr1+/+ on 5.2% diet were substantially decreased (−74 ± 15 pA in 2.1 ± 0.4 MΩ; Figure 7B). ATP levels were also increased by 5.2% potassium diet in Ryr1AG/+ soleus muscle, similar to Ryr1+/+ (38.5 ± 6.2 and 41.1 ±8.3 nmol ATP/mg soleus, respectively on 5.2% diet; Figure 7C). Moreover, the 5.2% K+ diet increased mitochondrial calcium marks in isolated fibers from Ryr1AG/+ soleus muscles to a rate similar to wild-type littermates, with no effect on ROS activity (Figure 7D,E). These data indicate a rescue of the myopathic muscle physiology by diet, indicating potential therapeutic avenues for RyR1-related myopathies in human.
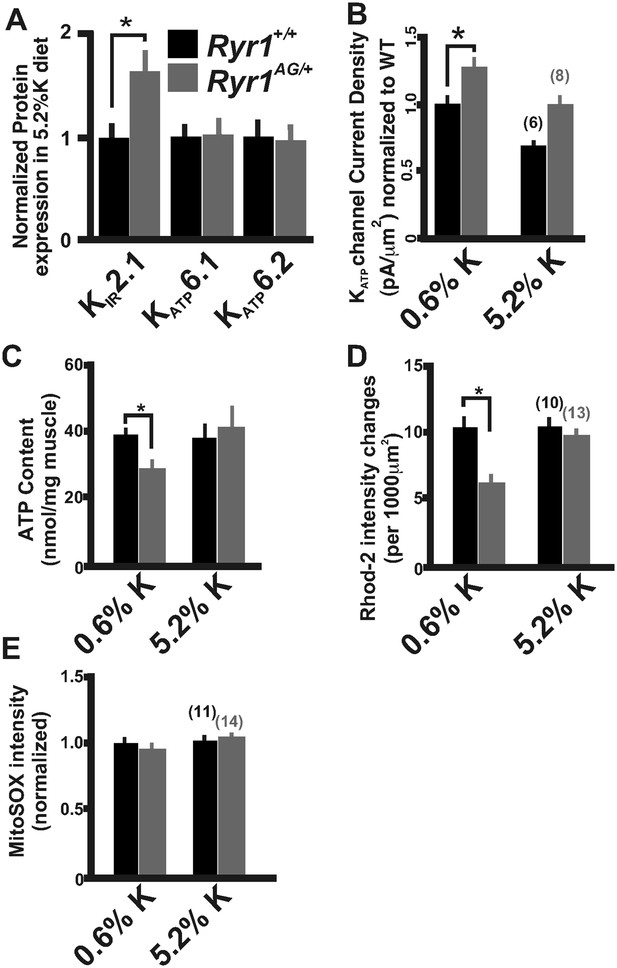
Increased potassium diet influences KATP channel activity and mitochondrial function.
(A) Membrane enriched lysate (lacking mitochondria, peroxisomes, and lysosomes) from soleus muscle of 2-month old Ryr1+/+ and Ryr1AG/+ mice fed 5.2% potassium supplemented diet for 4 weeks analyzed by Western blot for KATP6.1, KATP6.2, and KIR2.1 (* is p < 0.001). (B) KATP current densities of isolated soleus muscle fibers from Ryr1+/+ and Ryr1AG/+ littermates after 4 weeks on 5.2% K diet compared to 0.6% K diet. (C) ATP content from Ryr1+/+ and Ryr1AG/+ isolated soleus muscle from mice on 0.6% or 5.2% potassium diet. (D) Quantification of calcium marks visualized with Rhod2-AM from isolated soleus muscle from Ryr1+/+ and Ryr1AG/+ mice with and without diet therapy. (E) Superoxide labeling of isolated soleus muscle from Ryr1+/+ and Ryr1AG/+ mice with and without therapy. Error bars as standard error of the mean (SEM).
Amelioration of myopathic phenotype through glibenclamide
Glibenclamide can inhibit KATP channels, which are overexpressed in the mutant muscle (Figure 5A), and rectify the K+ ion leak when acutely applied in vitro (Figure 4C). To extend these findings in vivo, we asked whether this FDA-approved drug could ameliorate the myopathic pathology in mice. To test this, glibenclamide (15 mg/kg/day) was orally administered to 1-month old mice for 30 days. Ryr1AG/+ mice treated with glibenclamide showed significantly increased grip strength (Figure 8A,B). ATP content and mitochondrial calcium changes in intensities in glibenclamide treated Ryr1AG/+ mice were increased to similar levels as Ryr1+/+ mice (36.4 ± 3.9 and 39.3 ± 4.3 nmol ATP/mg soleus muscle, respectively; n = 4 mice per condition, Figure 8C; 11.8 ± 1.5 and 11.1 ± 1.8 mitochondrial changes in intensities, respectively; n = 8 muscle fibers for each group, Figure 8D) with no alteration in MitoSOX labeling (data not shown). Ryr1+/+ and Ryr1AG/+ mice on glibenclamide therapy showed no significant difference in internal potassium concentrations (140 ± 9 mM in Ryr1+/+ soleus fibers compared to 145 ± 28 mM in Ryr1AG/+ soleus fibers; n = 17 and 20 muscle fibers, respectively; normalized in Figure 8E). Current densities from Ryr1AG/+ soleus muscle fibers from mice treated with glibenclamide were −82 ± 13 pA with an average pipette resistance of 2.1 ± 0.3 MΩ (n = 8 fibers from 4 muscles; Figure 8F) and showed no significant difference from wild-type littermates on glibenclamide (−93 ± 18 pA with 2.2 ± 4 MΩ tip resistance, n = 8 fibers from 4 muscles; Figure 8F). Furthermore, a significant decrease in internal nuclei was detected in Ryr1AG/+ muscles (9.8 ± 5.2 in 0.6% K+ diet compared to 4.8 ± 2.7 in glibenclamide diet; n = 4 mice per condition; Figure 8G). Administration of glibenclamide also rescued the histological manifestations of the myopathy in Ryr1AG/+ mice (Figure 8H–K, Table 2), suggesting an alternative treatment with an FDA-approved drug that does not involve modulation of serum potassium levels. Together our in vivo data suggest that the mitochondrial dysfunction in Ryr1AG/+ soleus muscle is significantly improved through 5.2% potassium diets and glibenclamide administration. These studies suggest that rescue of the weakened state of muscles in this mouse myopathic model are, in part, due to a potassium-dependent mechanism.
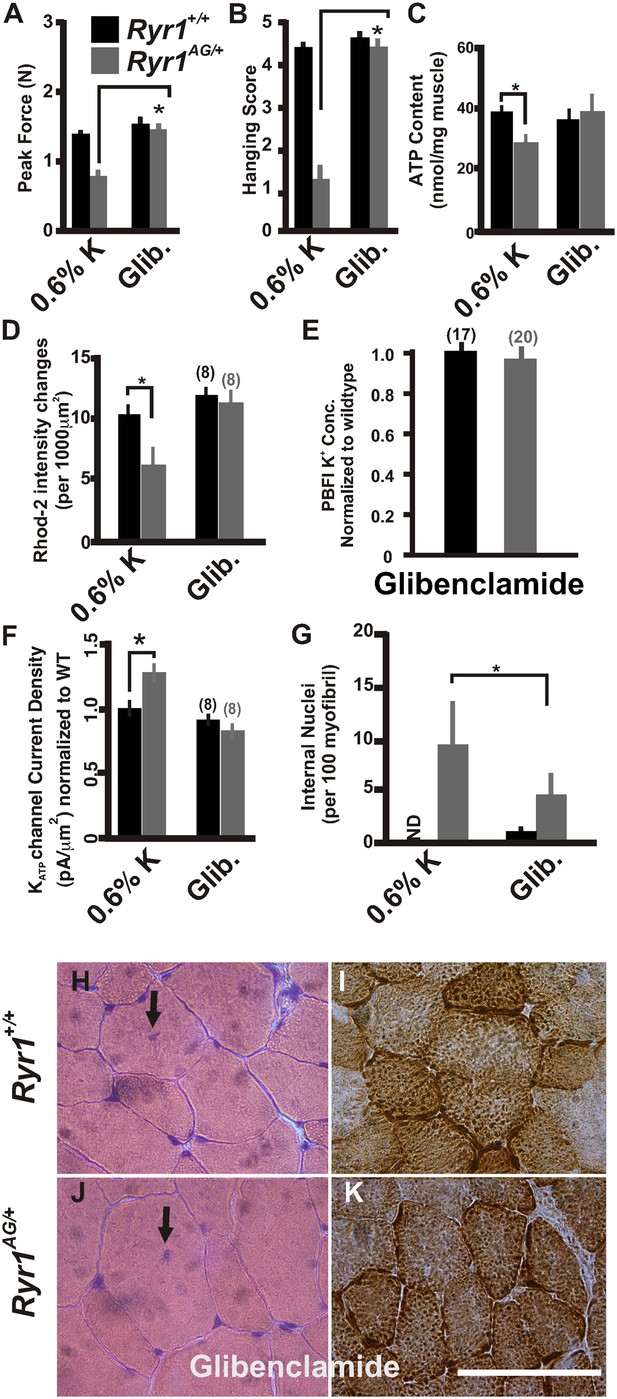
Inhibition of KATP channels can reverse the histological and myopathic phenotypes.
(A, B) Average grip strength (A, five trials/mouse and 5 mice/set; p values <0.001) and in vivo hanging task (B, 10 trials/mouse, n = 5 per set; p values <0.001) assayed from 2-month old Ryr1+/+ (black bar) and Ryr1AG/+ (grey bar) mice maintained for 4 weeks on 0.6% K diet and glibenclamide (15 mg/kg/day). (C) ATP content from soleus muscle of Ryr1+/+ and Ryr1AG/+ mice after 4 weeks on 0.6% K diet without or with glibenclamide (n = 4 muscles per condition). (D) Quantification of calcium marks visualized with Rhod2-AM from isolated soleus muscle from Ryr1+/+ and Ryr1AG/+ mice with and without glibenclamide therapy. (E) Normalized internal potassium concentrations after glibenclamide therapy. (F) KATP current densities of isolated soleus muscle fibers from Ryr1+/+ and Ryr1AG/+ littermates after 4 weeks on glibenclamide therapy compared to 0.6% K diet. (G) Quantification of number of internalized nuclei per 100 myofibers in all conditions (n = 10 per muscle, n = 3 per set of muscles for total of n = 30; p values <0.001). (H–K) Vastus lateralis myofiber from Ryr1+/+ and Ryr1AG/+ mice maintained for 4 weeks on glibenclamide. Arrow in Ryr1+/+ myofiber (F) shows the rare occurrence of internal nuclei. Cross-sections stained with H&E (left panels) and COX (right panels). Ryr1AG/+ mice on glibenclamide show increased COX staining, similar to Ryr1+/+. Scale bar = 50 μm; error bars as standard error of the mean (SEM).
High-potassium diet affects biomarkers for potassium homeostasis
The levels of protein and gene expression might serve as measurements of recovery in Ryr1AG/+ mice following administration of a high-potassium diet. Indeed, as shown in Figure 7A and Table 3, we found that expression of proteins or transcripts that encode KATP6.1, KATP6.2, AMPK, and SUR2 were returned to normal levels on high-potassium diet in Ryr1AG/+ adult muscle, whereas Na+, K+-ATPase α1 transcripts were considerably decreased and KIR2.1 protein was increased in high-potassium diet in Ryr1AG/+ muscle. Thus, potassium supplementation rescues the expression of key molecules required for potassium homeostasis in RyR1AG/+ muscle, suggesting that KIR2.1, KATP6.1, KATP6.2, Prkaa1, Abbc9, and Na+, K+-ATPase α1 are potential biomarkers for myopathies associated with mutations in RyR1 and for evaluation of future potential therapies.
CCD patient muscles show upregulation of potassium homeostasis biomarkers
Central core disease is the most common diagnosed myopathy due to mutations in RyR1 (Jungbluth, 2007). Using the molecular insights gained from our myopathic mouse model, we examined these biomarkers in RNA isolated from human skeletal muscles collected from CCD patients and controls without muscle disease (normalized to a pooled control muscle sample, see methods). Expression levels of potential biomarkers from human muscle samples of healthy individuals, patients with RyR1 mutations causing CCD, and patients with CCD without identified RyR1 mutations were compared (Table 4; Figure 9). While PRKAA1 was increased in all muscle biopsies compared to the pooled human RNA sample, excluding it as a useful biomarker, all CCD patients showed a significant increase in expression of ABCC8 (SUR1), KCNJ2 (KIR2.1), KCNJ8 (KATP6.1), and KCNJ11 (KATP6.2), indicating that these four transcripts may be useful biomarkers for CCD in humans. Together, these data suggest specific biomarkers that could aid in diagnosis and treatment of CCD.
Relative variation of quantitative RT-PCR of control RNA and RNA from human muscle biopsies
Patient | RYR1 Mutation | Congenital Myopathy | ABCC8 (SUR1) | ATPA1 (NKAα1) | KCNJ8 (KATP6.1) | KCNJ11 (KATP6.2) | PRKAA1 (AMPK) |
---|---|---|---|---|---|---|---|
1 | No | No | 0.98 ± 0.02 | 1.35 ± 0.05 | 2.15 ± 0.19 | 1.59 ± 0.39 | |
2 | No | No | 0.94 ± 0.08 | 0.85 ± 01 | 1.06 ± 0.06 | 0.78 ± 0.16 | |
3 | Yes | Yes | |||||
4 | Yes | Yes | |||||
5 | Yes | Yes | |||||
6 | Yes | Yes | |||||
7 | Yes | Yes | |||||
8 | Yes | Yes | |||||
9 | Yes | Yes | |||||
10 | No | Yes | 1.09 ± 0.11 | ||||
11 | No | Yes | 1.09 ± 0.10 |
-
Values normalized to GAPDH before normalization to pooled control human muscle RNA.
indicates significant value ; indicates significant value .
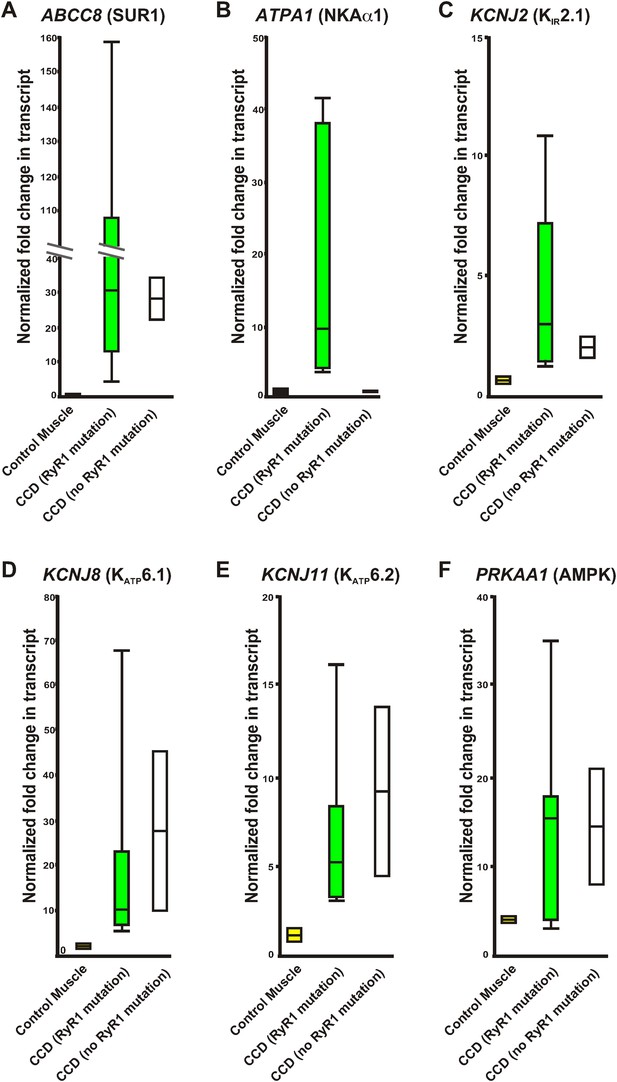
Relative variation of quantitative qRT-PCR of human control RNA and RNA from human muscle biopsies.
Whisker plots derived from the data in Table 2 of control muscle (yellow), congenital myopathy (CCD) with a mutation in RyR1 (green), and without a mutation in RyR1 (white). (A) ABCC8, (B) ATPA1, (C) KCNJ2, (D) KCNJ8, (E) KCNJ11, (F) PRKAA1. Values normalized to GAPDH before normalization to pooled control human muscle RNA.
Discussion
In this study, we present a myopathic mouse model with core-like pathology that illuminates mechanisms underlying muscle weakness and mitochondria dysfunction. First, we identified a mutation in RyR1 that induces a myopathy with ultrastructural hallmarks of cores similar to the Ryr1I4895T/+ mutant mouse, a known mouse model of CCD (Boncompagni et al., 2009, 2010). Second, we used this myopathic mouse model to identify a novel link between decreased internal calcium (Figure 2), decreased mitochondrial ATP production (Figure 3), and altered potassium homeostasis (Figure 4), mechanisms that are important in controlling excitability in muscle. Third, we provide evidence that KATP channels are elevated in the membrane and overactive, leading to increased potassium efflux in this myopathic mouse model, which could have direct effects on membrane excitability. Fourth, we provide clinically relevant findings that suggest a potassium-enriched diet, perhaps in combination with the sulfonylurea class of anti-diabetic drugs, which are potential therapies for myopathies with defects in potassium homeostasis. Using multiple assays and different muscles with different fiber type compositions, our data are consistent and show muscle weakness, pathological changes, and gene expression changes. The consistency of our data between muscle types further strengthens our hypotheses and conclusions. It should be noted that malignant hyperthermia, a disease associated with RyR1 mutations, is sensitive to potassium chloride (Moulds and Denborough, 1974), and therefore a testing method in patients or biopsy samples need to be initiated prior to potassium supplementation. Importantly, our work identifies potential diagnostic biomarkers of disease in patients with CCD and potentially non-RyR1 myopathies, which could be utilized to identify patients who may be candidates for potassium modulation-based therapies.
The physiological mechanisms underlying RyR1-related myopathy are not well defined. One histological hallmark of RyR1-related myopathy is the presence of muscle cell cores, which indicate focal mitochondrial loss and decreased ATP production (Boncompagni et al., 2009, 2010; Jungbluth et al., 2011). The Ryr1AG/+ mutant mouse muscles have decreased intracellular calcium, core-like structures and decreased ATP content, as well as other ultrastructural hallmarks of core-like diseases. Data from our model suggest that proper calcium release from internal stores is required for mitochondrial production of ATP and increased ATP levels, which in a feedback loop can then stimulate RyR1-dependent calcium release (Meissner et al., 1986; Laver et al., 2001). As muscle ATP decreases, KATP channels open to efflux K+ from the myoplasm to the interstitial space, which may protect against excessive muscle contraction. Efflux of K+ into the t-tubules depolarizes the tubular membranes and inhibits the opening of sodium channels (Fraser et al., 2011). Sodium channel inhibition decreases the probability that a nerve-derived action potential will activate DHPR receptors that mechanically activate Ryanodine receptors, and thus myoplasmic release of stored Ca2+ is reduced. Therefore, in the Ryr1AG/+ model, RyR1 dysfunction, decreased internal Ca2+ release, potassium leakage and increased KATP-dependent efflux may potentiate muscle weakness and muscle deterioration associated with core-like structures. The chronic low-level calcium uptake into the mitochondria may then induce long-term mitochondrial dysfunction. The histological appearance of cores in the center of the muscle but more normal staining near the plasma membrane may be explained by the possibility that more centralized mitochondria receive less calcium, whereas SOCE and calcium influx from external sources at the plasma membrane may provide buffering to mitochondria that are adjacent to the plasma membrane.
Our data suggest that the SR calcium release deficiencies and decreased mitochondrial ATP production might be sufficient to induce the myopathic phenotype in RyR1AG/+ mice. ATP provides the energy to cause muscle contraction, and relative ATP/ADP levels are important in providing information about muscle fatigue and ion homeostasis to the cell. In skeletal muscle, 90% of ATP is used for muscle contractions by the myosin ATPase, Ca2+ ATPase, and Na+/K+ ATPase (Homsher, 1987; Clausen et al., 1991; Smith et al., 2005; Barclay et al., 2007; Clausen, 2013b). Additional ATP-dependent enzymes may be particularly sensitive to a 30% loss of ATP in RyR1AG/+ muscle and this might contribute to a significant effect on excitability and membrane homeostasis after muscle contraction and fatigue. During skeletal muscle fatigue and fluctuations in potassium homeostasis, KATP channels open and efflux potassium from the muscle (Pedersen et al., 2009). Moreover, plasma membrane and t-tubule KATP channels become more active as ATP is utilized and ADP accumulates (Baczko et al., 2005). KATP channels are mechanistically linked to the DHPR-RyR1 complex (Tricarico et al., 1999) but the ATP binding sites within RyR1 are at a considerable distance from the point mutation in the RyR1AG protein (Popova et al., 2012). Finally, KATP channel activity increases potassium conductance within the t-tubules after an action potential (Gramolini and Renaud, 1997; Cifelli et al., 2008; Banas et al., 2011). These data suggest a mechanistic link between E–C coupling, RyR1 function, ATP levels, KATP channel function, and potassium homeostasis.
In our mouse model of a myopathy with core-like structures, increased intake of dietary potassium increases interstitial K+, which leads to an increase in myoplasmic K+. The resulting increase in interstitial K+ might counterbalance the K+ leak permeability and increase membrane excitability. A more excitable membrane increases the likelihood of voltage-dependent DHPR-RyR1 release of calcium from the SR during an action potential. Increased calcium in the myoplasm will enhance mitochondrial uptake of calcium. We propose that the waves of increased calcium into the mitochondria enhance ATP production (Griffiths and Rutter, 2009), which inhibits KATP channel opening and reverses the K+ leak, and stimulates RyR1-dependent calcium release from internal stores (Meissner et al., 1986; Laver et al., 2001), further perpetuating mitochondrial ATP production in this model of myopathy in which the phenotype can be ameliorated by increased potassium. However, we cannot rule out the possibility that the potassium supplementation may work indirectly at the muscle by depolarizing the locomotor circuit to increase neurotransmitter release at the neuromuscular junction and potentiate the voltage-dependent DHPR-RyR1 release of calcium.
Defective potassium mechanisms appear to be the hallmark of Ryr1AG/+ mice as evidenced by 1) increased KATP channel current that would drive K+ efflux, 2) decreased internal K+ concentrations, and 3) a potassium leak. Altogether this may suggest an increase in interstitial K+, but serum K+ levels may not correlate with interstitial K+ levels (Clausen, 2013a) and increased interstitial K+ would be expected to be rapidly removed by diffusion and through the capillary network of blood vessels. We did not observe a significant difference in serum K+ levels in 2-month old Ryr1AG/+ mice, but there was an increase in serum K+ in 6-month old Ryr1AG/+ mice relative to wild-type (Figure 4—figure supplement 1). In vitro, the rapid rise in intracellular K+ in Ryr1AG/+ muscle bathed in 3 mM K+ and treated with glibenclamide, an inhibitor of KATP channels, is in part due to the transport of K+ into the myoplasm through KIR2.1 and Na+, K+-ATPases. These mechanisms that transport K+ into the myoplasm in Ryr1AG/+ muscles may not be sufficient in vivo to compensate for the K+ leakage or the increased activation of KATP channels located on the plasma membrane. We suggest that Ryr1AG/+ myopathic muscles have a K+ leak that persistently decreases intracellular K+ and leads to a loss of membrane excitability.
Amelioration of muscle weakness through low-cost potassium supplementation could have profound impact on patients suffering from core myopathies and these data may have important implications for other disease states. Recent guidelines from the World Health Organization suggest that the world population is consuming inadequate levels of dietary potassium, which can lead to heart disease, stroke, respiratory distress, and increased fatigue (Hartl, 2013). Our studies suggest that Ryr1AG/+ muscle is in a myopathic state due to decreased intracellular Ca2+ levels, decreased K+ levels, decreased ATP levels and increased activation of KATP6.2 channels, which may mimic a fatigue-like state. Currently, myopathies with mutations in RyR1 are not considered metabolic myopathies, even though RyR1-S2844D mice with an intracellular calcium leak and muscle weakness exhibit decreased levels of mitochondrial activity and metabolic enzymes (Andersson et al., 2011). Considering that our data suggest mitochondrial dysregulation of metabolic pathways and potassium signaling in Ryr1AG/+ muscle and muscles from patients with CCD, we were intrigued by the findings that some fatigue-inducing metabolic myopathies can be rescued through dietary potassium supplementation (Villabona et al., 1987; Caradonna et al., 1992; Ismail et al., 2001). In the future, it will be of interest to determine whether the positive response to increased potassium levels is applicable to myopathies due to other RyR1 mutations and to other genetic causes. Our data also suggest that muscles of human CCD patients are sensitive to pathways that mediate potassium homeostasis. ATPA1 (Na+, K+-ATPase α1) showed increased expression only in CCD patients with mutations in RYR1, suggesting a selective sensitivity to this ATP-sensitive transporter. With our suggestion that KATP6.2 channel inhibition may help protect skeletal muscles from the formation of cores, one might predict that the human patient data would show consistently higher levels of KCNJ11 (KATP6.2) transcripts in the muscle biopsies. This is the case in CCD patients, although the level is variable. This variability could be due to the location of the muscle biopsy, as KATP6.2 channels in mice are more highly expressed in glycolytic muscle than oxidative skeletal muscle fibers (Banas et al., 2011). These biomarkers may therefore provide a less invasive approach to identify patients with CCD myopathies and may eventually identify patients who are suitable candidates for treatment of CCD through modulation of potassium signaling pathways.
Materials and methods
Forward genetic screen and mouse strains
Request a detailed protocolENU mutagenesis was performed as described (Kasarskis et al., 1998) on males of C57BL/6J background and then outcrossed onto 129S1/Svlmj background to score G3 embryos at embryonic day 18.5 for recessive mutations that affect embryonic locomotion. The Ryr1m1Nisw allele showed muscle weakness when heterozygous (described here) and a non-motile embryonic phenotype when homozygous (unpublished results). The gene mutation was mapped using data from homozygous mutants, starting with a panel of 96 MIT and SKI SSLP markers, which mapped the Line1–4 mutation to the proximal third of chromosome 7. The genetic region containing the mutation was narrowed to 3 Mb by the use of additional MIT SSLP markers on chromosome 7 and further meiotic mapping which followed linkage between the phenotype and C57BL/6J markers. As Ryr1 was a strong candidate gene in the critical interval, a complementation cross was performed between Ryr1AG (Ryr1m1Nisw) and Ryr1 tm1TAlle (dyspedic null allele generated by Richard Allen, [Buck et al., 1997]). These alleles did not complement as E18.5 trans-heterozygotes showed a similar non-motile phenotype as homozygotes of either allele. To identify the mutation in Line1-4, genomic DNA in overlapping segments of the Ryr1 gene was amplified by PCR from phenotypic E18.5 embryos and compared with control E18.5 C57Bl/6J DNA. Subsequently, embryos were genotyped as follows: tissue was placed in tail lysis buffer (100 mM Tris–Cl, pH 8.0, 5 mM EDTA, 0.2% SDS, 200 mM NaCl) overnight. DNA was amplified using Taqman Gold (Applied Biosystems) with primer set to RyR1 mutation (forward primer: GTGGAGTGTGGTGCTGTATATC forward and CCGTCACTGTCACCTTCTTG reverse primers). PCR amplification was performed for 35 cycles at 55°C. PCR product was sent to Barbara Davis Center Molecular Biology Service Center at the University of Colorado Denver for sequencing. For non-sequencing genotyping, the MIT SSLP markers, D7MIT114 and D7MIT267, were amplified to examine linkage between phenotypic background (C57BL/6J) and control background (129/SvJ). Transcript accession for RyR1 was NM_0091009 and the ENU-induced change was A12864G (protein NP_033135, resulting in E4242G). All of the data presented here were obtained after outcrossing >8 generations onto 129S1/Svlmj background.
Electron microscopy
Request a detailed protocolSoleus muscles of 2-month old mice were fixed with 3.5% glutaraldehyde in 0.1 M cacodylate buffer (pH 7.2) at 37°C. The central region of muscle was dissected to maximize the endplate regions, followed by a secondary post-fixation in 2% osmium tetroxide + 1.5% potassium ferrocyanide in 0.1 M cacodylate buffer for 1 hr at room temperature. Samples were dehydrated though an ethanol series (50, 70, 90, 95, and 100% ETOH) and embedded in Epon/Araldite resin polymerized overnight at 60°C. Ultrathin sections were cut, placed on copper mesh grids, and double contrasted with 2% aqueous uranyl acetate and Reynold's lead citrate. Grids were photographed using a FEI Technai G2 BioTwin transmission electron microscope.
Histochemistry
Request a detailed protocolCOX and NADH staining protocols are available at html://neuromuscular.wustl.edu/pathol/histol. Histostained samples were examined on a Zeiss LSM 510 META laser scanning microscope.
[Ca2+]i/PBFI measurements
Request a detailed protocolSoleus muscle was dissected from limb with tendons attached and bifurcated at the proximal tendon. Bifurcated muscle was affixed to glass bottom culture dishes (MatTeK) with Vetbond (3M, St. Paul, MN) and cleaned of debris and adipose tissue. Tyrode buffer: 140 mM NaCl, 5 mM KCl, 10 mM HEPES, 2 mM MgCl2, 2.5 mM CaCl2, pH 7.2, 290 mosm. The ratiometric cell-permeant calcium indicator Fura-2 AM (Molecular Probes, Eugene, OR, USA) was administered to the preparation for 45 min at 33°C then washed two times for 20 min at room temperature. Muscles were analyzed at 33°C using the 340/380 filters in a Zeiss Axio Observer Z1 with a Photometrics CoolSNAP HQ2 camera for wide-field imaging, then performing ratio intensity analysis. To deplete SR Ca2+ store, soleus fibers were treated with 10 μm thapsigargin for 2–4 min to induce Ca2+ release. The perfusion solution was then switched to 0.5 mM Mn2+ for 2–4 min to observe the extent of Mn2+ entry and establish the rate of SOCE. For all measurements of SOCE by Mn2+ quenching, the fluorescence signal was normalized using values determined by lysis of the cells with a solution containing 0 mM Ca2+/EGTA and 0.1% Triton X-100 at the end of the experiment. All experiments were conducted at ∼33°C.
[K+]i/PBFI measurements
Request a detailed protocolFor [K+]i measurements, soleus muscle was affixed to glass bottom culture dishes (MatTeK, Ashland, MA) with Vetbond (3 M). The ratiometric cell-permeant potassium indicator PBFI-AM (5 μM; Life Technologies, Grand Island, NY) together with 0.2% Pluronic F-127 for enhanced dye loading was loaded for 30 min in Ringer's solution (3 mM K+). After loading, muscles were washed for 30 min to remove excess dye and to allow de-esterification of the AM dye. Data were collected from 10 regions per muscle with three muscles per condition. Muscles were analyzed at 33°C using the 340/380 filters in a Zeiss Axio Observer Z1 with a Photometrics CoolSNAP HQ2 camera for wide-field imaging, then performing ratio intensity analysis. To calibrate intracellular potassium concentrations, PBFI ratio intensities were fitted to PBFI intensities due to the variable K+ solutions with the addition of 10 μM gramicidin in extracellular solutions and using the intensity of ∼10 fibers of interest per muscle in >3 muscles from each condition. Calibration solutions were prepared with appropriate volumes of a high [K+] solution with potassium gluconate.
Western analysis
Request a detailed protocolMuscle tissue from the limbs was dissected and washed in PBS and resuspended in RIPA buffer supplemented with Complete Mini Protease Inhibitor Cocktail (Roche Basel, Switzerland) and Phosphatase Inhibitor Cocktail Set II (EMD Millipore, Billerica, MA). Samples were sonicated for 1 min (15 s on, 15 s off, 25% power) and incubated at 4°C for 1 hr on a rocking platform. Protein was quantified with the Bio-Rad Protein Assay (BioRad, Hercules, CA). All samples were incubated at 37°C for 30 min prior to loading. Protein was separated on 4–12% Bis-Tris gels (Invitrogen) and transferred to Immobilon-FL PVDF membranes (Millipore). Membranes were blocked in 5% dry milk in TBST for 1 hr. Primary antibody incubations were performed overnight at 4°C while rocking. Antibodies were diluted in TBST supplemented with 5% wt/vol BSA (Sigma, St Louis, MO). Secondary antibody incubations were performed for 1 hr at room temperature in TBST supplemented with 5% dry milk. Western blots were imaged using the Odyssey Infrared Imaging System (Li-Cor, Lincoln, NE). Quantitation was performed using Odyssey v3.0 software (Li-Cor). Primary and secondary antibodies were used as follows: KIR6.1 (KATP6.1; Santa Cruz, goat, 1:200), KIR6.2 (KATP6.2; Abcam, goat, 1:1000), KIR2.1 (Abcam, rabbit, 1:1000), Goat anti-Rabbit IRDye 680 (Li-Cor, 1:10,000), Goat anti-Mouse IRDye 800CW (Li-Cor, 1:10,000), Donkey anti-Goat IRDye 680LT (Li-Cor, 1:30,000), Donkey anti-Mouse 800CW (Li-Cor, 1:10,000).
Membrane extract lysate
Request a detailed protocolMitochondrial and cytosolic protein fractions were isolated using differential centrifugation. Immediately after sacrifice, soleus skeletal muscles from RyR1+/+ and RyR1AG/+ mice were extracted, rinsed in saline solution, weighed, then cut into four fragments, and homogenized in 1:5 wt/vol ice-cold isolation buffer containing 0.21 M mannitol, 0.07 M sucrose, 0.005 M HEPES, 0.001 M EDTA, 0.2% fatty acid-free BSA, pH 7.4, using glass homogenizer. The homogenate was centrifuged at 600×g for 10 min at 4°C. The supernatant was then centrifuged at 12,000×g for 20 min. After the second spin, the supernatant (membrane, t-tubule and cytosol) was separated from the pellet (peroxisome, lysome, and mitochondria) and was stored at −80°C until used for Western blot assays.
ATP content
Request a detailed protocolSoleus skeletal muscles were assayed immediately after homogenization using buffer described in membrane extract lysate to determine ATP content with a luciferin–luciferase based bioluminescence assay. The methodology of the ATP determination kit is provided in the experimental protocol by Molecular Probes (A-22066, Eugene, OR, USA). In order to determine ATP content, freshly extracted homogenate was added to a cuvette containing reaction buffer, D-luciferin, luciferase, and DTT and placed in a Sirius luminometer v.2.2 (Berthold Detection Systems, Pforzheim, Germany). Known concentrations of ATP standards were used to establish a standard curve.
Quantitative RT-PCR
Request a detailed protocolRNA was extracted from muscles using Trizol followed by DNaseI digestion and clean-up (Qiagen RNAeasy Minikit, Venlo, Limburg) and reverse transcribed using random hexamer primers and SuperScript III Reverse Transcriptase (Invitrogen) and amplified using TaqMan Universal PCR Master Mix (Applied Biosystems). Quantitative PCR was performed on a Roche LightCycler 480 Real-Time PCR System. Calculations were performed by a relative standard curve method. Probes for target genes were from TaqMan Assay-on-Demand kits (Applied Biosystems). Samples were adjusted for total RNA content by GAPDH in mice on diets and for the human samples. Control human muscle RNA was purchased from Amsbio (R1234171-50) and Invitrogen (AM7982). The RNA samples were compared to a five sample human RNA skeletal muscle pool (R1234171-P). RNA samples of myopathies were generously provided by Telethon Biobank (Italy) and University of Colorado Neurology Department following University of Colorado COMIRB approval for human subject research.
Potassium diets and drug treatments
Request a detailed protocolDiets were based on studies examining hypertension in mice (Grimm et al., 2009). Diets were purchased through Harlan Laboratories, Inc. and are TD.10942 (0.6% K+) and TD.94121 (5.2% K+). These diets have similar ratios of three K+ sources. Following weaning (1 month of age), animals were placed on potassium diets ad libitum for 4 weeks followed by determination of muscle strength and molecular and histological examination. For enalapril and glibenclamide studies, following weaning, mice were placed on 0.6% K+ diet and given enalapril in drinking water (0.02 mg/ml) or glibenclamide via daily oral gavage (15 mg/kg/day) for 4 weeks.
Muscle fiber isolation
Request a detailed protocolSkeletal muscle fibers were isolated from the soleus muscles by a classical enzymatic dissociation process; muscles were incubated for 1 hr at 37°C in Tyrode's solution containing collagenase (2.0 mg/ml, Sigma, Type 1) for patch-clamp experiments or 1.5 hr at 37°C in Tyrode's solution containing collagenase (2.2 mg/ml, Sigma, Type 1) for calcium imaging and mitochondrial function experiments.
Patch-clamp experiments
Request a detailed protocolAfter enzyme treatment, muscles were rinsed with Tyrode's solution and kept in low calcium Ringer's solution at 4°C until analysis. Ringer's solution used during collection of isolated muscle fibers contained 145 mM NaCl, 5 mM KCl, 1 mM MgCl2, 0.5 mM CaCl2, 5 mM glucose, and 5 mM HEPES and was adjusted to pH 7.2. The patch-pipette solutions contained 150 mM KCl, 2 mM CaCl2, and 1 mM HEPES (pH 7.2). The bath solution contained 150 mM KCl, 5 mM EGTA, and 1 mM HEPES (pH 7.2).
Intact skeletal muscle fibers were separated from the muscle mass by gently triturating the muscle with a plastic 1000 μl pipette. Experiments were performed in inside-out configurations by using the standard patch-clamp technique. Initial pipette resistance was between 1.3–3.0 MΩ. Channel currents were recorded during voltage steps going from 0 mV of holding potential to −60 mV voltage membrane (Vm) immediately after excision, at 20–22°C, in the presence of KCl on both sides of membrane patches. Useful patches required minimal seal resistance of 1.1 GΩ. The mean currents were calculated by subtracting the baseline level from the open-channel level of each current trace. A minimum of three traces was collected for each seal. Digital averaging of traces was performed using CLAMPFIT (Molecular Devices, Sunnyvale, CA). ATP (5 mM) or glibenclamide (1 mM) was used to examine the pharmacological response of the channel.
Grip strength and Hanging Wire Task
Request a detailed protocolTo evaluate muscle weakness in vivo, we compared RyR1AG/+ and RyR1+/+ mice on the 5.2% K+ or 0.6% K+ diet without or with glibenclamide or enalapril using grip strength tests (Costa et al., 2010) and wire hanging task (Ogura et al., 2001). Similar tests have been used to evaluate muscle weakness in CCD mice (Loy et al., 2011). Performance in Hanging Wire Task was scored on a 0 to 5 scale according to Loy et al. (2011): 0, immediately fell off the bar; 1, hung onto bar with two forepaws; 2, hung onto bar with two forepaws and attempted to climb onto the bar; 3, hung onto the bar with two forepaws and one or both hind paws; 4, hung onto the bar with all four paws and tail wrapped around the bar; 5, hung onto the bar with all four paws and tail wrapped around the bar and escaped onto one of the supports. Body weight was measured before and after therapies with no significant difference in weight (data not shown).
Malignant hyperthermia and isoflurane sensitivity
Request a detailed protocolMalignant hyperthermia test was performed by placing Ryr1+/+ and Ryr1AG/+ mice in a 41°C humidified incubator for 30 min. To test for sensitivity to halogenated anesthetics, stage 3 anesthetic isoflurane (∼5.5 × 10−5 ml/cm3) was administered through a nasal cone to Ryr1AG/+ mice at a maximum of 30 min exposure.
Mitochondrial functionality
Request a detailed protocolIsolated soleus muscle fibers from 2-month old Ryr1+/+ and Ryr1AG/+ were examined for mitochondrial function. MitoTracker Deep Red FM (Invitrogen) was added to all fibers to identify the mitochondria and Powerload (Invitrogen) was added to calcium indicators for 20 min. To examine mitochondria calcium changes in intensity, Rhod2-AM (Invitrogen) was added to the fibers in Normal Ringer's solution for 30 min at room temperature and then the cultures were washed with normal Ringers. To examine mitochondrial membrane potential, TMRE (Invitrogen) with saponin (Sigma) were added at the manufacturer's recommended dose for 30 min at room temperature. FCCP (carbonyl cyanide 4-(trifluoromethoxy)phenylhydrazone; ABCAM, Cambridge, England) was added at the end of the experiment to eliminate the mitochondrial membrane potential and the value was used to normalized to the experimental fluorescent TMRE value. To examine superoxides, MitoSOX (Invitrogen) indicator was added at the manufacturer's recommended dose for 15 min at 37°C. Antimycin A (Sigma) was used as a positive control at the end of the experiment to normalize to the experimental signal. We placed the fiber cultures into a Zeiss Environmental incubation system (5% humidity, 95% O2) at 22°C. Images of mitochondrial regions (3 per fiber and 5 fibers per muscle in 3 muscles) were taken on a Zeiss LSM510 laser-scanning microscope of 1000 μm2 fields. Scans were taken every 2 min. All data were normalized to baseline per mitochondrial region to give a Δf/f value (Image J).
Statistical analysis
Request a detailed protocolThe unpaired two-tailed Student's t test was used to compare means for statistical differences. Data in the manuscript are represented as mean ± SEM unless otherwise indicated. p < 0.05 was considered significant.
Serum analysis
Request a detailed protocolNormal methods to collect serum do not provide an accurate representation of interstitial (extracellular) potassium levels (Mazzaccara et al., 2008). However, to determine whether any overt discrepancies of serum potassium, sodium, and chloride can be detected, serum was collected from 2-month and 6-month old wild-type and Ryr1AG/+ mice. Analysis was performed using a Starlyte ISE Analyzer (Alfa Wassermann, West Caldwell, NJ).
Study approval
Request a detailed protocolAll experiments were conducted in accordance with the protocols described in the Guide for the Care and Use of Laboratory Animals (NIH. Revised, 2011) and we received University of Colorado COMIRB approval for human subject research.
References
-
Glibenclamide increases post-fatigue tension in slow skeletal muscle fibers of the chickenJournal of Comparative Physiology B, Biochemical, Systemic, and Environmental Physiology 181:403–412.https://doi.org/10.1007/s00360-010-0527-1
-
Plasma membrane KATP channel-mediated cardioprotection involves posthypoxic reductions in calcium overload and contractile dysfunction: mechanistic insights into cardioplegiaFASEB Journal 19:980–982.
-
The KATP channel Kir6.2 subunit content is higher in glycolytic than oxidative skeletal muscle fibersAmerican Journal of Physiology Regulatory, Integrative and Comparative Physiology 301:R916–R925.https://doi.org/10.1152/ajpregu.00663.2010
-
Energy turnover for Ca2+ cycling in skeletal muscleJournal of Muscle Research and Cell Motility 28:259–274.https://doi.org/10.1007/s10974-007-9116-7
-
Xenon does not induce contracture in human malignant hyperthermia muscleBritish Journal of Anaesthesia 85:712–716.https://doi.org/10.1093/bja/85.5.712
-
Characterization and temporal development of cores in a mouse model of malignant hyperthermiaProceedings of the National Academy of Sciences of USA 106:21996–22001.https://doi.org/10.1073/pnas.0911496106
-
Cardiomyocyte ATP production, metabolic flexibility, and survival require calcium flux through cardiac ryanodine receptors in vivoThe Journal of Biological Chemistry 288:18975–18986.https://doi.org/10.1074/jbc.M112.427062
-
Dyspedic mouse skeletal muscle expresses major elements of the triadic junction but lacks detectable ryanodine receptor protein and functionThe Journal of Biological Chemistry 272:7360–7367.https://doi.org/10.1074/jbc.272.11.7360
-
Sarcoplasmic reticulum Ca(2+) release by 4-chloro-m-cresol (4-CmC) in intact and chemically skinned ferret cardiac ventricular fibersThe Journal of Pharmacology and Experimental Therapeutics 290:578–586.
-
Sodium and potassium balance depends on alphaENaC expression in connecting tubuleJournal of the American Society of Nephrology 21:1942–1951.https://doi.org/10.1681/ASN.2009101077
-
Regulation of active Na+-K+ transport in skeletal musclePhysiological Reviews 66:542–580.
-
Excitation-induced exchange of Na+, K+, and Cl- in rat EDL muscle in vitro and in vivo: physiology and pathophysiologyThe Journal of General Physiology 141:179–192.https://doi.org/10.1085/jgp.201210892
-
Quantification of Na+,K+ pumps and their transport rate in skeletal muscle: functional significanceThe Journal of General Physiology 142:327–345.https://doi.org/10.1085/jgp.201310980
-
Significance of cation transport in control of energy metabolism and thermogenesisPhysiological Reviews 71:733–774.
-
Identification of a key determinant of ryanodine receptor type 1 required for activation by 4-chloro-m-cresolThe Journal of Biological Chemistry 278:28727–28735.https://doi.org/10.1074/jbc.M303821200
-
Relationships between resting conductances, excitability, and t-system ionic homeostasis in skeletal muscleThe Journal of General Physiology 138:95–116.https://doi.org/10.1085/jgp.201110617
-
KATP channels depress force by reducing action potential amplitude in mouse EDL and soleus muscleAmerican Journal of Physiology Cell Physiology 285:C1464–C1474.https://doi.org/10.1152/ajpcell.00278.2003
-
Blocking ATP-sensitive K+ channel during metabolic inhibition impairs muscle contractilityThe American Journal of Physiology 272:C1936–C1946.
-
Mitochondrial calcium as a key regulator of mitochondrial ATP production in mammalian cellsBiochimica Et Biophysica Acta 1787:1324–1333.https://doi.org/10.1016/j.bbabio.2009.01.019
-
Hypertension of Kcnmb1-/- is linked to deficient K secretion and aldosteronismProceedings of the National Academy of Sciences of USA 106:11800–11805.https://doi.org/10.1073/pnas.0904635106
-
BookWHO Issues New Guidance on Dietary Salt and PotassiumHartl G, editors. World Health Organization.
-
4-Chloro-m-cresol, a potent and specific activator of the skeletal muscle ryanodine receptorBiochimica Et Biophysica Acta 1289:31–40.https://doi.org/10.1016/0304-4165(95)00131-X
-
Muscle enthalpy production and its relationship to actomyosin ATPaseAnnual Review of Physiology 49:673–690.https://doi.org/10.1146/annurev.ph.49.030187.003325
-
Core myopathiesSeminars in Pediatric Neurology 18:239–249.https://doi.org/10.1016/j.spen.2011.10.005
-
Phosphocreatine and ATP content in human single muscle fibres before and after maximum dynamic exercisePflügers Archiv : European Journal of Physiology 442:467–474.https://doi.org/10.1007/s004240100552
-
Metabolism changes in single human fibres during brief maximal exerciseExperimental Physiology 86:411–415.https://doi.org/10.1113/eph8602223
-
A phenotype-based screen for embryonic lethal mutations in the mouseProceedings of the National Academy of Sciences of USA 95:7485–7490.https://doi.org/10.1073/pnas.95.13.7485
-
An integrative, in situ approach to examining K+ flux in resting skeletal muscleCanadian Journal of Physiology and Pharmacology 79:996–1006.https://doi.org/10.1139/cjpp-79-12-996
-
Molecular cloning and characterization of the ryanodine receptor/junctional channel complex cDNA from skeletal muscle sarcoplasmic reticulumProceedings of the National Academy of Sciences of USA 86:8683–8687.https://doi.org/10.1073/pnas.86.22.8683
-
Muscle K+, Na+, and Cl disturbances and Na+-K+ pump inactivation: implications for fatigueJournal of Applied Physiology 104:288–295.https://doi.org/10.1152/japplphysiol.01037.2007
-
Identification of susceptibility to malignant hyperpyrexiaBritish Medical Journal 2:245–247.https://doi.org/10.1136/bmj.2.5913.245
-
Molecular insights into excitation-contraction couplingCold Spring Harbor Symposia on Quantitative Biology 55:1–7.https://doi.org/10.1101/SQB.1990.055.01.003
-
Molecular cloning of cDNA encoding the Ca2+ release channel (ryanodine receptor) of rabbit cardiac muscle sarcoplasmic reticulumThe Journal of Biological Chemistry 265:13472–13483.
-
Ca2+ marks: miniature calcium signals in single mitochondria driven by ryanodine receptorsProceedings of the National Academy of Sciences of USA 99:2380–2385.https://doi.org/10.1073/pnas.032423699
-
Regulation of ClC-1 and KATP channels in action potential-firing fast-twitch muscle fibersThe Journal of General Physiology 134:309–322.https://doi.org/10.1085/jgp.200910290
-
Phosphocreatine content in single fibers of human muscle after sustained submaximal exerciseThe American Journal of Physiology 273:C172–C178.
-
The efficiency of muscle contractionProgress in Biophysics and Molecular Biology 88:1–58.https://doi.org/10.1016/j.pbiomolbio.2003.11.014
-
Congenital muscle disorders with cores: the ryanodine receptor calcium channel paradigmCurrent Opinion in Pharmacology 8:319–326.https://doi.org/10.1016/j.coph.2008.01.005
-
Hybrid assemblies of ATP-sensitive K+ channels determine their muscle-type-dependent biophysical and pharmacological propertiesProceedings of the National Academy of Sciences of USA 103:1118–1123.https://doi.org/10.1073/pnas.0505974103
-
Impairment of skeletal muscle adenosine triphosphate-sensitive K+ channels in patients with hypokalemic periodic paralysisThe Journal of Clinical Investigation 103:675–682.https://doi.org/10.1172/JCI4552
-
Selective loading of Rhod 2 into mitochondria shows mitochondrial Ca2+ transients during the contractile cycle in adult rabbit cardiac myocytesBiochemical and Biophysical Research Communications 236:738–742.https://doi.org/10.1006/bbrc.1997.7042
-
Potassium disturbances as a cause of metabolic neuromyopathyIntensive Care Medicine 13:208–210.https://doi.org/10.1007/BF00254706
-
5-amino-imidazole carboxamide riboside acutely potentiates glucose-stimulated insulin secretion from mouse pancreatic islets by KATP channel-dependent and -independent pathwaysBiochemical and Biophysical Research Communications 330:1073–1079.https://doi.org/10.1016/j.bbrc.2005.03.093
-
Detection of calcium sparks in intact and permeabilized skeletal muscle fibersMethods in Molecular Biology 798:395–410.https://doi.org/10.1007/978-1-61779-343-1_23
-
RYR1 mutations are a common cause of congenital myopathies with central nucleiAnnals of Neurology 68:717–726.https://doi.org/10.1002/ana.22119
-
Elevated resting [Ca(2+)](i) in myotubes expressing malignant hyperthermia RyR1 cDNAs is partially restored by modulation of passive calcium leak from the SRAmerican Journal of Physiology Cell Physiology 292:C1591–C1598.https://doi.org/10.1152/ajpcell.00133.2006
-
Molecular cloning of cDNA encoding human and rabbit forms of the Ca2+ release channel (ryanodine receptor) of skeletal muscle sarcoplasmic reticulumThe Journal of Biological Chemistry 265:2244–2256.
-
Chlorocresol: an activator of ryanodine receptor-mediated Ca2+ releaseMolecular Pharmacology 44:1192–1201.
-
Ca2+ dysregulation in Ryr1(I4895T/wt) mice causes congenital myopathy with progressive formation of minicores, cores, and nemaline rodsProceedings of the National Academy of Sciences of USA 106:21813–21818.https://doi.org/10.1073/pnas.0912126106
Article and author information
Author details
Funding
Howard Hughes Medical Institute
- M Gartz Hanson
- Jonathan J Wilde
- Angela D Minic
- Lee Niswander
Muscular Dystrophy Association (MDA-69338)
- M Gartz Hanson
National Institute of Neurological Disorders and Stroke (F32-NS059267)
- M Gartz Hanson
The funders had no role in study design, data collection and interpretation, or the decision to submit the work for publication.
Acknowledgements
We thank Lori Bulwith, Radu Moldovan, and Chris Rivald for technical assistance, Alberto Costa for providing the apparatus to measure muscle strength, and Emily Bates, William Betz, and John Caldwell for comments on the manuscript. We thank Telethon Genetic Network of Biobanks and UC Denver Neurology Department for the use of RNA samples of muscle biopsies from CCD patients. Angela Ribera supplied physiology equipment (NIH R01 NS25217). This work was supported by the Department of Pediatrics and by the Neuroscience Program NS 48154. MGH was supported by postdoctoral fellowships from MDA69338 and NIH F32 NS059267. LN was an investigator of the Howard Hughes Medical Institute. The authors have declared that no conflict of interest exists.
Ethics
Human subjects: Ethical approval was obtained through University of Colorado COMIRB approval for human subject research (protocol number 12-1504). All samples were provided through an exempt IRB which stated that we were disallowed patient information that could be used as an identifier.
Animal experimentation: All experiments were conducted in accordance with the protocols described in the Guide for the Care and Use of Laboratory Animals (NIH. Revised 2011). All of the animals were handled according to approved institutional animal care and use committee (IACUC) protocol (B-69913(10)1D) of the University of Colorado Denver.
Copyright
© 2015, Hanson et al.
This article is distributed under the terms of the Creative Commons Attribution License, which permits unrestricted use and redistribution provided that the original author and source are credited.
Metrics
-
- 1,660
- views
-
- 170
- downloads
-
- 9
- citations
Views, downloads and citations are aggregated across all versions of this paper published by eLife.
Citations by DOI
-
- 9
- citations for umbrella DOI https://doi.org/10.7554/eLife.02923