Gap junctions composed of connexins 41.8 and 39.4 are essential for colour pattern formation in zebrafish
Abstract
Interactions between all three pigment cell types are required to form the stripe pattern of adult zebrafish (Danio rerio), but their molecular nature is poorly understood. Mutations in leopard (leo), encoding Connexin 41.8 (Cx41.8), a gap junction subunit, cause a phenotypic series of spotted patterns. A new dominant allele, leotK3, leads to a complete loss of the pattern, suggesting a dominant negative impact on another component of gap junctions. In a genetic screen, we identified this component as Cx39.4 (luchs). Loss-of-function alleles demonstrate that luchs is required for stripe formation in zebrafish; however, the fins are almost not affected. Double mutants and chimeras, which show that leo and luchs are only required in xanthophores and melanophores, but not in iridophores, suggest that both connexins form heteromeric gap junctions. The phenotypes indicate that these promote homotypic interactions between melanophores and xanthophores, respectively, and those cells instruct the patterning of the iridophores.
https://doi.org/10.7554/eLife.05125.001eLife digest
The colour patterns that mark an animal's skin, hair, or feathers—called the pigmentation pattern—can be very important for its survival and fitness, helping it to hide from predators or to attract a mate. As a result, there is considerable interest in understanding how genes, proteins, and cells work together to produce the many different pigmentation patterns that exist in the animal world.
Adult zebrafish have a characteristic pigmentation pattern of horizontal dark and light stripes on their bodies and fins. There are three types of pigment cell that create this pattern. Xanthophores and iridophores are found all over the body, and the dark stripes also contain melanophore cells. The silvery, reflective iridophores are the first of the cells to populate the skin, giving rise to the first light stripe. They then form a dense network of cells that breaks up to form the darker stripes. However, iridophores are not required to form stripes in the fins, suggesting that patterning occurs differently in the fins and the body.
Mutations to a gene called leopard, or leo for short, cause spots to form on the skin of the zebrafish in place of the usual stripes. This gene encodes a member of the connexin family of proteins, which form channels in the membranes that surround cells. These channels—known as gap junctions—allow neighbouring cells to communicate with each other. Each gap junction is made up of two half channels, with one half coming from each neighbouring cells. If the two half channels are identical, the gap junction is known as ‘homomeric’; ‘heteromeric’ gap junctions are made from two different half channels, each consisting of a different connexin protein. The connexin encoded by leo is required for both types of gap junction to form between melanophores and xanthophores.
Irion et al. discovered a new mutation to the leo gene that completely disrupts the patterning of the zebrafish. A technique called a genetic screen revealed that the same patterning defects are also seen in the body of zebrafish with mutations to another gene called luchs, which encodes a different connexin protein to the one produced by leo. However, the fins of zebrafish with mutant versions of luchs remain striped.
The findings of Irion et al. suggest that heteromeric gap junctions formed from the connexins produced by leo and luchs are important for xanthophores and melanophores to communicate with each other and so form the stripy patterning seen on the body of the zebrafish. The signals transmitted through the gap junctions may also make the iridophores adopt the looser arrangement that is required for the dark stripes to form. As a next step, it will be important to identify the signals that pass through these gap junctions that allow the cells to communicate with their neighbours and establish the pigmentation pattern.
https://doi.org/10.7554/eLife.05125.002Introduction
Adult zebrafish (Danio rerio) display a characteristic pattern of horizontal dark and light stripes on their bodies as well as on their anal fins and tailfins (Figure 1A). Three types of pigment cells (chromatophores) are required to create this pattern. In the dark stripes of the trunk, a net of loose or blue iridophores and pale, stellate xanthophores cover the melanophores, the light stripes are composed of dense silvery iridophores covered by compact orange xanthophores (Hirata et al., 2003, 2005; Frohnhofer et al., 2013; Mahalwar et al., 2014; Singh et al., 2014).
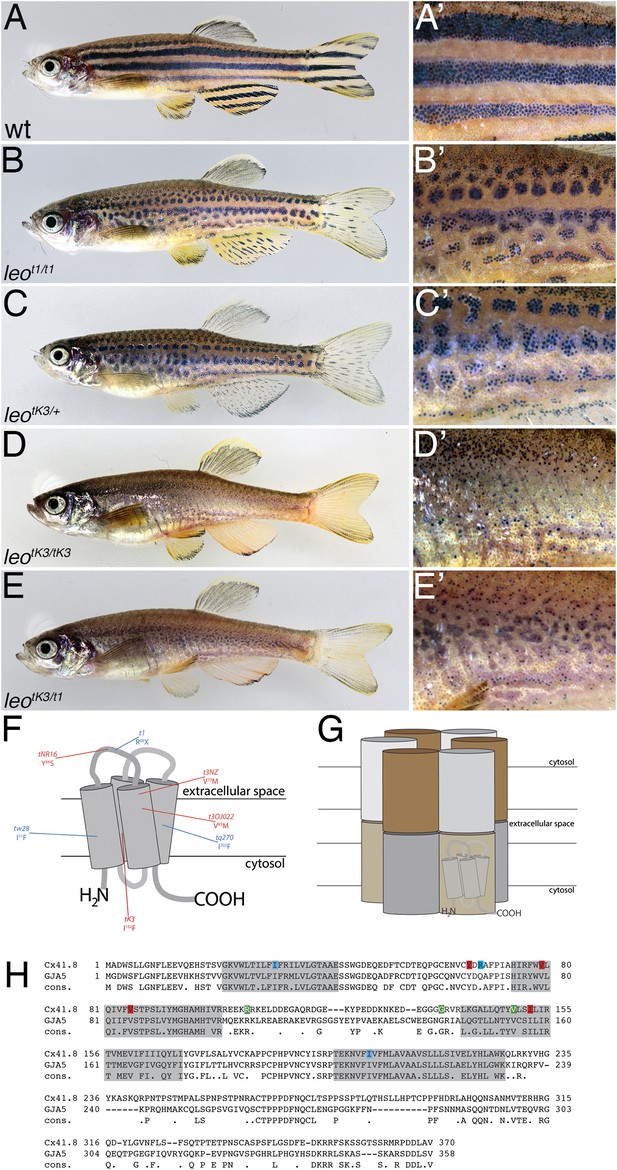
The leo mutant phenotype.
Wild-type zebrafish (A) show a pattern of dark and light stripes on the body and on anal- and tail-fins. At higher magnification (A′), dark melanophores in the stripe regions and orange xanthophores in the light stripe regions are discernible. In mutants homozygous for leot1 (B) and heterozygous for leotK3 (C), the stripes are dissolved into spots. Clusters of melanophores are still visible (B′ and C′). Fish homozygous for leotK3 (D) or trans-heterozygous for leotK3 over leot1 (E) show an identical phenotype of a completely dissolved pattern. Individual melanophores that hardly cluster together are still present, mostly associated with blue iridophores (D′ and E′). In (F) a cartoon of Connexin 41.8 is depicted showing the positions of the leo mutations. Gap junctions are composed of two hemi-channels in adjacent cells. Each hemi-channel is made of six connexin subunits, they can be identical (homomeric) or different (heteromeric). In (G) a heteromeric/heterotypic gap junction is schematically shown. An alignment of the amino acid sequence from zebrafish Cx41.8 with its human orthologue, GJA5, is shown in (H). The transmembrane regions are shaded in grey. Newly identified point mutations in Connexin 41.8 are highlighted in red: leotNR16: Y66S; leotNZ: V79M; leot3OJ022: V85M; leotK3: I152F. Already known alleles are highlighted in blue: leotw28: I31F; leot1: R68X; leotq270: I202F. Polymorphisms found in sequences from wild-type fish are highlighted in green: 106 R/K, 136 G/R; 149 V/I.
The adult pigmentation pattern is formed during metamorphosis, a period between approximately 3 and 6 weeks of development. At the onset of metamorphosis, iridophores appear in the skin at the region of the horizontal myoseptum that provides a morphological pre-pattern (Frohnhofer et al., 2013). They proliferate and spread as densely connected cells to form the first light stripe. While spreading further ventrally and dorsally, into the regions where the first two dark stripes will form, they change their appearance and become more loose, then they aggregate again at a distance to form the next light stripes (Singh et al., 2013). Larval xanthophores, covering the flank of the fish, start to proliferate and re-organize into densely packed compact cells above the dense iridophores of the light stripe and into more loosely organized, stellate cells in the dark stripe regions (Mahalwar et al., 2014). Melanoblasts migrate along spinal nerves into the skin in the presumptive stripe regions where they finally differentiate and expand to fill the space (Budi et al., 2011; Dooley et al., 2013; McMenamin et al., 2014; Singh et al., 2014). In the fins, stripe formation does not require iridophores, suggesting that the patterning mechanisms in the body and fins are different.
A number of mutants are known in which the pattern is not formed normally. In one class of them, one type of pigment cell is absent; in mutants for nacre/mitfA melanophores are missing (Lister et al., 1999), mutations in pfeffer/csf1rA, lead to the lack of xanthophores (Odenthal et al., 1996; Parichy et al., 2000b), and in shady/ltk, rose/ednrb1b, and transparent/mpv17 mutants iridophores are absent or strongly reduced (Parichy et al., 2000a; Lopes et al., 2008; Krauss et al., 2013). In all these cases, the remaining two types of chromatophores form an irregular residual striped pattern. These genes are autonomously required in the respective cell types indicating that interactions among all three chromatophore types are necessary to generate the striped pattern on the trunk of the fish (Maderspacher and Nusslein-Volhard, 2003; Parichy and Turner, 2003; Frohnhofer et al., 2013; Krauss et al., 2014). Based on the analysis of these mutants and on ablation experiments, several attractive and repulsive signals acting over long or short ranges between the chromatophores have been postulated (Maderspacher and Nusslein-Volhard, 2003; Yamaguchi et al., 2007; Nakamasu et al., 2009; Frohnhofer et al., 2013; Patterson and Parichy, 2013; Krauss et al., 2014).
In another class of mutants, an abnormal pattern is formed with all three chromatophore types present (Haffter et al., 1996); in these animals, the communication between the cells might be affected. The genes identified in this group encode integral membrane proteins, for example, obelix/Kir7.1, a rectifying potassium channel (Iwashita et al., 2006), seurat/Igsf11, a cell-adhesion molecule of the immunoglobulin superfamily (Eom et al., 2012), or dali/Tetraspanin 3c, a transmembrane-scaffolding protein (Inoue et al., 2012). The best-known example for this class of mutants is leopard (leo), where the stripes are wavy or broken up into a series of dark spots (Figure 1B). The original mutant has been regarded as a separate Danio species (Kirschbaum, 1975; Kirschbaum, 1977; Frankel, 1979). Subsequently several dominant alleles were identified in Danio rerio (Haffter et al., 1996), and it has been shown that the leo phenotype is caused by a mutation in connexin 41.8 (Watanabe et al., 2006), which codes for a subunit of gap junctions (gap junction protein α5, GJA5).
Gap junctions are intercellular channels that allow the passage of small molecules and ions between neighbouring cells, and thus are responsible for their chemical and electrical coupling (Kar et al., 2012). They are formed by the juxtaposition of two hemi-channels (connexons), composed of six connexin subunits, in adjacent cells (Unwin and Zampighi, 1980). Connexins are integral membrane proteins with four transmembrane domains, two extracellular loops, one intracellular loop, and intracellular N- and C-termini (Milks et al., 1988) (Figure 1F,G). Hemi-channels can be composed of different subunits; the resulting gap junctions are homotypic, if identical connexons from neighbouring cells pair, or heterotypic, if different hemi-channels come together. In addition to the subunit composition, gap junction conductivity is regulated by a number of different factors, for example, by the intracellular levels of Ca2+, by polyamines, by the membrane potential, or by phosphorylation (Thévenin et al., 2013).
It has been reported that the function of the leo gene is required in two of the chromatophore types, the melanophores and the xanthophores, for homotypic and heterotypic cellular interactions (Maderspacher and Nusslein-Volhard, 2003). Wild-type and mutant forms of Cx41.8 artificially expressed in melanophores can lead to different variations of the stripe pattern (Watanabe and Kondo, 2012). This could indicate that gap junctions are responsible for some of the short range signals postulated to occur within and between these two chromatophore types (Parichy and Turner, 2003; Inaba et al., 2012; Frohnhofer et al., 2013).
Three alleles of leo have been described so far. The original allele, leot1, is recessive; it has a premature stop codon at position 68 of the coding sequence and is most likely a functional null-allele. Two dominant alleles, leotq270 and leotw28, carry missense mutations; they lead to a stronger phenotype (Haffter et al., 1996; Watanabe et al., 2006). The dominance of these alleles led to the suggestion that heterotypic as well as homotypic connexons containing Cx41.8 could be involved in pigment patterning, postulating the existence of other connexin partner(s) in the potential heterotypic channels (Watanabe et al., 2006).
In this study, we identified several additional alleles of leo. All of them are dominant, and the strongest of them, leotK3, leads to a complete loss of the pigmentation pattern in homozygous carriers; the number of melanophores is reduced, and they appear as small groups or individual cells in the skin, embedded in an expanded light region of dense iridophores covered by xanthophores. In a genetic screen, we found two dominant alleles of a gene we named luchs (luc) as enhancers of the leo loss-of-function phenotype. We show that the luc gene codes for another connexin, Cx39.4. A loss-of-function allele for luc displays a phenotype similar to leot1; however, the fins are not affected. Further we show that both genes, leo and luc, are required in the trunk in xanthophores and in melanophores, but not in iridophores. Our results suggest that Cx41.8 and Cx39.4 form heteromeric gap junction channels in the plasma membranes of melanophores and xanthophores. In the complete absence of the channels, iridophores take over and almost fully fill the space normally occupied by alternating light and dark stripes, whereas melanophores are suppressed. This suggests that the heteromeric gap junctions function in the communication between xanthophores and melanophores and potentially in the transduction of signals to the dense iridophores to induce the transition into the loose shape required for dark stripe formation.
Results
New alleles of leo
In a number of experiments designed to find mutants with adult phenotypes, we identified four additional alleles of leo. All of them are dominant. Three of our new alleles result in a phenotypic series of patterns from undulating stripes to breaking up of the dark stripe regions into small spots. As in wild-type (Figure 1A–A′), the dark stripe regions of melanophores are covered with loose blue iridophores. The light stripe regions composed of dense iridophores covered by compact xanthophores are expanded and ingress into the stripe regions. Depending on the genotypes, they display varying strengths, similar to the previously described dominant alleles leotw28 and leotq270 (Haffter et al., 1996; Watanabe et al., 2006). However, one allele, leotK3, is considerably stronger. In heterozygous fish, it leads to an intermediate phenotype similar to the one seen in homozygotes for the loss-of-function allele leot1. Fish homozygous for leotK3, or trans-heterozygous for leotK3 and leot1, show a much stronger phenotype with a loss of any striped arrangement (Figure 1B–E). Instead of dark and light stripe regions, they display variable numbers of small groups of melanophores in islands of blue iridophores, or even single melanophores distributed in an almost even background of dense iridophores covered by xanthophores characteristic for the light stripe regions (Figure 1D′,E′). The stripes in the anal and caudal fins are also affected in leo mutants, weak phenotypes show some short residual melanophore stripes, in homozygous leotK3 animals the pattern is completely lost and only few melanophores are present at the margins of the fins.
The newly identified leo alleles carry mis-sense mutations in the coding sequence for Connexin 41.8. The affected amino acid residues are at highly conserved positions in the N-terminal half of the protein (Figure 1F,H); the mutation in leotK3, Ile152Phe, lies within the third transmembrane domain at a position where all connexins invariably have a small hydrophobic residue.
Dominant mutations in luc enhance the phenotype of leot1
The fact that the mis-sense mutation in leotK3 leads to a much stronger phenotype than the one seen in fish homozygous for the loss-of-function allele leot1 argues for a dominant negative effect of the mutant protein on an additional component involved in pigment patterning. To identify this component, we performed a genetic screen for dominant mutations that enhance the phenotype of leot1. We mutagenized males homozygous for leot1 with ENU and crossed them to homozygous mutant females. In their offspring, we screened for fish with a stronger phenotype, similar to the one observed in leotK3 mutants. Among 4469 F1 fish, we found three individuals with a strongly enhanced phenotype. One of them turned out to be an allele of obelix (Haffter et al., 1996), which has been shown before to enhance the leo phenotype (Maderspacher and Nusslein-Volhard, 2003). The other two mutants, tXA9 and tXG1 (Figure 2B,E), carry alleles of the same gene, which we named luchs (luc) after the German name for lynx. Both have almost identical phenotypes, heterozygous fish show wavy stripes with some gaps, in homozygotes and in trans-heterozygotes, the stripes are completely dissolved into very small spots and individual melanophores (Figure 2C,D,F,G). Mutants of luc display patterns that are not significantly different from those of the leo phenotypic series, however, the fins are much less affected in our new mutants. To identify the responsible mutations, we used a candidate approach and sequenced the coding sequences of several connexin genes. For both alleles we identified a mis-sense mutation in the gene encoding Connexin 39.4 (cx39.4). In luctXA9 an A to C transversion leads to an amino acid exchange T29P, in luctXG1 a G to T transversion results in W47L, both residues are highly conserved and lie within the first transmembrane domain and the first extracellular loop, respectively (Figure 3A).
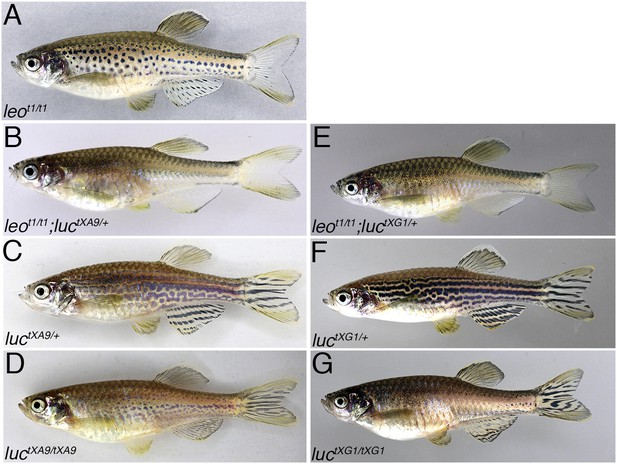
Dominant enhancers of the leot1 phenotype.
The spotted pattern of fish homozygous for leot1 (A) is lost when they carry an additional mutant allele for luctXA9 (B) or luctXG1 (E). Fish heterozygous for luctXA9 (C) and luctXG1 (F) have undulating and disrupted stripes on their flanks. In mutants homozygous for luctXA9 (D) or luctXG1 (G) the pattern is almost as strongly disrupted as in leotK3 mutants (compare to Figure 1D).
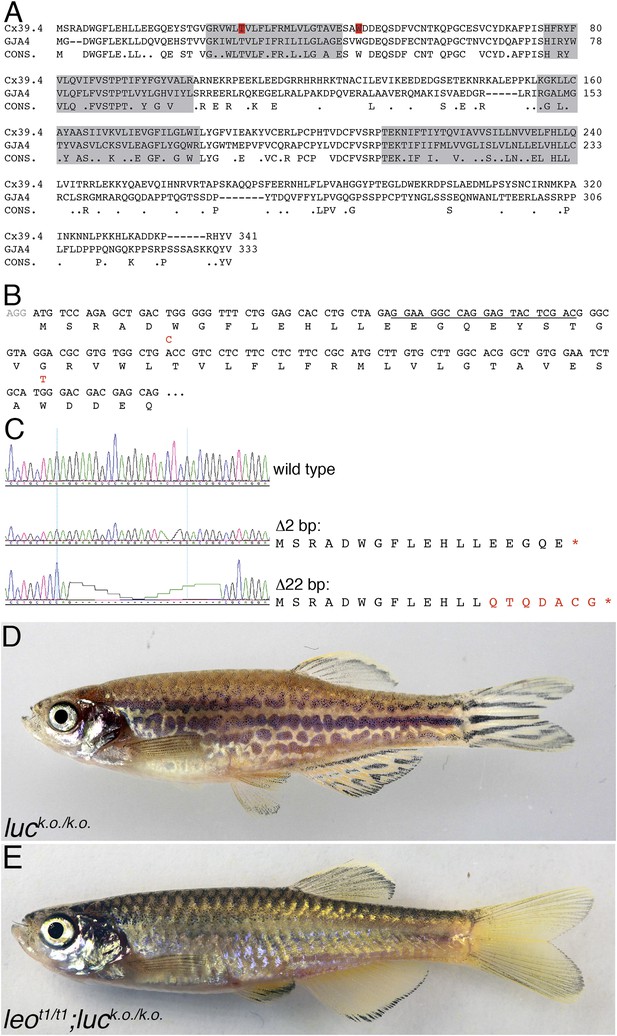
luc loss-of-function leads to patterning defects.
A comparison of the amino acid sequences of Cx39.4 of zebrafish, encoded by the luc gene, and human Cx37 (GJA4) is shown in (A). The transmembrane domains are shaded in grey, the residues mutated in luctXA9 (T29 P) and luctXG1 (W47 L) are highlighted in red. Note the extension of the N-terminal cytoplasmic domain of Cx39.4 by two amino acid residues. In (B) the beginning of the coding sequence for Cx39.4 is shown, the mutations in luctXA9 and luctXG1 are indicated in red above the sequence; the CRISPR target site is underlined. The sequence traces of wild-type fish and two fish homozygous for deletions of 2 and 22 bp are shown in (C). The predicted amino acid sequences for these mutants are indicated. In (D) a fish homozygous for the 2 bp deletion is shown, the striped pattern on the flank of the fish is disrupted and partly dissolved into spots, the fins are almost normal. Double mutants for leot1 and luc loss-of-function (E) have only very few melanophores on the flank and show an almost uniform pattern of iridophores and xanthophores.
luc function in pigment patterning
To investigate the role of Cx39.4 in pigment pattern formation in zebrafish, we created loss-of-function mutations in the luc gene using the CRISPR/Cas9 system (Hwang et al., 2013). We targeted the 5′ region of the coding sequence (Figure 3B) and found a high incidence of small deletions leading to frame shift mutations. Most often we found a 3 bp (CTC) deletion accompanied by a 1 bp (G) insertion resulting in a premature stop codon and leading to a truncation of the translated protein after 18 amino acids. We also frequently found a 22 bp deletion resulting in a truncated protein of only 13 amino acids followed by seven unrelated residues (Figure 3C). Fish homozygous or trans-heterozygous for these knock-out alleles (luck.o.) develop an irregular pattern with interrupted stripes and spots very similar to leo mutants. Again, like in the case of the dominant alleles, the patterning of the fins is less affected in the mutants and they show an almost normal arrangement of stripes (Figure 3D). We also reverted the phenotype of heterozygous luctXA9 mutants to wild-type by inducing loss-of-function mutations with the CRISPR/Cas system on the chromosome carrying the dominant allele. This confirms that the luc alleles carry mutations in the Cx39.4 gene. Interestingly, double mutants homozygous for loss-of-function alleles of leo and luc display a phenotype which is indistinguishable from that of homozygous mutants of the dominant leotK3 allele and slightly stronger than that of the homozygous dominant alleles of luc (Figure 3E). This indicates that the dominant negative effect observed in leotK3 is mediated through connexin 39.4, and that in leotK3 both the leo and luc functions are abolished. Our results are most easily explained if the two connexins form heteromeric gap junctions.
The development of the leo and luc phenotypes
To establish the time point when the phenotype of the leo and luc mutants becomes first apparent, we followed wild-type and mutant fish during the course of metamorphosis. The first signs of the phenotype become visible at stage PR (8.6 mm SSL). When in wild-type, melanophores gather immediately dorsally and ventrally to the dense iridophores of the first light stripe, they stay more dispersed in the mutants (Figure 4A–E). Dense iridophores covered with xanthophores disperse in an irregular manner in the mutants to form a wider first light stripe, branching into the dark stripe region and suppressing melanophores dorsally and ventrally to form irregular patches (Figure 4F–J). At stage J++, when in wild-type three dark and three light stripes are formed, in the luc and leo loss-of-function mutants the spotted pattern is already clearly apparent (Figure 4L,M) and the dominant mutants show fields of dense iridophores and xanthophores with few, scattered melanophores (Figure 4N,O). This analysis reveals that the leo;luc function is required during development and the mutant patterns are not caused by pattern instability.
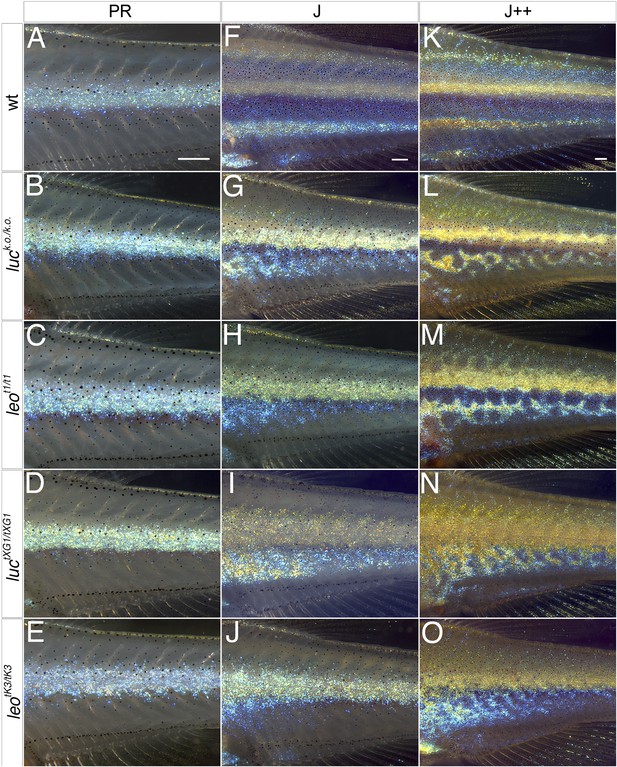
The leo and luc phenotypes arise during metamorphosis.
In wild-type dense iridophores of consecutively formed interstripes become visible during stages PR (A), J (F), and J++ (K). Melanophores appear in the stripe regions. In luc loss-of-function (B, G, L), leot1 (C, H, M), luctXG1 (D, I, N), and leotK3 mutants (E, J, O) iridophores of the first interstripe form normally at stage PR (B–E), however, the melanophores ventral to the first interstripe are more scattered. In the mutants at stage J (G–J) the second interstripe fails to form in the ventral region where dense iridophores appear in irregular positions. Later at stage J++ irregular stripes and spots become visible in luc loss-of-function and leot1 mutants (L and M), in luctXG1 and leotK3 mutants (N and O) the number of melanophores is low and dense iridophores cover increasing areas on the flank of the fish. Scale bars: 0.25 mm.
Requirement of leo and luc function in chromatophores
To understand the function of leo and luc in the different types of chromatophores, we analysed combinations with mutants lacking one of the three chromatophore types, in which incomplete residual striped patterns are generated (Maderspacher and Nusslein-Volhard, 2003; Frohnhofer et al., 2013). We created double mutants with leotK3, where leo and luc functions are abolished, and observed that the residual striped pattern is lost in all three cases (Figure 5). In nac/mitfa mutants, where melanophores are absent, light stripe fields of dense iridophores covered by xanthophores are enlarged and display irregular boundaries between regions of blue iridophores characteristic for the dark stripe (Figure 5A,A′). Double mutants of nac with leotK3 show an expansion of dense iridophores covered with xanthophores, in some cases blue iridophore regions are completely lost (Figure 5B). In pfe/csf1rA homozygotes, which lack xanthophores, melanophore stripes are broken into rows of spots separated by dense iridophores, and isolated melanophores appear in the light stripe regions (Figure 5C,C′). If pfe is combined with leotK3, individual isolated melanophores are evenly dispersed over the flank of the fish that are almost uniformly covered with dense iridophores (Figure 5D). In mutants lacking iridophores, tra/mpv17, shd/ltk, or rse/ednrb1b, the first two melanophore stripes are broken into spots, and further stripes are missing (see Figure 5E,E′ for shd and Figure 5—figure supplement 1G,G′ for tra). In the double mutants with leotK3, only few, if any, melanophores are present that are scattered without forming an aligned pattern (Figure 5F). We also analysed these double mutant combinations with leot1 or luck.o. (Figure 5—figure supplement 1) and observed very similar, albeit slightly weaker double mutant phenotypes.
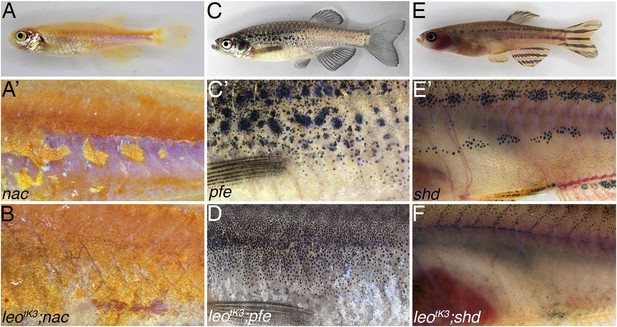
Complete loss of the pigment pattern in double mutants of nac, pfe, and shd with leotK3.
Mutants lacking one pigment cell type, nac (A and A′), pfe (C and C′), and shd (E and E′), only form remnants of the pigmentation pattern. In nac mutants fields of dense iridophores covered with compact xanthophores (corresponding to light stripes) and loose iridophores (corresponding to dark stripes) are discernible as patches (A′). In homozygous double mutants leotK3;nac (B) the regions of dense iridophores covered by compact xanthophores expand at the expense of blue iridophore areas. In pfe mutants (C) in the absence of xanthophores the melanophores form patches in the regions around the first light stripe but are increasingly more disorganized at some distance to it (C′). In double mutants of pfe with homozygous leotK3 melanophores are distributed almost evenly as individual cells over the flank of the fish (D). In shd mutants (E), where no iridophores are present, melanophores and xanthophores only partly form the first light and dark stripes (E′). In double homozygous double mutants leotK3;shd this residual pattern is lost and only very few individual melanophores are left on the flank of the fish (F).
In the mutants, the strongest phenotypic effect is seen in iridophores, which seem to appear mostly in the dense form, representative of the light stripes. However, iridophores do not autonomously require the leo or luc function (see below, Figure 6A,A′). This suggests that iridophore behaviour is affected via xanthophores and/or melanophores. From the pfe phenotype an inhibitory effect of xanthophores on the expansion of dense melanophores has been deduced: in the absence of xanthophores, the dense iridophore regions of the light stripe expand and ingress into the stripe region, breaking it up into spots (Frohnhofer et al., 2013). Therefore, we assume that xanthophores mutant for leo and luc have lost their ability to restrict the expansion of dense iridophores. This explains the reduction of blue iridophore regions in the leotK3;nac double mutants, when compared with nac alone.
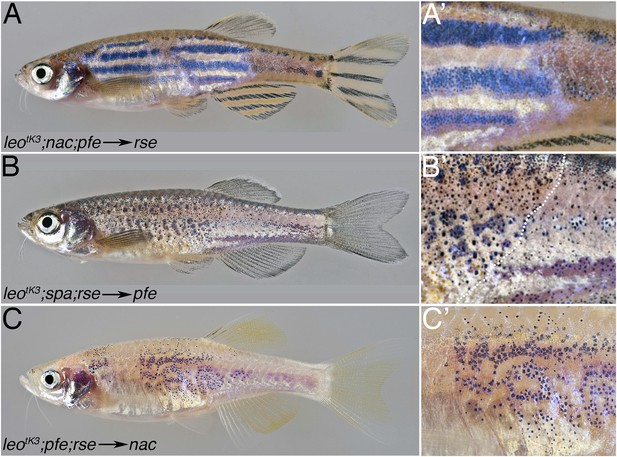
leo gap junctions are required in melanophores and xanthophores but not in iridophores.
Typical examples of chimeric animals derived from blastomere transplantations are shown. Cells from leotK3;nac;pfe donor embryos, which can provide iridophores as their only chromatophore type, were transplanted in rse hosts, which lack most iridophores. The resulting chimeric animals (17 clones in 34 fish) show that mutant iridophores form a wild-type pattern when confronted with wild-type xanthophores and melanophores (A and A′). When cells are transplanted from leotK3;spa;rse donors into pfe hosts, thereby creating chimeras with mutant xanthophores (present in the anterior part of the fish, left to the dotted line in B′) and wild-type iridophores and melanophores, there is no wild-type pattern formed, but the chromatophores are almost uniformly distributed (B and B′) (9 clones in 72 fish). When cells are transplanted from leotK3;pfe;rse donors into nac hosts, thereby creating chimeras with mutant melanophores and wild-type iridophores and xanthophores (3 clones in 68 fish), again, no wild-type pattern is formed, but the mutant melanophores form loosely arranged fields of cells (C and C′).
A requirement for leo and luc in melanophores, as observed in the chimeras (see below, Figure 6C), is corroborated by the phenotypes of the double mutants with pfe: if leo and luc were only required in xanthophores, the phenotypes of pfe mutants, which lack xanthophores completely, and the double mutants should be identical. However, we find an even distribution of melanophores in the double mutants rather than the series of spots that are still present in pfe (Figure 5C,D). This phenotype suggests a requirement of leo and luc for the homotypic association of melanophores.
Cx41.8 and Cx39.4 channels are required in xanthophores and melanophores but not in iridophores
Chimeric animals created by blastomere transplantations suggested that the function of leo is required in melanophores and in xanthophores (Maderspacher and Nusslein-Volhard, 2003). The requirement in iridophores had not been tested. We assessed the requirement in all three chromatophore types by creating chimeric animals with the allele leotK3, where all leo and luc function is absent. In these experiments, we used appropriate combinations of mutant donor and host embryos to assert that in the chimeric animals only one of the three chromatophore types was mutant. First, we tested whether luc and leo have any function in iridophores. Therefore we transplanted cells from nac;pfe;leotK3 donors into rse hosts (that lack iridophores). The donor embryos, due to mutations in nac and pfe, provide mutant iridophores as the only chromatophore type, whereas the melanophores and xanthophores of the chimeric animals must result from the rse mutant host that can form only rudimentary stripes. Thus, chimeric animals develop with leotK3 mutant iridophores juxtaposed to wild-type xanthophores and melanophores. In these animals, clusters of donor derived mutant iridophores can cause a complete restoration of the stripe pattern (Figure 6A,A′), demonstrating that the function of leo and luc is not required in iridophores. Next, we tested the requirement for leo and luc function in xanthophores. Therefore we created chimeric fish with mutant xanthophores next to wild-type melanophores by transplanting cells from leotK3;spa;rse mutant blastula embryos (that provide mutant xanthophores [Parichy et al., 1999]) into pfe hosts (lacking xanthophores). Whereas the transplantation of wild-type xanthophore progenitors can completely restore the striped pattern (Parichy and Turner, 2003), the chimeric animals that received leotK3 xanthophores display a distribution of melanophores strongly resembling the leotK3 mutant phenotype, indicating that mutant xanthophores cannot restore the wild-type striped pattern (Figure 6B,B′). Therefore the leo;luc function must be required cell autonomously in xanthophores. Finally, we assessed the function of leo and luc in melanophores by transplanting cells from leotK3;pfe;rse donor embryos into nac hosts. In these cases, where mutant melanophores are confronted with non-mutant xanthophores and iridophores, we again did not get a normal stripe pattern. However, the mutant melanophores did cluster together producing meandering fields of cells rather than creating spots or staying as individual cells, like in leotK3 (Figure 6C,C′). This indicates that the function of leo and luc is required in melanophores, but the wild-type xanthophores are able to exert some normalising influence over the mutant melanophores. Similar transplantation experiments using leot1 or luctXG1 embryos instead of leotK3 confirm that leo and luc are required in xanthophores and, to a lesser extent, in melanophores, but not in iridophores (Figure 6—figure supplement 1). Taken together, heteromeric connexons formed by Cx41.8 and Cx39.4 are not required in iridophores, but the function of leo and luc is required in melanophores and xanthophores instructing iridophores.
Discussion
A new connexin involved in pigment pattern formation
The leo phenotype varies, depending on the strength and combination of different alleles, from irregular, wavy stripes that are occasionally interrupted, over rows of spots to a complete loss of the pigment pattern, where isolated melanophores remain dispersed in a uniform background of dense iridophores and xanthophores. In the complete absence of Cx41.8 protein, in leot1 mutants, there is still some patterning activity present, leading to the formation of spots. The homozygous leotK3 phenotype is considerably stronger than this, indicating that the mutant Cx41.8 protein present in leotK3 cells has a dominant negative effect on some further component(s) involved in pigment pattern formation. In a simple model, this additional factor could be another connexin involved in the formation of heteromeric gap junctions together with Cx41.8, a notion that has been suggested previously, based on the analysis of leotq270 (Watanabe et al., 2006). The zebrafish genome contains 37 genes coding for connexins and it is impossible to deduce from the predicted amino acid sequences alone possible partners for Cx41.8. Therefore we performed a mutagenesis of leot1 fish and screened for mutants enhancing the phenotype to a strength similar to leotK3. In this screen we identified two dominant alleles of luc; we show that luc encodes another connexin, Cx39.4, and that loss-of-function of luc leads to a phenotype very similar to that of leot1. The loss of both connexins results in a phenotype that is as strong as the one seen in homozygous mutants for the dominant alleles of leo or luc. Therefore the strong phenotypes of the dominant alleles can be fully explained by the loss of the two proteins.
Cx39.4 is a fish specific connexin, closely related sequences can only be found in the genomes of other teleost fish. It has been classified either as novel (Eastman et al., 2006) or placed into an orthology group together with mammalian Cx37 (GJA4) (Cruciani and Mikalsen, 2006) with which it shares 54% amino acid similarity. However, Cx39.4 is the only α-Connexin where the N-terminal cytoplasmic domain of 23 amino acids is extended by two residues. As in the case of Cx41.8, the N-terminal cytoplasmic domain in Cx39.4 also contains an ExxE motif, therefore the conductivity of gap junctions formed by Cx39.4 could also be regulated by polyamines (Musa et al., 2004; Watanabe et al., 2012). Both, Cx41.8 and Cx39.4, have neither an R type nor a W type sequence motifs regulating heteromeric compatibility (Koval et al., 2014), so that no sequence based predictions about their ability to form heteromeric complexes are possible.
Requirement for leo and luc function in chromatophores
Based on transplantation experiments, the function of leo was reported to be required in melanophores and in xanthophores (Maderspacher and Nusslein-Volhard, 2003). We repeated the experiments with the loss-of-function allele leot1, the new strong allele leotK3, and one of our dominant alleles of luc as donors. In agreement with the previous study, we show that leo function is needed in melanophores and xanthophores. We find the same requirement for luc function in both cell types. But neither leo nor luc has a role in iridophores. The results of our transplantation experiments also show that there is no contribution of non-pigment cells to the leo and luc phenotypes. Otherwise we would not have expected to find the complete rescue of a wild-type pattern to coincide in all cases with the transplanted iridophores into the shd or tra mutants.
Mutant melanophores, in chimeric animals, seem to be influenced by wild-type xanthophores in their neighbourhood, as they form continuous meandering patches larger than the spots found in leo or luc mutants. However, unlike wild-type melanophores when transplanted into nac hosts (Maderspacher and Nusslein-Volhard, 2003), they do not form normal stripes, so clearly leo and luc are required in melanophores for the formation of a wild-type pattern. The function of both genes is also required in xanthophores; mutant xanthophores, when confronted with wild-type melanophores in the chimeras, do not generate a wild-type pattern but spot or disperse melanophores in large dense iridophore regions. When we tested iridophores we found that they require neither leo nor luc function, as the mutant cells can contribute to a completely wild-type pattern if they are next to other wild-type cells. This is surprising and indicates that the dramatic expansion of the dense iridophores that is associated with the mutant phenotypes is an indirect effect, possibly caused by mutant xanthophores covering the iridophores.
Gap junction communication between chromatophores
Taken together our data suggest that Cx41.8 and Cx39.4 interact to form heteromeric gap junctions and that these channels are responsible for some of the interactions that take place among chromatophores while the pigment pattern is established. This is corroborated by our analysis of double mutants where one chromatophore type is missing and leo or luc is mutant. In the absence of xanthophores, in pfe mutants, melanophores cluster together; this clustering is lost in double mutants with leotK3, where leo and luc function are affected, indicating that the channels are responsible for homotypic interactions among melanophores. In the double mutants of leotK3 with nac, where melanophores are missing, mutant xanthophores are unable to confine the light stripe regions, suggesting an instructive interaction between xanthophores and iridophores during normal development. In double mutants with shd or tra, where iridophores are lacking, the number of melanophores is further reduced and xanthophores are evenly distributed, indicating that in wild-type there might be a positive interaction between melanophores and xanthophores via gap junctions. The phenotypes of the double mutants with the leo or luc loss-of-function alleles are generally a bit weaker than with leotK3. This indicates that in the absence of one of the connexins, when heteromeric channels cannot form, homomeric channels retain some functionality.
The leo and luc mutant phenotypes seem to be caused by iridophores not behaving appropriately and failing to undergo the transition from the dense to the loose form at the correct time and place. Recently it was shown that proliferation and dispersal of iridophores during metamorphosis, the time when the adult pigment pattern is generated, outline the light and dark stripe regions in the trunk of zebrafish (Singh et al., 2014); at the same time the larval xanthophores also start to proliferate, they re-organize and change their shapes to densely cover the light stripe regions and loosely the dark melanophore stripes (Mahalwar et al., 2014). This raises the possibility that in normal development the xanthophores, which cover the iridophores, induce the transition to the loose form when they reach the regions destined to form dark stripes. It is interesting in this context that in schachbrett (sbr) mutants, which display interrupted dark stripes, the affected gene is specifically expressed in iridophores and codes for Tight Junction Protein 1a (Tjp1a, ZO-1) (Andrey Fadeev, JK, HGF, and CN-V, submitted). If gap junctions are indeed involved in the signalling events between xanthophores and iridophores, there must be (at least) one, so far unidentified, connexin, which is expressed in iridophores. Alternatively, other signalling pathways could be responsible for the cross-talk between iridophores and xanthophores, and gap junctions might only be required for the homotypic interactions among xanthophores. Whether direct interactions between xanthophores and melanophores, which have been demonstrated in an in vitro system (Yamanaka and Kondo, 2014), are also possible in vivo is not known. In the skin of the fish these two cell types are always separated by a layer of iridophores (Hirata et al., 2003, 2005; Watanabe et al., 2006), however, recently it has been shown that melanophores of the dark stripe produce long cellular extensions reaching towards the light stripe xanthophores (Hamada et al., 2014); these extensions could allow direct contact between melanophores and xanthophores. These direct cell-to-cell contacts would permit communication between the cells via gap junctions.
Our data suggest that in the absence of one connexin-partner, homomeric channels can exist, but they retain only some residual functionality, as can be seen in leo or luc loss-of-function mutants. The non-functional proteins present in the dominant mutants can lead to a complete block of activity, equal to the one seen in the leok.o.;luck.o. double mutants. Interestingly, in luc mutants the fins are striped, suggesting that homomeric leo channels suffice for patterning the fins, where stripes can be formed in the absence of iridophores.
Materials and methods
Fish husbandry
Request a detailed protocolZebrafish were maintained as described earlier (Brand et al., 2002); we used the following genotypes: Tübingen, leot1, nacW2, pfetm236b, rsetLF802, shdj9s1, spab134, trab6. ENU mutagenesis was performed as described (Rohner et al., 2011), 40 male fish were mutagenized over the course of 6 weeks. All animal experiments were performed in accordance with the rules of the State of Baden-Württemberg, Germany, and approved by the Regierungspräsidium Tübingen (Aktenzeichen: 35/9185.81-5/Tierversuch-Nr. E 1/09).
Genotyping
Request a detailed protocolFor the PCR amplification of genomic DNA from fin clips, the tissue samples were incubated in TE supplemented with 5% Chelex-100 (BioRad Laboratories, Hercules, CA) and 10 µg/ml Proteinase K (Roche, Germany) for 4 hr at 55°C and 10 min at 95°C and then stored at 4°C. 0.1–1 µl of the supernatant was used as template in a standard PCR of 25 µl. The following oligonucleotides were used as primers:
leo_for1: 5′-CTCTTATTTCCACCTCAGGCTCC-3′
leo_for2: 5′-GCGGTGTCACTGCTGCTTAGTATC-3′
leo_rev1: 5′-CTATTCGACTGCATGAAGGTTGC-3′
leo_rev2: 5′-CAACTGCTTCCATGCCAAATG-3′
obe_for1: 5′-GAAACTATTCTTGCCGTGACTTGG-3′
obe_for2: 5′-TGTTCACAGTGACATGCACAGATG-3′
obe_rev1: 5′-TCAAACAAACCTGGGTGTGGAC-3′
obe_rev2: 5′-GTGTACCTAACAGGGCAAACGG-3′
luc_for1: 5′-TGCCTCTAGGAACATGATTGGG-3′
luc_rev1: 5′-TCAAACATAATGTCTCGGTTTG-3′
luc_rev2: 5′-CGGAGTTGAGACGAAGATGACC-3′
Generation of luc k.o.
Request a detailed protocolThe luc loss-of-function was generated using the CRISPR-Cas system as described in Hwang et al. (2013). The following oligonucleotides were cloned into pDR274 to generate the sgRNA vector:
Cx39.4_1: 5′-TAGGAAGGCCAGGAGTACTCGA-3′
Cx39.4_2: 5′-AAACTCGAGTACTCCTGGCCTT-3′
One-cell stage embryos were injected with 2–4 nl of a solution containing 200 ng/µl Cas9 mRNA and 15 ng/µl sgRNA. Cas9 mRNA was transcribed from the linearized plasmid pMLM3613 using the mMessage mMachine Kit and a polyA tail was added with the Poly(A) Tailing Kit (both Ambion(Europe), UK). The sgRNA was transcribed from the linearized vector using the Megascript Kit (Ambion). The efficiency of indel generation was tested on eight larvae at 4 dpf by PCR and sequence analysis. The other injected larvae were raised to adulthood and mature F0 fish with pigmentation pattern defects were incrossed. F1 fish from these crosses were genotyped and fish with 2 bp and 22 bp deletions were used to establish mutant stocks.
Blastula transplantations
Request a detailed protocolChimeric animals were generated by transplantations of cells during blastula stage as described (Kane and Kishimoto, 2002).
Image acquisition and processing
Request a detailed protocolAdult fish were anaesthetised with 0.004% MS-222 (Sigma-Aldrich, Germany) and photographed with a Canon EOS 5D MarkII camera and a Macro 100 objective. Metamorphic fish (Figure 4) were anaesthetised, embedded in 1.5% low melting point agarose with 4.5 mg/ml ± epinephrine (Sigma-Aldrich) for melanosome contraction, and photographed under a Leica M205 FA stereomicroscope with a Leica DCF300 FX camera using the software LAS V4.1 to allow multifocus images.
References
-
Zebrafish: a practical approachZebrafish: a practical approach, Oxford, New York, NY, Oxford University Press.
-
The vertebrate connexin familyCellular and Molecular Life Sciences 63:1125–1140.https://doi.org/10.1007/s00018-005-5571-8
-
Mutations affecting pigmentation and shape of the adult zebrafishDevelopment Genes and Evolution 206:260–276.https://doi.org/10.1007/s004270050051
-
Pigment cell organization in the hypodermis of zebrafishDevelopmental Dynamics 227:497–503.https://doi.org/10.1002/dvdy.10334
-
Efficient genome editing in zebrafish using a CRISPR-Cas systemNature Biotechnology 31:227–229.https://doi.org/10.1038/nbt.2501
-
Tetraspanin 3c requirement for pigment cell interactions and boundary formation in zebrafish adult pigment stripesPigment Cell & Melanoma Research 27:190–200.https://doi.org/10.1111/pcmr.12192
-
BookCell labeling and transplantation techniquesIn: Nüsslein-Volhard C, Dahm R, editors. Zebrafish: A Practical Approach. New York: Oxford University Press. pp. 95–119.
-
Biological role of connexin intercellular channels and hemichannelsArchives of Biochemistry and Biophysics 524:2–15.https://doi.org/10.1016/j.abb.2012.03.008
-
Untersuchungen über das farbmuster der zebrabarbe 'brachydanio rerio' (Cyprinidae, Teleostei)Wilhelm Roux's Archives of Developmental Biology 177:129–152.https://doi.org/10.1007/BF00848526
-
Zur Genetik einiger Farbmustermutanten der zebrabarbe 'Brachydanio rerio' (Cyprinidae, Teleostei) und zum Phänotyp von Artbastarden der Gattung 'Brachydanio'Biologisches Zentralblatt 96:211–222.
-
nacre encodes a zebrafish microphthalmia-related protein that regulates neural-crest-derived pigment cell fateDevelopment 126:3757–3767.
-
Topology of the 32-kd liver gap junction protein determined by site-directed antibody localizationsThe EMBO Journal 7:2967–2975.
-
Interactions between zebrafish pigment cells responsible for the generation of Turing patternsProceedings of the National Academy of Sciences of USA 106:8429–8434.https://doi.org/10.1073/pnas.0808622106
-
Mutations affecting xanthophore pigmentation in the zebrafish, Danio rerioDevelopment 123:391–398.
-
An orthologue of the kit-related gene fms is required for development of neural crest-derived xanthophores and a subpopulation of adult melanocytes in the zebrafish, Danio rerioDevelopment 127:3031–3044.
-
Zebrafish sparse corresponds to an orthologue of c-kit and is required for the morphogenesis of a subpopulation of melanocytes, but is not essential for hematopoiesis or primordial germ cell developmentDevelopment 126:3425–3436.
-
Changing clothes easily: connexin41.8 regulates skin pattern variationPigment Cell & Melanoma Research 25:326–330.https://doi.org/10.1111/j.1755-148X.2012.00984.x
-
Pattern regulation in the stripe of zebrafish suggests an underlying dynamic and autonomous mechanismProceedings of the National Academy of Sciences of USA 104:4790–4793.https://doi.org/10.1073/pnas.0607790104
-
In vitro analysis suggests that difference in cell movement during direct interaction can generate various pigment patterns in vivoProceedings of the National Academy of Sciences of USA 111:1867–1872.https://doi.org/10.1073/pnas.1315416111
Article and author information
Author details
Funding
European Commission (ZF-HEALTH grant, 242048)
- Christiane Nüsslein-Volhard
The funders had no role in study design, data collection and interpretation, or the decision to submit the work for publication.
Acknowledgements
We thank Brigitte Walderich for her support with the zebrafish work, Christian Söllner, Prateek Mahalwar, and Ajeet Singh for many insightful discussions, and Ajeet Singh for his comments on the manuscript.
Ethics
Animal experimentation: All animal experiments were performed in accordance with the rules of the State of Baden-Württemberg, Germany. The protocol for ENU mutagenesis was approved by the Regierungspräsidium Tübingen (Aktenzeichen: 35/9185.81-5/Tierversuch-Nr. E 1/09).
Copyright
© 2014, Irion et al.
This article is distributed under the terms of the Creative Commons Attribution License, which permits unrestricted use and redistribution provided that the original author and source are credited.
Metrics
-
- 2,530
- views
-
- 320
- downloads
-
- 88
- citations
Views, downloads and citations are aggregated across all versions of this paper published by eLife.
Citations by DOI
-
- 88
- citations for umbrella DOI https://doi.org/10.7554/eLife.05125