A host enzyme reduces metabolic dysfunction-associated steatotic liver disease (MASLD) by inactivating intestinal lipopolysaccharide
eLife Assessment
This important study highlights the key role of the gut–liver axis mediated by LPS in causing hepatic steatosis. The authors provide solid evidence, in vivo, in vitro, and in silico, for the role of acyloxyacyl hydrolase in mediating this effect using KO mice subjected to MASD-inducing diets. The findings are significant for the liver research community and others interested in the gut–liver axis.
https://doi.org/10.7554/eLife.100731.3.sa0Important: Findings that have theoretical or practical implications beyond a single subfield
- Landmark
- Fundamental
- Important
- Valuable
- Useful
Solid: Methods, data and analyses broadly support the claims with only minor weaknesses
- Exceptional
- Compelling
- Convincing
- Solid
- Incomplete
- Inadequate
During the peer-review process the editor and reviewers write an eLife Assessment that summarises the significance of the findings reported in the article (on a scale ranging from landmark to useful) and the strength of the evidence (on a scale ranging from exceptional to inadequate). Learn more about eLife Assessments
Abstract
The incidence of metabolic dysfunction-associated steatotic liver disease (MASLD) has been increasing worldwide. Since gut-derived bacterial lipopolysaccharides (LPS) can travel via the portal vein to the liver and play an important role in producing hepatic pathology, it seemed possible that (1) LPS stimulates hepatic cells to accumulate lipid, and (2) inactivating LPS can be preventive. Acyloxyacyl hydrolase (AOAH), the eukaryotic lipase that inactivates LPS and oxidized phospholipids, is produced in the intestine, liver, and other organs. We fed mice either normal chow or a high-fat diet for 28 weeks and found that Aoah-/- mice accumulated more hepatic lipid than did Aoah+/+ mice. In young mice, before increased hepatic fat accumulation was observed, Aoah-/- mouse livers increased their abundance of sterol regulatory element-binding protein 1, and the expression of its target genes that promote fatty acid synthesis. Aoah-/- mice also increased hepatic expression of Cd36 and Fabp3, which mediate fatty acid uptake, and decreased expression of fatty acid-oxidation-related genes Acot2 and Ppara. Our results provide evidence that increasing AOAH abundance in the gut, bloodstream, and/or liver may be an effective strategy for preventing or treating MASLD.
Introduction
Metabolic dysfunction-associated steatotic liver disease (MASLD) is a common human affliction. Its global prevalence, currently about 25%, has been increasing (Diehl and Day, 2017; Fan et al., 2017; Younossi et al., 2018). MASLD may progress from nonalcoholic fatty liver to nonalcoholic steatohepatitis, cirrhosis, and even hepatic cancer (Friedman et al., 2018; Sheka et al., 2020). Multiple factors may contribute to its pathogenesis (Friedman et al., 2018) prominent among these are the lipopolysaccharides (LPS, endotoxins) produced by many of the Gram-negative bacteria that inhabit the intestine. Gut-derived LPS may translocate into the portal venous system and traffic to the liver, triggering or exacerbating hepatic inflammation (Albillos et al., 2020; Carpino et al., 2020; Han et al., 2021; Kazankov et al., 2019; Leung et al., 2016; Munford, 1978; Wang et al., 2022).
The LPS molecules that contribute to MASLD pathogenesis are able to stimulate host cells because their lipid A structure is recognized by MD-2/TLR4 receptors (Ye et al., 2012). Most γ-Proteobacteria such as Escherichia coli produce stimulatory hexaacyl LPS (with six acyl chains) while Bacteroidetes produce non-stimulatory LPS that has four or five acyl chains (Anhê et al., 2021; d’Hennezel et al., 2017). MASLD is often associated with intestinal dysbiosis produced by increased abundance of γ-Proteobacteria, which leads to tissue inflammation and increased intestinal permeability that allows even more gut-derived LPS to reach the liver (Albillos et al., 2020; Aron-Wisnewsky et al., 2020a; Aron-Wisnewsky et al., 2020b; Mouries et al., 2019; Rodrigues et al., 2024). Although gut-derived LPS is known to induce hepatic inflammation and exacerbate MASLD, how LPS influences hepatocyte fatty acid metabolism before MASLD develops has not been well understood.
Acyloxyacyl hydrolase (AOAH) is a highly conserved animal lipase that is mainly expressed in macrophages, monocytes, neutrophils, microglia, dendritic cells, NK cells, and ILC1 cells (Munford et al., 2020). It can inactivate Gram-negative bacterial LPSs by releasing two of the six fatty acyl chains present in the lipid A moiety (Figure 1A). It also can deacylate/inactivate oxidized phospholipids and lysophospholipids, molecules that are also known to contribute to MASLD (Sun et al., 2020; Zou et al., 2021). We previously reported that AOAH is expressed by gut macrophages and dendritic cells and can inactivate bioactive LPS in feces (Cheng et al., 2023; Janelsins et al., 2014; Qian et al., 2018). AOAH also inactivates LPS in the liver, diminishing and shortening hepatic inflammation induced by bloodborne LPS (Ojogun et al., 2009; Shao et al., 2011; Shao et al., 2007). Han et al. found that intestine-derived LPS can bind high-density lipoprotein 3 (HDL3) and be inactivated by AOAH as it traffics from the jejunum to the liver via the portal vein (Han et al., 2021). The enzyme’s ability to prevent MASLD by inactivating gut-derived LPS had not been tested (Ojogun, 2008).
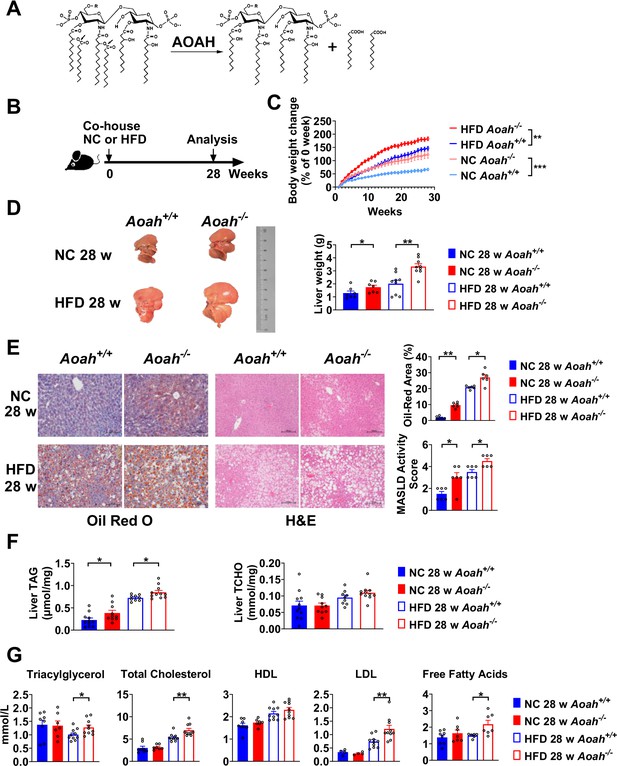
Acyloxyacyl hydrolase (AOAH) reduces hepatic lipid accumulation.
(A) AOAH cleaves the two piggyback fatty acyl chains from the lipid A moiety, inactivating lipopolysaccharides (LPS). The arrows indicate the cleavage sites. (B) Co-housed Aoah+/+ and Aoah-/- mice were fed either a normal diet (NC) or a high-fat diet plus high fructose (23.1 g/l) and glucose (18.9 g/l) in their drinking water (HFD) for 28 weeks. (C) Body weight was measured weekly for 28 weeks. Data were combined from four experiments. n=12–17. (D) Representative images of livers at 28 weeks are shown, and the liver weight was measured. n=7–9, each symbol represents one mouse. (E) Mouse livers were fixed, sectioned, and stained with Oil Red O or H&E. In Oil Red O-stained sections, the percentage of area occupied by the lipid droplets was quantified using ImageJ. H&E staining results were semi-quantitatively scored for disease severity. Data were combined from three experiments, n=6. Scare bars = 100 μm. (F) Triacylglycerol (TAG) and total cholesterol (TCHO) were measured in liver homogenates. Data were combined from at three experiments, n=9–11, each symbol represents one mouse. (G) The serum concentrations of triglyceride, TCHO, high-density lipoprotein (HDL), low-density lipoprotein (LDL), and free fatty acids were measured. Data were combined from three experiments. n=6–10, each symbol represents one mouse. (C–G) Mann–Whitney test and two-way ANOVA were used. *p<0.05; **p<0.01; ***p<0.001.
In this study, we found that when mice were fed either normal chow (NC) or a high-fat diet (HFD), AOAH reduced LPS-induced lipid accumulation in the liver, probably by decreasing the expression and activation of sterol regulatory element-binding protein 1 (SREBP1) (also called sterol regulatory element-binding transcription factor 1 [SREBF1]), an important transcription factor that promotes fatty acid synthesis (Horton et al., 2002; Shimano and Sato, 2017). AOAH also reduced the expression of Cd36 and Fabp3, fatty acid uptake-related genes, and increased that of fatty acid oxidation-related genes (Acot2 and Ppara). In addition, AOAH reduced hepatic inflammation and minimized tissue damage. Our results suggest that AOAH plays a regulatory role in ameliorating MASLD and that measures that increase AOAH abundance in the intestine, liver, and/or bloodstream may help prevent this common disease.
Results
AOAH reduces hepatic lipid accumulation
To find out if AOAH prevents MASLD, we fed co-housed Aoah+/+ and Aoah-/- mice NC or a HFD plus fructose and glucose in the drinking water, for 28 weeks (Figure 1B; Liu et al., 2018). Aoah-/- mice fed either NC or HFD gained more weight than did Aoah+/+ control mice (Figure 1C). When they were fed either NC or HFD, the livers of Aoah-/- mice were heavier than those of Aoah+/+ control mice (Figure 1D). Histological examination and Oil Red O staining revealed that Aoah-/- mouse livers accumulated more lipid droplets than did the livers of Aoah+/+ mice (Figure 1E). When we scored MASLD severity based on steatosis, hepatocyte ballooning degeneration, and inflammation, Aoah-/- mice developed more severe MASLD than did Aoah+/+ mice whether the mice were fed NC or HFD (Sheka et al., 2020; Figure 1E). When the mice were fed either NC or HFD, Aoah-/- mouse livers contained more triacylglycerol (TAG) than did Aoah+/+ mouse livers, while livers from both mouse strains contained a similar amount of total cholesterol (TCHO, Figure 1F). When the mice were fed the HFD, Aoah-/- mice had higher serum levels of TAG, TCHO, low-density lipoprotein (LDL), and free fatty acids than did Aoah+/+ mice (Figure 1G). Collectively, these findings were evidence that AOAH reduced hepatic TAG accumulation when mice were fed either NC or HFD.
AOAH prevents hepatic inflammation and tissue injury when mice are fed HFD
Aoah-/- mice fed HFD had significantly higher serum alanine aminotransferase (ALT) and aspartate aminotransferase (AST) levels than did control Aoah+/+ mice, suggesting that Aoah-/- mice experienced more severe liver inflammation and tissue damage (Figure 2A). To assess liver inflammation, we measured pro- and anti-inflammatory cytokine expression. When mice were fed the HFD, Aoah-/- mouse livers produced more pro-inflammatory Il6, Ifng, and anti-inflammatory Il10 mRNA than did Aoah+/+ mouse livers, suggesting greater inflammation (Figure 2B). We also found that Aoah-/- livers had more Timp1 (a pro-fibrosis gene) mRNA and less Mmp2 (an anti-fibrosis gene) mRNA than did Aoah+/+ mouse livers (Kisseleva and Brenner, 2021), indicating that Aoah-/- mouse livers may be developing more severe fibrosis, although we did not detect fibrosis with Masson staining (Figure 2C). We analyzed the myeloid cells in the liver (Daemen et al., 2021) and found that when the mice were fed the HFD, Aoah-/- mouse livers contained more neutrophils, monocytes, and lipid-associated macrophages (hepatic LAMs) (Remmerie et al., 2020; Su, 2002) than did Aoah+/+ mouse livers (Figure 2D and E). Collectively, when the mice were fed an HFD, the livers of Aoah-/- mice developed significantly greater inflammatory responses and tissue damage.
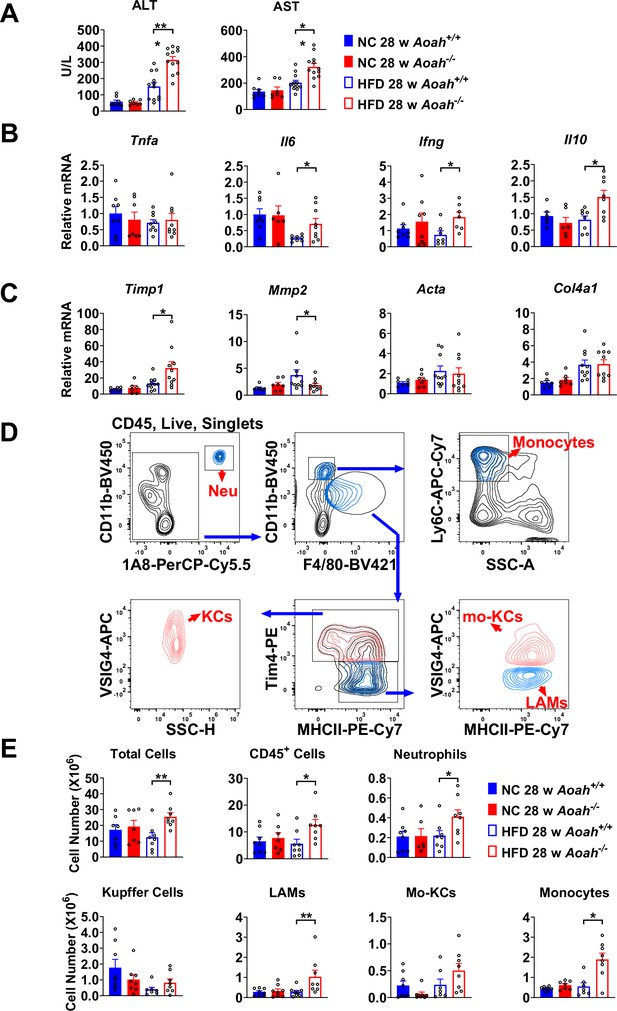
Acyloxyacyl hydrolase (AOAH) prevents hepatic inflammation and tissue injury when mice are fed high-fat diet (HFD).
(A) Serum alanine aminotransferase (ALT) and aspartate aminotransferase (AST) were measured at 28 weeks. Data were combined from three experiments, n=7–12. (B, C) Inflammation-related Tnfa, Il6, Ifng, Il10 mRNA and fibrosis-related Timp1, Mmp2, Acta, Col4a1 mRNA were measured in livers at 28 weeks. Data were combined from three experiments, n=6–10. (D) Gating strategy to identify hepatic neutrophils, monocytes, Kupffer cells, lipid-associated macrophages (LAM), and monocyte-derived Kupffer cells (Mo-KC) subsets. (E) The myeloid cell numbers in Aoah+/+ and Aoah-/- livers were calculated using FACS analysis. Data were combined from three experiments, n=6–8. Each symbol represents one mouse. Mann–Whitney test was used. *p<0.05; **p<0.01.
AOAH reduces hepatic LPS levels and the expression of fatty acid synthesis genes
We found previously that AOAH was mainly expressed in Kupffer cells in the liver (Shao et al., 2007). To confirm the previous results, we first consulted the single-cell RNA-seq analysis reported by Remmerie et al., 2020, who found that AOAH is expressed in Kupffer cells, monocytes, monocyte-derived cells, NK (circulating NK), and ILC1 (tissue resident NK) cells in mouse livers (Remmerie et al., 2020; Figure 3A). We used flow cytometry to sort mouse Kupffer cells (CD45+NK1.1- F4/80hiCD11bmid), monocytes (CD45+NK1.1- F4/80midCD11bhi), NK cells (CD45+SSClo NK1.1+) (including circulating NK and resident ILC1), and purified the hepatocytes. Using qPCR analysis, we found that AOAH mRNA was present in Kupffer cells, monocytes, and NK cells but not in hepatocytes, in keeping with previous findings (Shao et al., 2007; Figure 3B). Western blot analysisanalysis confirmed that Aoah+/+ mouse livers but not Aoah-/- mouse livers had AOAH protein (Figure 3C). When Aoah+/+ mice aged or had an HFD, their hepatic AOAH expression increased (Figure 3D). We also studied the expression of AOAH in human liver cells by analyzing the single-cell RNA-seq data obtained by Ramachandran et al., 2019; AOAH was expressed in macrophages, monocytes, resident and circulating NK cells, and some T cells (Figure 3E). AOAH expression increased in liver macrophages and monocytes in MASLD patients (Figure 3E). As gut-derived LPS can be transported via the portal vein into the liver (Han et al., 2021), we hypothesized that AOAH prevents hepatic inflammation and fat accumulation by inactivating LPS in the gut, portal vein, and liver. We found that Aoah-/- mouse feces, liver, and plasma had higher bioactive LPS levels when Aoah-/- and Aoah+/+ mice were fed either NC or HFD (Figure 3F). HFD increased gut permeability, but there was no permeability difference between Aoah+/+ and Aoah-/- mice that were fed either NC or HFD for 28 weeks, suggesting that the increased hepatic LPS levels in Aoah-/- mouse livers were mainly caused by failure to inactivate LPS (Figure 3G). After the mice were fed HFD for 28 weeks, Aoah-/- mouse livers increased fatty acid uptake gene Fabp3 mRNA and fatty acid synthesis gene Fasn mRNA, changes that may have contributed to lipid accumulation (Figure 3H). Thus, AOAH may prevent hepatic lipid accumulation by diminishing bioactive LPS in the liver.
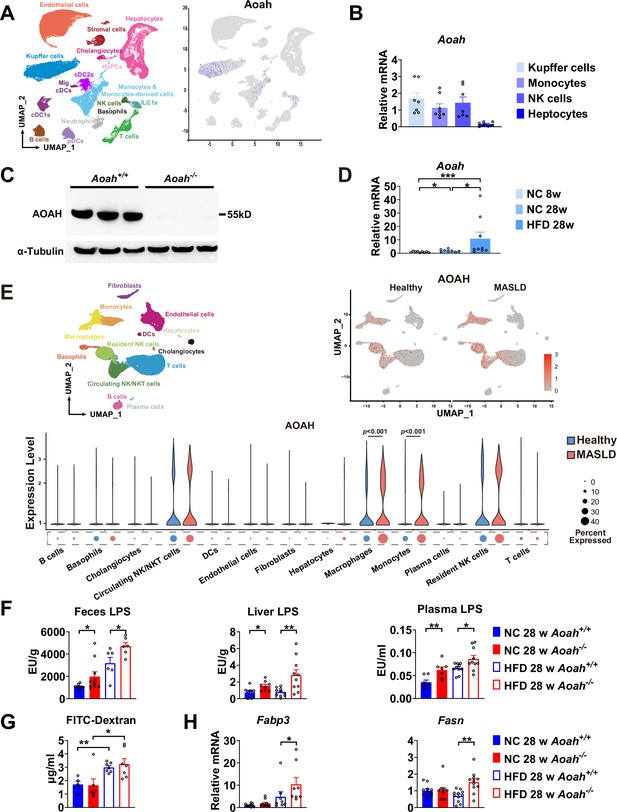
Acyloxyacyl hydrolase (AOAH) reduces hepatic lipopolysaccharides (LPS) levels and the expression of fatty acid uptake and synthesis genes.
(A) AOAH expression in mouse hepatic cells based on single-cell analysis by Remmerie et al., 2020 is shown, n=4. (B) Kupffer cells, monocytes, and NK cells were sorted using flow cytometry and hepatocytes were purified from 6- to 8-week-old mice. AOAH mRNA was measured. Data were combined from three experiments, n=7–8, each symbol represents one mouse. (C) Using western blot analysis, we confirmed that AOAH protein was present in 6–8 week-old Aoah+/+ mouse livers but not in Aoah-/- mouse liver homogenates. Similar results were obtained in two other experiments. (D) AOAH mRNA levels were measured in the livers of Aoah+/+ mice fed with a normal chow for 8 and 28 weeks, and a high-fat diet for 28 weeks. Data were combined from 2 experiments, n=5–7, each symbol represents one mouse. (E) Single-cell RNA sequencing data from livers of MASLD patients and healthy controls by Ramachandran et al., 2019. n=5/group. (F) Heat-inactivated feces suspension, liver homogenates, and plasma from Aoah+/+ and Aoah-/- mice were tested for TLR4-stimulating activity. Data were combined from at least three experiments, n=6–10, each symbol represents one mouse. (G) Gut permeability was measured. Mice were fasted for 18 h. Mice were orally gavaged with fluorescein isothiocyanate (FITC)-conjugated dextran and 4 h later, FITC fluorescence was measured in plasma. Data were combined from three experiments, n=5–7, each symbol represents one mouse. (H) At 28 weeks of normal diet (NC) or high-fat diet (HFD) feeding, liver Fabp3 and Fasn mRNAs were measured. Data were combined from three experiments, n=8–11, each symbol represents one mouse. Mann–Whitney test was used. *p<0.05; **p<0.01.
-
Figure 3—source data 1
Original tiff files of western blots for Figure 3C.
- https://cdn.elifesciences.org/articles/100731/elife-100731-fig3-data1-v1.zip
-
Figure 3—source data 2
Original tiff files containing uncropped western blots with labeling for Figure 3C.
- https://cdn.elifesciences.org/articles/100731/elife-100731-fig3-data2-v1.zip
AOAH can regulate the expression of hepatic fatty acid metabolism genes
We found that AOAH reduced hepatic lipid accumulation when mice were about 8 months old if they were fed either NC or HFD. To investigate the mechanism, we analyzed the livers of young mice. We co-housed 3–4-week-old Aoah+/+ and Aoah-/- mice for 3–4 more weeks before removing their livers for RNA-seq analysis. The expression of several fatty acid biosynthesis genes, such as Acacb (acetyl-coenzyme A carboxylase beta), Acss2 (acetyl-CoA synthetase 2), Pcx (pyruvate carboxylase), Acly (ATP citrate lyase), Fasn (fatty acid synthase), and Scd1 (stearoyl-coenzyme A desaturase 1), was significantly increased in Aoah-/- mouse livers (Figure 4A). When we used gene set enrichment analysis (GSEA) to analyze deferentially expressed genes, we found that the fatty acid biosynthesis pathway was enriched (Figure 4B). We then did qPCR and confirmed the increases in mRNAs for FA biosynthesis genes described in Figure 4A as well as for Acaca (acetyl-coenzyme A carboxylase alpha, encoding ACC1, the first and key enzyme on FA synthesis pathway) in Aoah-/- mouse livers compared with Aoah+/+mouse livers (Figure 4C). In addition, mRNAs for enzymes involved in fatty acid oxidation (Acot2 [acyl-CoA thioesterase 2] and Ppara [peroxisome proliferator-activated receptor α]) (Bougarne et al., 2018; Moffat et al., 2014) decreased (Figure 4D), while Cd36 (fatty acid uptake; Chen et al., 2022) mRNA levels increased in Aoah-/- mouse livers (Figure 4E). We confirmed that FASN and SCD1 protein levels also increased in Aoah-/- mouse livers (Figure 4F). We found previously that LPS and other TLR agonists increase lipid accumulation in cultured macrophages by increasing expression of Acsl1 (acyl-CoA synthetase long-chain family member 1) and Dgat2 (diacylglycerol O-acyltransferase 2) and by reducing the production of Pnpla2 (Patatin-like phospholipase domain containing 2, Atgl) (Huang et al., 2014), yet the livers of Aoah+/+ and Aoah-/- mice had similar levels of Acsl1, Dgat2, and Pnpla2 mRNA, suggesting that AOAH does not regulate hepatic TAG metabolism (Figure 4—figure supplement 1). These results suggest that AOAH reduces liver fat accumulation by diminishing the expression of fatty acid synthesis and uptake genes and increasing that of fatty acid oxidation genes.
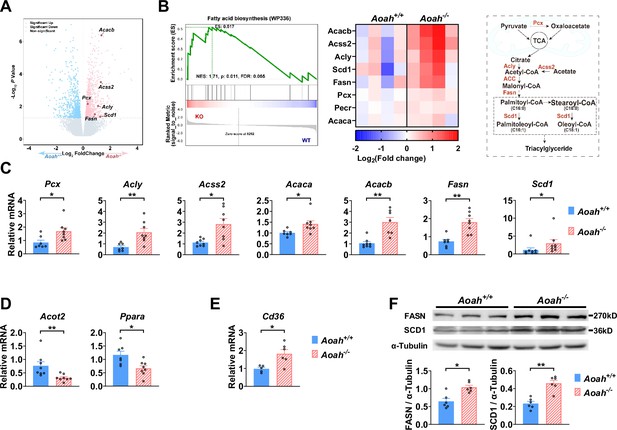
Acyloxyacyl hydrolase (AOAH) regulates the expression of hepatic fatty acid metabolism genes.
(A, B) Co-housed 6–8-week-old (i.e., young) Aoah+/+ and Aoah-/- mouse livers were subjected to RNA-seq analysis. The differentially expressed genes are shown in the volcano plot (A). The fatty acid biosynthesis pathway was found enriched using gene set enrichment analysis (GSEA) (B, left panel). The fatty acid biosynthesis-associated gene expression levels of co-housed Aoah+/+ and Aoah-/- mouse livers are shown as a heatmap, n=4 (B, middle panel). The fatty acid biosynthesis pathway is shown. The enzymes marked red had increased expression in young Aoah-/- mouse livers (B, right panel). (C–E) The hepatic expression of fatty acid synthesis (C), oxidation (D), and uptake (E) genes was measured in co-housed 6–8-week-old Aoah+/+ and Aoah-/- mice. Data were combined from three experiments, n=5–8, each symbol represents one mouse. (F) Liver homogenates from co-housed 6–8-week-old Aoah+/+ and Aoah-/- mice were subjected to western analysis. FASN, SCD1, and α-tubulin protein levels were quantitated using ImageJ. Data were combined from two experiments, n=6, each symbol represents one mouse. Mann–Whitney test was used. *p<0.05; **p<0.01.
-
Figure 4—source data 1
Original tiff files of western blots for Figure 4F.
- https://cdn.elifesciences.org/articles/100731/elife-100731-fig4-data1-v1.zip
-
Figure 4—source data 2
Original tiff files containing uncropped western blots with labeling for Figure 4F.
- https://cdn.elifesciences.org/articles/100731/elife-100731-fig4-data2-v1.zip
AOAH reduces hepatic SREBP1
As Acaca, Fasn, and Scd1 are all target genes for sterol regulatory element-binding protein 1 (SREBP1, encoded by Srebf1 gene), a key transcription factor for fatty acid biosynthesis, we next analyzed SREBP1 expression in the liver (Horton et al., 2002; Shimano and Sato, 2017; Yokoyama et al., 1993). There are two isoforms of SREBP1, SREBP1a, and SREBP1c, and the liver predominantly expresses SREBP1c (Horton et al., 2002). The abundance of Srebf1a and Srebf1c mRNA increased in the livers of young Aoah-/- mice (Figure 5A). SREBP1 is synthesized as a 125 kDa precursor (full length, flSREBP1) in the endoplasm reticulum, transferred to the Golgi apparatus, and cleaved sequentially by Site-1 protease and Site-2 protease to generate nuclear SREBP1 (nSREBP1, 68 kDa), which enters the nucleus and activates fatty acid biosynthesis gene transcription (Horton et al., 2002; Shimano and Sato, 2017). The livers mainly had the short-form (68 kDa) nSREBP1 protein, which was significantly more abundant in Aoah-/- mice (Figure 5B). We separated liver cytosol and nuclei and found that the short-form SREBP1 was mainly present in nuclei and that Aoah-/- mouse liver nuclei contained significantly more SREBP1 than did Aoah+/+ mouse liver nuclei (Figure 5C).
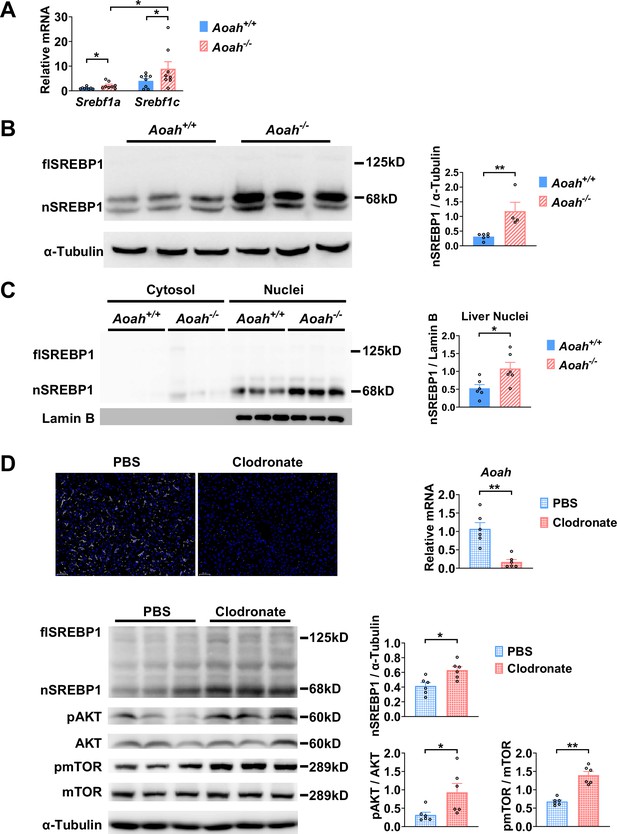
Acyloxyacyl hydrolase (AOAH) reduces hepatic SREBP1.
(A) Srebf1a and Srebf1c gene mRNA was measured in livers from Aoah+/+ and Aoah-/- mice. n=8, each symbol represents one mouse. (B) Liver homogenates from Aoah+/+ and Aoah-/- mice were subjected to western analysis. SREBP1 protein levels were quantitated using ImageJ. flSREBP1 is full-length SREBP1, which is a precursor; nSREBP1is nuclear SREBP1, which is an active form. n=4–6, each symbol represents one mouse. (C) Liver cytosol and nuclei were separated from Aoah+/+ and Aoah-/- mice and then subjected to western analysis. SREBP1 protein levels were quantitated using ImageJ. n=6, each symbol represents one mouse. (D) Aoah+/+ mice were injected i.v. with 200 μl clodronate-liposomes or PBS-liposomes. After 2 days, livers were dissected, sectioned, and stained with anti-F4/80 antibody (white) and DAPI (blue). AOAH mRNA was measured in livers. SREBP1, pAKT, AKT, pmTOR, and mTOR were measured using western blotting. n=6, each symbol represents one mouse. Mann–Whitney test was used. *p<0.05; **p<0.01.
-
Figure 5—source data 1
Original tiff files of western blots for Figure 5B–D.
- https://cdn.elifesciences.org/articles/100731/elife-100731-fig5-data1-v1.zip
-
Figure 5—source data 2
Original tiff files containing uncropped western blots with labeling for Figure 5B–D.
- https://cdn.elifesciences.org/articles/100731/elife-100731-fig5-data2-v1.zip
When we analyzed the transcript profiles from co-housed 6–8-week-old Aoah+/+ and Aoah-/- mouse livers, we noticed that the expression of inflammatory genes for serum amyloid A1 (Saa1), Saa2, and Saa3 was significantly higher in Aoah-/- mouse livers (Figure 5—figure supplement 1A). We isolated hepatocytes from Aoah+/+ and Aoah-/- mice and found that Aoah-/- mouse hepatocytes expressed increased levels of Saa1, Saa2, Saa3, and inflammation regulatory Irak3 mRNA (Figure 5—figure supplement 1B). Purified hepatocytes from Aoah-/- mice had increased levels of Acly, Acaca, Acacb, Fasn, and Cd36 mRNA (Figure 5—figure supplement 1C and D) and decreased levels of Acot2 and Ppara mRNA (Figure 5—figure supplement 1E), changes that may contribute to lipid accumulation as mice grow older. Thus, in young mice, even before hepatic lipid accumulation can be observed, Aoah-/- mouse hepatocytes have altered expression of genes that may promote lipid storage.
We next used clodronate-liposomes to deplete Kupffer cells and found that clodronate-liposome treatment diminished liver Aoah mRNA, confirming that Kupffer cells are the major source of hepatic AOAH (Shao et al., 2007; Figure 5D). Notably, after clodronate liposome treatment, nSREBP1 levels increased in the liver significantly, resembling Aoah-/- mice (Figure 5D). AKT-mTOR1-p70 S6-kinase (S6K) activation induces SREBP1c processing in hepatocytes (Jeon et al., 2023; Owen et al., 2012; Yecies et al., 2011). Livers from clodronate-liposome-treated mice had increased AKT and mTOR activation (Figure 5D), suggesting that when gut-derived LPS cannot be inactivated by AOAH in the liver, bioactive LPS stimulates the mTOR pathway and induces SREBP1 activation.
Excessive gut-derived LPS increases hepatic nSREBP1 and mTOR activation
To find out if excessive gut LPS increases liver LPS levels and promotes fatty acid synthesis gene expression, we orally gavaged Aoah+/+ mice with LPS. We confirmed that orally gavaged (i.g.) LPS increased hepatic LPS levels in Aoah+/+ mice (Figure 6A). Like Aoah-/- mice, Aoah+/+ mice that received gavaged LPS had increased levels of Srebf1a, Srebf1c, Pcx, Acaca, Acacb, Fasn, Scd1, and Cd36 mRNA in their livers (Figure 6B and C). LPS administered i.g. also increased nSREBP1 in Aoah+/+ mouse livers (Figure 6D). Consistently, elevated AKT-mTOR-S6K activation was found in Aoah-/- mouse livers, and when we gave Aoah+/+ mice i.g. LPS, hepatic AKT-mTOR-S6K activity increased (Figure 6D). To find out whether LPS can directly stimulate hepatocytes to induce SREBP1 activation, we isolated primary hepatocytes and found that LPS stimulated mTOR activation and nSREBP1 upregulation (Figure 6E). Adding purified Kupffer cells to the hepatocyte culture did not further increase SREBP1 activation, suggesting that LPS directly acts on hepatocytes (Yu et al., 2021), at least in vitro. Blocking mTOR activation using torin1 prevented LPS-induced nSREBP1 upregulation (Figure 6E), suggesting that upon LPS stimulation SREBP1 activation depends upon the mTOR pathway. Collectively, these results confirm that excessive hepatic LPS derived from the Aoah-/- mouse intestine induces hepatocyte mTOR activity, which increases nSREBP1 abundance; AOAH prevents hepatic lipid accumulation by inactivating gut-derived LPS.
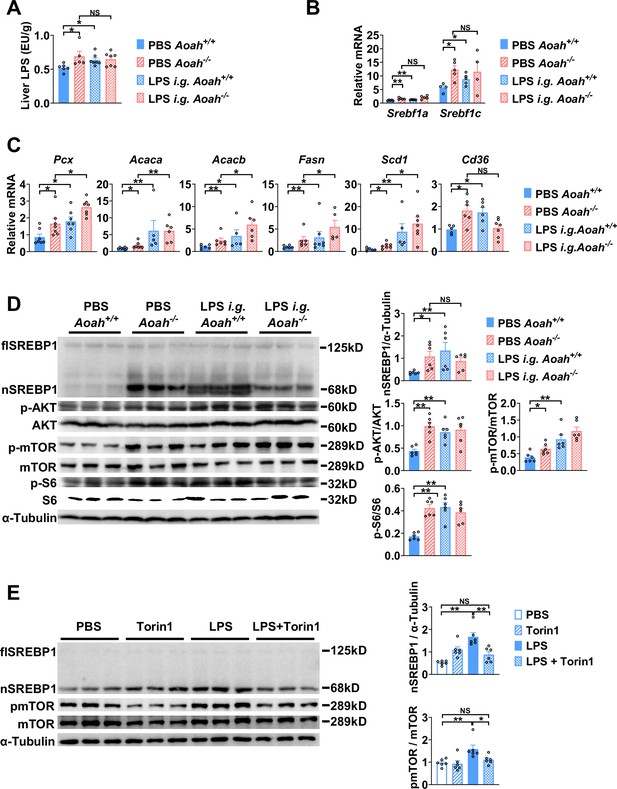
Excessive gut-derived lipopolysaccharides (LPS) increases hepatic nSREBP1 and mTOR activation.
Livers from Aoah+/+ mice, Aoah-/- mice, and Aoah+/+ mice that were orally gavaged (i.g.) with 200 μg LPS 24 h earlier were obtained. (A) Hepatic LPS levels were measured. (B) Srebf1a and Srebf1c mRNA was measured in livers. (C) The mRNA levels of fatty acid biosynthesis-related genes and Cd36 were measured using qPCR. (A–C) Data were combined from two experiments, n=5–7, each symbol represents one mouse. (D) SREBP1 protein levels and AKT-mTOR activities were measured using western and ImageJ. Livers from Aoah-/- mice and Aoah+/+ mice that were orally gavaged (i.g.) with 200 μg LPS 24 h earlier had higher nSREBP1 and mTOR activities than did those from control Aoah+/+ mice. Data were combined from two experiments, n=6, each symbol represents one mouse. (E) Primary hepatocytes were isolated from co-housed 6–8-week-old Aoah+/+ mice and treated with 10 ng/ml LPS with or without 100 nM Torin1 for 6 h. Cells were lysed for western analysis. Data were combined from two experiments, n=6. Mann–Whitney test was used. *p<0.05; **p<0.01; ***p<0.001.
-
Figure 6—source data 1
Original tiff files of western blots for Figure 6D and E.
- https://cdn.elifesciences.org/articles/100731/elife-100731-fig6-data1-v1.zip
-
Figure 6—source data 2
Original tiff files containing uncropped western blots with labeling for Figure 6D and E.
- https://cdn.elifesciences.org/articles/100731/elife-100731-fig6-data2-v1.zip
Discussion
The intestine and liver are connected by the portal vein, enabling the transport of gut commensal-derived molecules, including Gram-negative bacterial LPS, to the liver (Albillos et al., 2020; Leung et al., 2016). Much evidence suggests that gut-derived LPS induces hepatic inflammation and therefore exacerbates MASLD, especially when dysbiosis and intestinal barrier dysfunction have occurred (An et al., 2022; Aron-Wisnewsky et al., 2020a; Aron-Wisnewsky et al., 2020b; Leung et al., 2016). As lipid accumulation in hepatocytes is considered to be the first hit, gut-derived LPS is usually thought to be the second hit in the pathogenesis of MASLD, mainly inducing inflammation (An et al., 2022), yet the possibility that LPS also has direct effects on hepatocyte lipid metabolism has received little attention. In previous studies, we found that when we co-housed Aoah+/+ and Aoah-/- mice for three or more weeks, they had similar microbiota (Qian et al., 2018), yet we found significantly more LPS in Aoah-/- mouse feces and livers. In this study, we found that Aoah-/- mice accumulated more hepatic fat than did Aoah+/+ mice when the mice were fed either NC or an HFD. Aoah-/- mouse livers also expressed more inflammation-inducing and pro-fibrosis genes and had more liver damage when they were fed an HFD. Notably, when Aoah-/- mice were young and had not developed MASLD, their livers already expressed significantly elevated levels of nSREBP1 and its target genes (Figure 7).
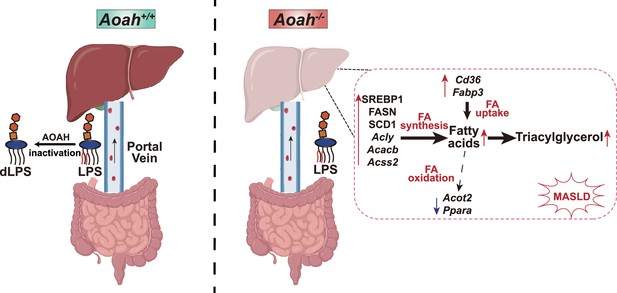
Acyloxyacyl hydrolase (AOAH) prevents metabolic dysfunction-associated steatotic liver disease (MASLD) by inactivating gut-derived lipopolysaccharides (LPS).
Gut-derived LPS may translocate via the portal vein to the liver. In Aoah+/+ mice, LPS can be deacylated by AOAH in the intestine and portal venous blood; when intact LPS reaches the liver, it can be inactivated by hepatic AOAH. In Aoah-/- mice, gut-derived LPS remains able to stimulate fat accumulation (steatosis) in the liver. LPS stimulates hepatocytes to generate nuclear SREBP1, which promotes fatty acid biosynthesis gene expression. LPS also increases the expression of fatty acid uptake genes Cd36 and Fabp3 while reducing that of fatty acid oxidation-related genes, Acot2 and Ppara. Persistent LPS stimulation renders Aoah-/- mice more likely to develop MASLD than are Aoah+/+ mice. dLPS = deacylated LPS.
Liver is an important tissue that converts carbohydrates into lipids. SREBP1c, the predominant isoform expressed in the liver, plays an important role in fatty acid synthesis (Shimano and Sato, 2017). SREBP1c mRNA was elevated in MASLD patient livers (Kohjima et al., 2008) and its chronic activation contributed to MASLD progression (Kawano and Cohen, 2013) SREBP1 has become a target for MASLD treatment (Ju et al., 2020; Jump et al., 2013). Intriguingly, nSREBP1 levels increased in the livers of 6–8-week-old (i.e., young) Aoah-/- mice before MASLD developed; the expression of many SREBP1 target genes, such as those involved in fatty acid biosynthesis (Acly, Acaca, Acacb, Fasn, Scd1, Acss2), also increased. In addition, Srebf1a and Srebf1c mRNA both increased in Aoah-/- mouse livers, suggesting that regulation occurs at the transcription level or, because the Srebf1 gene (encoding SREBP1) promoter contains SREs, increased nSREBP1 induced a feed-forward transcription of SREBP1 (DeBose-Boyd and Ye, 2018). We found that orally gavaged LPS increased hepatic LPS, nSREBP1 abundance, and the expression of nSREBP1’s target genes in Aoah+/+ mice, suggesting that gut-derived LPS reaches the liver and promotes fatty acid synthesis. Thus, our data suggest that when AOAH is lacking, excessive gut-derived LPS stimulates SREBP1 activation to promote de novo lipogenesis in the liver, contributing to more severe MASLD.
Notably, AKT-mTOR-S6K activity increased in Aoah-/- mouse livers; it may contribute to increased SREBP1 translocation and processing (DeBose-Boyd and Ye, 2018; Jeon et al., 2023; Owen et al., 2012). When we isolated primary mouse hepatocytes and stimulated them with LPS in vitro, we found that nSREBP1 upregulation was induced in an mTOR-dependent manner, suggesting that LPS stimulates hepatocyte directly to promote mTOR activation, which induces lipid accumulation.
In addition to fatty acid biosynthesis gene expression, the expression of Cd36, which is involved in free fatty acid uptake (Chen et al., 2022), also increased in the livers of young Aoah-/- mice while the expression of fatty acid oxidation-related genes Acot2 and Ppara decreased (Bougarne et al., 2018; Moffat et al., 2014). In addition to taking up fatty acids, CD36 interacts with INSIG2, a negative regulator of SREBP1, promoting the translocation of SREBP1 from ER to Golgi for cleavage and activation (Zeng et al., 2022). In keeping with our findings, Kim et al. found that LPS suppressed PPAR-α expression via ERK activation and HNF4 phosphorylation in primary mouse hepatocyte culture (Kim et al., 2024). In addition to promoting hepatic FA oxidation, PPAR-α is a transactivating factor that enhances Insig2 expression in hepatocytes, preventing SREBP activation (Lee et al., 2017). These results suggest that hepatic LPS stimulation promotes lipid accumulation via many mechanisms. In a previous study, Huang et al. found that LPS and other TLR agonists promoted fat retention in murine macrophages by increasing TAG synthesis and reducing lipolysis, yet fatty acid synthesis gene abundance did not change (Huang et al., 2014). In contrast, we found that Aoah-/- and Aoah+/+ mouse livers had similar levels of Acsl1, Dgat2, and Pnpla2 mRNAs, suggesting that in response to LPS or other PAMPs, hepatocytes and macrophages may accumulate fat via different mechanisms. Notably, we found previously that after LPS stimulation of macrophages in vitro, the culture medium became acidic due to aerobic glycolysis (the Warburg effect). The acidic environment contributed more to increasing fat accumulation than did LPS stimulation (Lu et al., 2014). A sensitive pH indicator is needed to find out if the blood and/or extracellular fluid in the liver become acidic due to excessive LPS stimulation and if the acidity promotes hepatic fat accumulation.
In addition to LPS, AOAH also deacylates and inactivates oxidized phospholipids and lysophospholipids (Zou et al., 2021), Danger-associated molecular pattern (DAMP) molecules that are induced by inflammation and known to contribute to MASLD (Sun et al., 2020; Wang et al., 2022). By inactivating gut-derived PAMPs and DAMPs, AOAH may decrease hepatic fat accumulation and prevent MASLD. Increasing AOAH abundance may be a useful way to prevent and/or reduce this common disease.
Materials and methods
Mice
C57BL/6J Aoah-/- mice were produced at the University of Texas Southwestern Medical Center, Dallas, Texas, (Lu et al., 2003), transferred to the National Institutes of Health, Bethesda, Maryland, USA, and then provided to Fudan University, Shanghai, China. The mutated Aoah gene had been backcrossed to C57BL/6J mice for at least 10 generations. Aoah+/+ and Aoah-/- male mice were housed in a specific pathogen-free facility with 12 h light/dark cycle at 22°C at the Fudan University Experimental Animal Center (Shanghai, China). Mice were randomly assigned to each experimental group. Aoah+/+ and Aoah-/- male mice were co-housed for at least 3 weeks before the start and throughout the experiments. Co-housed Aoah+/+ and Aoah-/- mice were processed and analyzed blindly. All studies used protocols approved by the Institutional Animal Care and Use Committee (IACUC) of Fudan University (2023-DWYY-03JZS). All animal study protocols adhered to the Guide for the Care and Use of Laboratory Animals.
MASLD mouse model
Request a detailed protocolCo-housed Aoah+/+ and Aoah-/- male mice were fed either an NC or a high-fat calorie diet (D12492, Research Diets, USA) that contained protein:carbohydrate:fat (20:20:60, kcal%) plus high fructose (23.1 g/l; F3510, Sigma, USA) and glucose (18.9 g/l; G8270, Sigma) in the drinking water (HFD) for 28 weeks (Liu et al., 2018).
Blood analysis
Request a detailed protocolTotal TAG, TCHO, LDL, HDL, AST, and ALT in mouse serum were measured at the Department of Laboratory Animal Science, Fudan University, using ADVIA Chemistry XPT.
Liver histology
Request a detailed protocolAfter livers were excised and fixed in 4% paraformaldehyde for 18 h, they were sectioned and stained with H&E and Oil Red O. The samples were examined for steatosis, hepatocyte ballooning degeneration, lobular inflammation, and lipid droplets using a Nikon E200 microscope.
MASLD activity score
Request a detailed protocolHistological analysis of the liver was performed based on the Sheka MASLD scoring criteria (Sheka et al., 2020). Liver steatosis is an infiltration of hepatic fat with minimal inflammation and is graded based on the fat percentage in hepatocytes: grade 0 (< 5%), grade 1 (5–33%), grade 2 (33–66%), and grade 3 (>66%). Inflammatory activity is manifested by two factors: grade 0 (no inflammation), grade 1 (<2 foci per 200× field), grade 2 (2–4 foci per 200× field), grade 3 (>4 foci per 200× field), and the presence of hepatocyte ballooning degeneration: no ballooned cells (grade 0), a few ballooned cells (grade 1), and many ballooned cells (grade 2).
Liver lipid analysis
Request a detailed protocolUndiluted serum samples and liver homogenates (50 mg/ml) were run in duplicate alongside a standard curve of glycerol (triglyceride assay), cholesterol (cholesterol assay), or palmitic acid (free fatty acid assay) according to the manufacturer’s instructions. Triglyceride Determination Kit and Cholesterol Determination Kit were obtained from Applygen. Free Fatty Acid Quantitation Kit was purchased from Sigma-Aldrich.
Real-time PCR (qPCR)
Request a detailed protocolRNA from livers or isolated hepatocytes was purified using TRNzol Universal Reagent (Tiangen) and reversely transcribed (Tiangen). The primers used for qPCR are listed in Supplementary file 1. Actb was used as an internal control, and the relative gene expression was calculated using the ΔΔCt quantification method.
Liver immune cell isolation
Request a detailed protocolAfter mice were exsanguinated, 2 ml PBS containing collagenase (5 mg/ml, type IV, Sigma) were injected into the liver via the inferior vena cava. The liver was cut into small pieces and treated with collagenase (0.5 mg/ml) for 10 min at 37°C. The liver pieces were mashed using syringe plungers. The cells were passed through a 70 μm strainer (WHB scientific) and then centrifuged at 50 × g for 3 min, three times, to pellet hepatocytes. The immune cells in the supernatant were pelleted (500 × g for 15 min) and then isolated on a 40% Percoll step gradient (Cytiva). The cells were then stained and analyzed using flow cytometry (Shao et al., 2007).
Flow cytometry
Request a detailed protocolLiver cells were collected by centrifugation and then incubated with Fc blocking antibody (purified anti-mouse CD16/32, BioLegend, AB_2262724) on ice for 15 min. After the cells were stained with fluorescence-conjugated antibodies for 30 min on ice, they were washed and subjected to FACS (BD, FACSCelesta). The FACS data were analyzed using FlowJo software (TreeStar, Inc). All antibodies used for flow cytometry were anti-mouse antigens. Anti-mouse antibodies used for flow cytometry were anti-CD45-BV785 (Clone 30-F11, BioLegend, AB_2564590), anti-CD11b-FITC (Clone M1/70, BioLegend, AB_312788), anti-F4/80-BV421 (Clone BM8, BioLegend, AB_2563102), anti-Ly6G-PerCP-Cy5.5 (Clone 1A8, BioLegend, AB_1877271), anti-Ly6C-APC-Cy7 (Clone HK1.4, BioLegend, AB_10643867), anti-MHC II-PE-Cy7 (Clone M5/114.15.2, BioLegend, AB_2069376), anti-VSIG4-APC (Clone NLA14, eBioscience, AB_2637428), and anti-Tim4-PE (Clone RMT4-54, BioLegend, AB_1227807).
Hepatocyte isolation
Request a detailed protocolPrimary hepatocytes were isolated from adult mice using a two-step collagenase perfusion method (Charni-Natan and Goldstein, 2020). In brief, the peritoneal cavity was opened and the liver was perfused in situ via the portal vein at 37°C with 20 ml PBS followed by 20 ml DMEM containing 10 mg collagenase (type IV, Sigma). The liver was then removed and gently minced, and the released cells were dispersed in DMEM containing 5% fetal bovine serum and 1% penicillin/streptomycin. The solution containing the mixed cells and debris was passed through a 100 µm cell strainer, and then centrifuged at 50 × g for 3 min, twice. The hepatocytes were pelleted and then isolated on a 90% Percoll step gradient (Cytiva). Hepatocytes were then resuspended in a TRNzol Universal Reagent (Tiangen) to measure mRNA.
Western blot
Request a detailed protocolSmall pieces of liver were lysed with RIPA buffer (Biyotime) containing 1 mM PMSF (Biyotime) and a proteinase inhibitor mixture (Selleck). A commercial cytosol and nucleus Protein Extraction Kit (P0027, Beyotime) was used to separate cytosolic and nuclear proteins in the liver. The following antibodies were used for western analysis: anti-SREBP1 (SC-17755, Santa Cruz, AB_628283), anti-AOAH (HPA021666, Sigma-Aldrich, AB_3678739), anti-Lamin B1 (12987-1-AP, Proteintech, AB_2136290), anti-SCD1 (ab39969, Abcam, AB_945374), anti-FASN (SC-48357, Santa Cruz, AB_627584), anti-Phospho-mTOR (Ser2448) (5536, Cell Signaling Technology, AB_10691552), anti-Phospho-p70 S6 Ribosomal Protein (Thr389) (9234, Cell Signaling Technology, AB_2269803), anti-Phospho-AKT (Ser473) (4060, Cell Signaling Technology, AB_2315049), anti-mTOR (2983, Cell Signaling Technology, AB_2105622), anti-p70 S6 Ribosomal Protein (2217, Cell Signaling Technology, AB_331355), anti-AKT (4691, Cell Signaling Technology, AB_915783), and anti-α-Tublin (HRP-66031; Proteintech, AB_2687491). Anti-mouse IgG (7076S; Cell Signaling Technology, AB_330924) and anti-rabbit IgG (7074S, Cell Signaling Technology, AB_2099233) were used as secondary antibodies. ECL substrate (Bio-Rad Diagnostic) was used to detect proteins in western blotting, and the blot bands were quantified using ImageJ.
Measurement of TLR4-stimulating activities in mouse feces, liver, and plasma
Request a detailed protocolFresh feces were collected and resuspended in endotoxin-free PBS (0.1 g/ml) and centrifuged at 800 × g for 5 min. The supernatant was heated at 70°C for 10 min. Mice were bled from the eye socket; 5 μl of 0.5 M EDTA was used as an anticoagulant. Livers were homogenized in PBS, centrifuged, and the supernatant was obtained. In some experiments, 200 µg of LPS was resuspended in 200 µl of PBS and then the suspension was slowly administered into the esophagus of mice using a gavage needle. Twenty-four hours later, the livers were collected for analysis. All the samples were collected for TLR4-stimulating activity using a cell-based colorimetric endotoxin detection kit (HEK-Blue LPS Detection Kit2, InvivoGen). Diluted samples were added to human embryonic kidney (HEK-293) cells that expressed hTLR4 and an NF-κB-inducible secreted embryonic alkaline phosphatase reporter gene. After 18 h incubation, cell culture media were applied to QUANTI-Blue medium to measure alkaline phosphatase activity. A preparation of E. coli 055:B5 LPS, standardized to FDA-approved control standard endotoxin, which was included in the kit, was used to quantitate TLR4-stimulating activity. Plates were read at a wavelength of 620 nm (Tecan).
Gut permeability analysis
Request a detailed protocolAfter mice were fasted for 18 h, they were orally gavaged with fluorescein isothiocyanate (FITC)-conjugated 4 kDa dextran (50 mg per 100 g body weight) (46944, Sigma-Aldrich). Four hours after gavage, blood was collected from the facial vein and the serum was used for FITC fluorescence measurements (excitation, 490 nm; emission, 520 nm).
RNA-sequencing analysis
Request a detailed protocolTotal RNA was isolated using TRNzol from co-housed 6–8-week-old Aoah+/+ and Aoah-/- mouse livers. The libraries were sequenced on an Ilumina Novaseq 6000 platform and 150 bp paired-end reads were generated. Differential expression analysis was performed using the DESeq2. Q value <0.05 and foldchange >1.5 were set as the threshold for significantly differential gene expression. GSEA was performed using GSEA software. RNA-seq analysis was conducted by Shanghai OE Biotech. Co, Ltd., China, and the results were deposited at PRJNA1022016.
Single-cell RNA sequencing analysis
Request a detailed protocolAOAH expression in mouse hepatic cells was analyzed based on single-cell RNA-seq data using the Liver Cell Atlas at https://www.livercellatlas.org/ (Remmerie et al., 2020). Liver single-cell RNA sequencing data from MASLD-cirrhosis patients (n=5) and healthy controls (n=5) were obtained from GSE136103 (Ramachandran et al., 2019). R package Seurat (version 5.1.0) was utilized for clustering and cell-type identification.
Kupffer cell deletion
Request a detailed protocolKupffer cells were depleted by injecting 200 μl Clodronate-liposomes (5 mg/ml, Liposoma BV) i.v. and PBS-liposomes were used as controls. Two days after injection, Kupffer cell deletion was confirmed by staining F4/80+ cells in cryostat liver sections.
Statistical analysis
Request a detailed protocolData are presented as mean ± SEM. Differences between groups were analyzed using Mann–Whitney test. To compare kinetic difference, two-way ANOVA was used. The statistical significance was set at p<0.05. *p<0.05, **p<0.01, and ***p<0.001.
Data availability
The RNA-seq data were deposited at PRJNA1022016.
-
NCBI BioProjectID PRJNA1022016. Mouse liver RNA-seq.
-
NCBI Gene Expression OmnibusID GSE192742. Spatial proteogenomics reveals distinct and evolutionarily-conserved hepatic macrophage niches.
-
NCBI Gene Expression OmnibusID GSE136103. Resolving the fibrotic niche of human liver cirrhosis using single-cell transcriptomics.
References
-
The gut-liver axis in liver disease: Pathophysiological basis for therapyJournal of Hepatology 72:558–577.https://doi.org/10.1016/j.jhep.2019.10.003
-
The role of gut-derived lipopolysaccharides and the intestinal barrier in fatty liver diseasesJournal of Gastrointestinal Surgery 26:671–683.https://doi.org/10.1007/s11605-021-05188-7
-
Gut microbiota and human NAFLD: disentangling microbial signatures from metabolic disordersNature Reviews. Gastroenterology & Hepatology 17:279–297.https://doi.org/10.1038/s41575-020-0269-9
-
Molecular actions of pparα in lipid metabolism and inflammationEndocrine Reviews 39:760–802.https://doi.org/10.1210/er.2018-00064
-
Protocol for primary mouse hepatocyte isolationSTAR Protocols 1:100086.https://doi.org/10.1016/j.xpro.2020.100086
-
CD36, a signaling receptor and fatty acid transporter that regulates immune cell metabolism and fateThe Journal of Experimental Medicine 219:e20211314.https://doi.org/10.1084/jem.20211314
-
SREBPs in lipid metabolism, insulin signaling, and beyondTren lipid metabolism, insulin signaling, and bnds in Biochemical Sciences 43:358–368.https://doi.org/10.1016/j.tibs.2018.01.005
-
Cause, pathogenesis, and treatment of nonalcoholic steatohepatitisThe New England Journal of Medicine 377:2063–2072.https://doi.org/10.1056/NEJMra1503519
-
New trends on obesity and NAFLD in AsiaJournal of Hepatology 67:862–873.https://doi.org/10.1016/j.jhep.2017.06.003
-
Mechanisms of NAFLD development and therapeutic strategiesNature Medicine 24:908–922.https://doi.org/10.1038/s41591-018-0104-9
-
SREBPs: activators of the complete program of cholesterol and fatty acid synthesis in the liverJournal of Clinical Investigation 109:1125–1131.https://doi.org/10.1172/JCI0215593
-
Toll-like receptor agonists promote prolonged triglyceride storage in macrophagesThe Journal of Biological Chemistry 289:3001–3012.https://doi.org/10.1074/jbc.M113.524587
-
Physiological and pathological roles of lipogenesisNature Metabolism 5:735–759.https://doi.org/10.1038/s42255-023-00786-y
-
Fatty acid-regulated transcription factors in the liverAnnual Review of Nutrition 33:249–269.https://doi.org/10.1146/annurev-nutr-071812-161139
-
Mechanisms of hepatic triglyceride accumulation in non-alcoholic fatty liver diseaseJournal of Gastroenterology 48:434–441.https://doi.org/10.1007/s00535-013-0758-5
-
The role of macrophages in nonalcoholic fatty liver disease and nonalcoholic steatohepatitisNature Reviews. Gastroenterology & Hepatology 16:145–159.https://doi.org/10.1038/s41575-018-0082-x
-
Molecular and cellular mechanisms of liver fibrosis and its regressionNature Reviews. Gastroenterology & Hepatology 18:151–166.https://doi.org/10.1038/s41575-020-00372-7
-
SREBP-1c, regulated by the insulin and AMPK signaling pathways, plays a role in nonalcoholic fatty liver diseaseInternational Journal of Molecular Medicine 21:507–511.
-
The role of the gut microbiota in NAFLDNature Reviews. Gastroenterology & Hepatology 13:412–425.https://doi.org/10.1038/nrgastro.2016.85
-
Characterization of a murine nonalcoholic steatohepatitis model induced by high fat high calorie diet plus fructose and glucose in drinking waterLaboratory Investigation; a Journal of Technical Methods and Pathology 98:1184–1199.https://doi.org/10.1038/s41374-018-0074-z
-
Stimulus-dependent deacylation of bacterial lipopolysaccharide by dendritic cellsThe Journal of Experimental Medicine 197:1745–1754.https://doi.org/10.1084/jem.20030420
-
Prolonged triglyceride storage in macrophages: pHo Trumps pO2 and TLR4The Journal of Immunology 193:1392–1397.https://doi.org/10.4049/jimmunol.1400886
-
Acyl-CoA thioesterase-2 facilitates mitochondrial fatty acid oxidation in the liverJournal of Lipid Research 55:2458–2470.https://doi.org/10.1194/jlr.M046961
-
Biochemical transformation of bacterial lipopolysaccharides by acyloxyacyl hydrolase reduces host injury and promotes recoveryThe Journal of Biological Chemistry 295:17842–17851.https://doi.org/10.1074/jbc.REV120.015254
-
BookConstitutive Overexpression of Acyloxyacyl Hydrolase in Mus musculusThe University of Texas Southwestern Medical Center at Dallas.
-
Overproduction of acyloxyacyl hydrolase by macrophages and dendritic cells prevents prolonged reactions to bacterial lipopolysaccharide in vivoThe Journal of Infectious Diseases 200:1685–1693.https://doi.org/10.1086/646616
-
LPS inactivation by a host lipase allows lung epithelial cell sensitization for allergic asthmaThe Journal of Experimental Medicine 215:2397–2412.https://doi.org/10.1084/jem.20172225
-
A host lipase detoxifies bacterial lipopolysaccharides in the liver and spleenThe Journal of Biological Chemistry 282:13726–13735.https://doi.org/10.1074/jbc.M609462200
-
SREBP-regulated lipid metabolism: convergent physiology - divergent pathophysiologyNature Reviews. Endocrinology 13:710–730.https://doi.org/10.1038/nrendo.2017.91
-
Lipopolysaccharides in liver injury: molecular mechanisms of Kupffer cell activationAmerican Journal of Physiology-Gastrointestinal and Liver Physiology 283:G256–G265.https://doi.org/10.1152/ajpgi.00550.2001
-
SREBP-1, a basic-helix-loop-helix-leucine zipper protein that controls transcription of the low density lipoprotein receptor geneCell 75:187–197.
-
Global burden of NAFLD and NASH: trends, predictions, risk factors and preventionNature Reviews. Gastroenterology & Hepatology 15:11–20.https://doi.org/10.1038/nrgastro.2017.109
-
Hepatocyte TLR4 triggers inter-hepatocyte Jagged1/Notch signaling to determine NASH-induced fibrosisScience Translational Medicine 13:eabe1692.https://doi.org/10.1126/scitranslmed.abe1692
Article and author information
Author details
Funding
National Natural Science Foundation of China (32370979)
- Mingfang Lu
National Natural Science Foundation of China (32170929)
- Mingfang Lu
National Natural Science Foundation of China (91742104)
- Mingfang Lu
National Natural Science Foundation of China (31770993)
- Mingfang Lu
National Natural Science Foundation of China (31570910)
- Mingfang Lu
National Natural Science Foundation of China (32260189)
- Wei Jiang
National Natural Science Foundation of China (32300772)
- Wei Jiang
National Natural Science Foundation of China (82301980)
- Benkun Zou
Shanghai Committee of Science and Technology (21ZR1405400)
- Mingfang Lu
Guizhou Provincial Science and Technology Department (ZK2024 - 240)
- Wei Jiang
Science and Technology Program of Guizhou Province (gzwkj2023-568)
- Wei Jiang
National Institutes of Health (AI18188)
- Robert S Munford
National Institutes of Health (AI44642)
- Robert S Munford
The funders had no role in study design, data collection and interpretation, or the decision to submit the work for publication.
Acknowledgements
This work was supported by the National Natural Science Foundation of China (32370979, 32170929, 91742104, 31770993, and 31570910 to ML, 32260189, 32300772 to WJ, 82301980 to BZ), the Shanghai Committee of Science and Technology (21ZR1405400 to ML), Guizhou Provincial Science and Technology Projects (ZK2024-240 to WJ), Science and Technology Program of the Health Commission of Guizhou Province (gzwkj2023-568 to WJ), and National Institutes of Health (AI18188 and AI44642 to RM).
Ethics
All studies used protocols approved by the Institutional Animal Care and Use Committee (IACUC) of Fudan University (2023-DWYY-03JZS). All animal study protocols adhered to the Guide for the Care and Use of Laboratory Animals.
Version history
- Sent for peer review:
- Preprint posted:
- Reviewed Preprint version 1:
- Reviewed Preprint version 2:
- Version of Record published:
Cite all versions
You can cite all versions using the DOI https://doi.org/10.7554/eLife.100731. This DOI represents all versions, and will always resolve to the latest one.
Copyright
This is an open-access article, free of all copyright, and may be freely reproduced, distributed, transmitted, modified, built upon, or otherwise used by anyone for any lawful purpose. The work is made available under the Creative Commons CC0 public domain dedication.
Metrics
-
- 576
- views
-
- 49
- downloads
-
- 0
- citations
Views, downloads and citations are aggregated across all versions of this paper published by eLife.