Inhibiting poly(ADP-ribosylation) improves axon regeneration
Abstract
The ability of a neuron to regenerate its axon after injury depends in part on its intrinsic regenerative potential. Here, we identify novel intrinsic regulators of axon regeneration: poly(ADP-ribose) glycohodrolases (PARGs) and poly(ADP-ribose) polymerases (PARPs). PARGs, which remove poly(ADP-ribose) from proteins, act in injured C. elegans GABA motor neurons to enhance axon regeneration. PARG expression is regulated by DLK signaling, and PARGs mediate DLK function in enhancing axon regeneration. Conversely, PARPs, which add poly(ADP-ribose) to proteins, inhibit axon regeneration of both C. elegans GABA neurons and mammalian cortical neurons. Furthermore, chemical PARP inhibitors improve axon regeneration when administered after injury. Our results indicate that regulation of poly(ADP-ribose) levels is a critical function of the DLK regeneration pathway, that poly-(ADP ribosylation) inhibits axon regeneration across species, and that chemical inhibition of PARPs can elicit axon regeneration.
https://doi.org/10.7554/eLife.12734.001eLife digest
Neurons carry information around the body along slender projections known as axons. An injury that crushes or cuts an axon can lead to permanent disability if the axon fails to regenerate. While some damaged neurons in the body can repair themselves, typically those present in the brain and spinal cord cannot regenerate successfully after injury.
The ability of a neuron to regenerate its axon depends in part on factors present inside the neuron itself. By understanding how these internal mechanisms regulate axon regeneration, researchers hope to develop new ways to boost the repair of damaged neurons.
A protein called DLK acts inside neurons to promote regeneration of injured axons across a range of species including worms and mammals. In the absence of DLK, regeneration is impaired. The DLK signaling pathway is activated in damaged neurons and is thought to promote repair by altering the activity of genes and proteins that control the regeneration process.
Byrne et al. have now identified genes that are activated by the DLK signaling pathway in the roundworm, Caenorhabditis elegans. The experiments show that DLK signaling increases the activity of genes encoding enzymes known as PARGs, which in turn enhance axon regeneration. PARG enzymes remove chain-like molecules called poly(ADP-ribose) that are attached to target proteins.
Further experiments showed that other enzymes known as PARPs, which add the poly(ADP-ribose) markers to proteins, act to inhibit axon regeneration in both Caenorhabditis elegans and in injured neurons from mice. Consistent with this, Byrne et al. found that drugs that inhibit PARP enzymes improved axon regeneration when they were given to C. elegans with injured neurons. These results suggest that a critical role of the DLK signaling pathway is to regulate poly(ADP-ribose) levels and that reducing the amount of poly(ADP-ribose) added to proteins can promote axon regeneration.
The next step is to understand exactly how poly(ADP-ribose) regulates axon regeneration and to identify the other factors – besides poly(ADP-ribose), PARGs and PARPs – that act downstream of DLK signalling to regulate regeneration.
https://doi.org/10.7554/eLife.12734.002Introduction
Unlike damaged peripheral nerves, the central nervous system does not successfully regenerate after injury. Failure to regenerate has been attributed to two components of the regeneration response: intrinsic and extrinsic factors. While extrinsic inhibitory factors such as the glial microenvironment can be modulated with some success, regeneration potential is still substantially hindered, providing evidence that intrinsic factors play a significant role in modulating the ability of an axon to regenerate (Richardson et al., 1980; Neumann and Woolf, 1999; GrandPré et al., 2000; Fournier et al., 2001; Qiu et al., 2002; Yiu and He, 2006; Park et al., 2008; Smith et al., 2009; Wang et al., 2011). Developing an understanding of the intrinsic mechanisms that regulate regeneration will provide insight into the treatment of neurological injury and disease.
DLK-1 (Dual Leucine Zipper Kinase) is a mitogen activated protein kinase kinase kinase (MAPKKK) identified in C. elegans that functions intrinsically to regulate regeneration of adult axons in the central and peripheral nervous systems across species, including flies and mammals (Hammarlund et al., 2009; Yan et al., 2009; Xiong et al., 2010; Shin et al., 2012; Wang et al., 2013; Watkins et al., 2013; Byrne et al., 2014). Activation of dlk-1 enhances axon regeneration and loss of dlk-1 function inhibits axon regeneration in young and aged animals (Hammarlund et al., 2009; Yan et al., 2009; Byrne et al., 2014). In worms, flies, and mice, the function of DLK signaling in regeneration depends on gene transcription (Xiong et al., 2010; Shin et al., 2012; Yan and Jin, 2012; Watkins et al., 2013; Stone et al., 2014). These data suggest that specific targets of DLK transcriptional regulation may mediate the ability of DLK signaling to promote regeneration. Further, these targets may identify novel aspects of the cell biology of axon regeneration. Finally, modulation of these targets might increase the intrinsic regenerative potential of injured axons.
Results and discussion
To identify targets of DLK transcriptional regulation in neurons, we took advantage of a recently developed method that uses FACS to isolate C. elegans neurons and compare their gene expression profiles (Spencer et al., 2014). We sorted GABA motor neurons from animals with activated DLK signaling (dlk-1(OE), conferred by overexpression of DLK-1L [Hammarlund et al., 2009; Yan and Jin, 2012]) and compared them to wild-type GABA neurons. To control for potential off-target effects of DLK activation, we also analyzed neurons that contained both dlk-1(OE) and a loss of function mutation in pmk-3, the MAP kinase at the end of the canonical DLK signaling pathway (Nakata et al., 2005; Hammarlund et al., 2009; Yan and Jin, 2012). RNA sequencing and analysis suggested the parg genes as candidates for further evaluation. The gene parg-2 (poly(ADP-ribose) glycohydrolase-2) was significantly upregulated in neurons with activated DLK signaling (187-fold upregulated in dlk-1(OE) vs wild type, p<0.01, two-way ANOVA, Bonferroni post-test) (Figure 1A). Further, examination of RNA-Seq results for the parg-2 paralog, parg-1, detected a 2.5-fold increase in the dlk-1(OE) background compared to wild type (p<0.05, two-way ANOVA, Bonferroni post-test, Figure 1A) (See Materials and methods). Elevated expression of parg-1 and parg-2 by DLK signaling depended on the canonical DLK MAP kinase pathway since up-regulation was eliminated in neurons that over-expressed dlk-1 but lacked its downstream effector pmk-3 (Figure 1A). These data suggested that regulation of PARG function might be a major effect of DLK signaling. Overall, up-regulation (>two fold, p<0.05) (See Materials and methods) of gene expression by DLK signaling was observed for only 1.9% of coding genes (386 out of 20,375 protein coding genes assayed); expression of most genes was not affected by DLK signaling. For example, expression levels of the pan-neuronal control gene rgef-1 (a ras nucleotide exchange factor) were not altered in either mutant background (Figure 1A). Together, our data indicate parg expression is regulated by DLK signaling in GABA neurons.
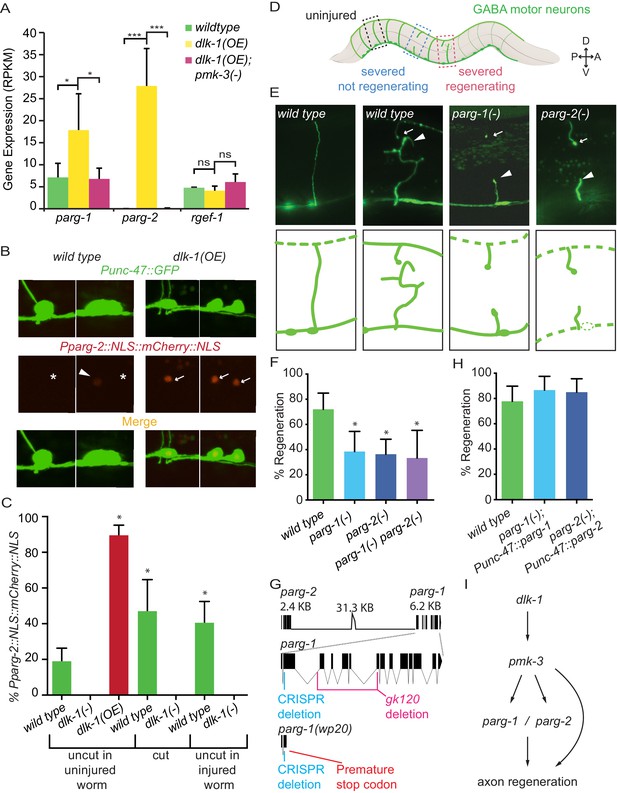
PARG genes regulate axon regeneration.
(A) dlk-1 overexpression upregulates parg-1 and parg-2 expression levels in GABA neurons. The upregulation is suppressed by loss of pmk-3 function. rgef-1 (a pan-neuronal Ras nucleotide exchange factor) expression levels are not affected by manipulations of the dlk-1 pathway (*p<0.05, ***p<0.01, two-way ANOVA, Bonferroni post-test). (B–C) dlk-1 regulates expression of nuclear-localized mCherry driven by the parg-2 promoter. Pparg-2::NLS::mCherry::NLS was observed (arrows) in 90% of nuclei of GABA neurons in dlk-1(OE) animals and in 19% of GABA neurons in wild type animals (asterisks). GABA neurons express GABA neuron-specific GFP marker, Punc-47::GFP. (C) parg-2 expression was significantly increased in both severed axons and neighboring uncut axons relative to axons in uninjured wild type animals. parg-2 was not expressed in dlk-1(lf) axons, whether severed or intact. (*p<0.05, Fisher’s exact test, relative to wild type, n = 111, 20, 115, 34, 18, 69, 36). (D) The GABA motor nervous system of C. elegans. GFP-labeled axons were severed with a pulsed laser at the midline (dark brown line) and scored for regeneration. (E) Representative micrographs of uninjured wild type, severed wild type, severed parg-1(-), and severed parg-2(-) GABA axons. Each carry the oxIs12 transgene which drives GFP expression in GABA neurons. Arrowheads and arrows indicate proximal and distal stumps, respectively. (F) Axon regeneration is significantly reduced in parg-1(-), parg-2(-) and parg-1(-) parg-2(-) mutants compared to wild type animals (*p<0.05, Fisher’s exact test, relative to wild type, n = 50, 39, 67, 21). (G) parg-1 and parg-2 are closely linked on chromosome IV, making construction of a double mutant difficult. To create a double parg-1 parg-2 mutant, parg-1 was mutated with CRISPR in a parg-2(lf) background. The resulting frameshift mutation (wp20) truncates PARP-1 earlier than the canonical gk120 deletion allele. (H) Expression of parg-1 or parg-2 in GABA motor neurons rescued axon regeneration in parg-1(lf) and parg-2(lf) mutants, respectively. (I) Model of PARG function in axon regeneration.
To further test whether DLK regulates parg expression in GABA neurons, we built a reporter construct that expresses nuclear-localized mCherry driven by the parg-2 promoter. At low magnification (4X), mCherry was only detected in dlk-1(OE) animals (20/20 dlk-1OE animals, 0/20 wild type animals). At high magnification (40X), mCherry was seen in 90% of GABA neurons in dlk-1(OE) animals and 19% of GABA neurons in control animals (Figure 1B,C) (p<0.0001, Fisher’s exact test). mCherry was not detected in dlk-1(lf) animals, which lack DLK-1. Therefore, parg-2 expression is dependent on dlk-1, even in intact, uninjured axons.
Next, we tested the effect of axon injury on dlk-1-dependent parg expression (Figure 1B,C). Approximately 10 hr post-axotomy, parg-2 expression was significantly elevated in cut GABA axons relative to parg-2 expression in GABA axons of uninjured animals (47% vs 19%, p=0.0028, Fisher’s exact test). Further, by examining neighboring uncut GABA axons, we found that parg-2 expression also increased in uninjured neurons to equivalent levels (47% vs 41%, p=0.6722, Fisher’s exact test). The increase in parg-2 expression in response to injury is entirely dependent on dlk-1, as parg-2 expression was not seen in cut or uncut axons in injured dlk-1(lf) animals (p=0.0003 and p=0.0001, relative to cut axons and uncut axons in injured wild type animals). Thus, parg-2 expression is upregulated in injured neurons and their neighbors, and dependent on dlk-1.
Poly(ADP-ribose) glycohydrolases (PARGs) catalyze dePARylation: the removal of the post-translational modification poly(ADP-ribose) (PAR) from target proteins (Miwa and Sugimura, 1971; Althaus and Richter, 1987). The parg-1 and parg-2 genes encode the only two PARGs in the C. elegans genome (Gagnon et al., 2002). We determined the function of parg-1 and parg-2 in axon regeneration by assessing regrowth after single neuron laser axotomy in GABA neurons (Byrne et al., 2011) (Figure 1D). Loss of either parg-1 or parg-2 reduced axon regeneration to approximately half of normal levels: only 39% and 36% of axons regenerated in parg-1 and parg-2 mutants, respectively, while 70% of axons regenerated in control animals (Figure 1E,F). Therefore, parg-1 and parg-2 regulate axon regeneration.
The parg-1 and parg-2 genes are closely linked on chromosome IV, complicating generation of a double mutant. To assess whether complete elimination of PARG activity could further reduce regeneration, we used a CRISPR-Cas9 approach (Friedland et al., 2013) to mutate parg-1 in the parg-2(lf) background (Figure 1G). The resulting parg-1(lf); parg-2(lf) double mutant was viable and displayed wild-type morphology and behavior, indicating PARG function is not essential. Axon regeneration in parg-1(lf); parg-2(lf) animals was similar to axon regeneration in either parg-1 or parg-2 mutant animals (Figure 1F). Thus, PARG activity is required for normal axon regeneration, but some regeneration occurs even in animals that completely lack parg.
To test whether the parg genes act within GABA neurons to regulate axon regeneration, we reintroduced parg-1 or parg-2 specifically in GABA neurons (using the unc-47 promoter) of parg-1(lf) or parg-2(lf) mutants, respectively, and assessed regeneration. We found that 87% and 85% of injured axons regenerated in parg-1 and parg-2 worms whose GABA neurons had restored PARG expression (Figure 1H). We conclude cell-intrinsic PARG function is required for axon regeneration of GABA neurons (Figure 1I).
Cellular levels of PARylation are determined by the balance between the activity of PARGs, which remove PAR, and the activity of poly (ADP-ribose) polymerases (PARPs), which transfer PAR onto target proteins (Schreiber et al., 2006; Gibson and Kraus, 2012). Thus, axon regeneration defects in parg-1 and parg-2 mutants (Figure 1F) could be due to accumulation of PAR. To test this hypothesis, we analyzed regeneration in animals with reduced PAR. The C. elegans genome contains two PARP homologs, parp-1 and parp-2 (Gagnon et al., 2002). We found that mutation of either parp-1 or parp-2 increased axon regeneration relative to control animals: 92% and 90% of axons regenerated in parp-1 and parp-2 mutants, respectively, while 76% of axons in controls regenerated (Figure 2A,B). Regenerating axons in these assays include all those that initiate a migrating growth cone after injury. To determine whether axons in PARP mutants are capable of sustained growth toward their original target, we assessed ability to extend towards the dorsal nerve cord (Figure 2C). We found that 56% and 53% of regenerating axons in parp-1 and parp-2 mutants, respectively, regrew at least 3/4 of the distance between the ventral and the dorsal nerve cords compared to only 26% of regenerating axons in controls (Figure 2D). Moreover, some parp-2 mutants sprouted new axons from the cell body (Figure 2E). The opposing effects of PARGs and PARPs on poly(ADP)-ribose and on axon regeneration indicate that PARylation is a critical determinant of regenerative potential.
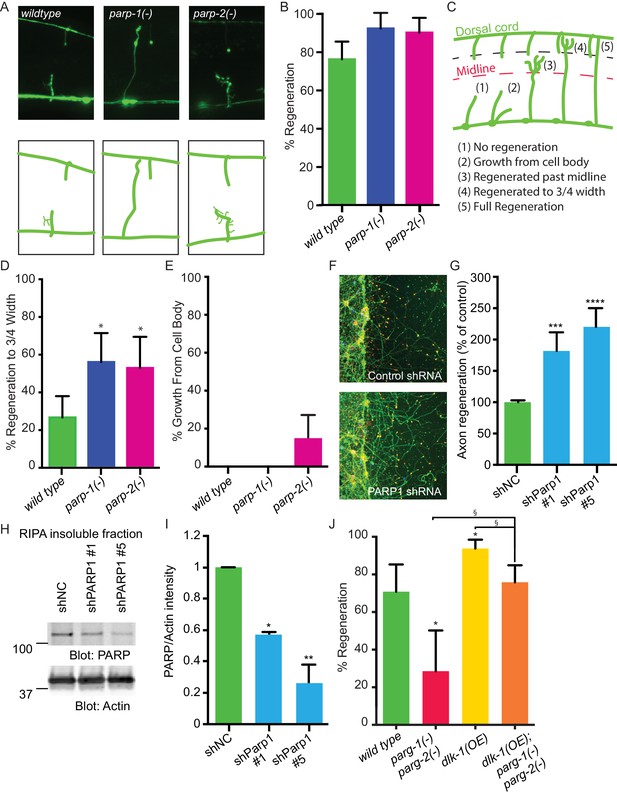
PARPs inhibit axon regeneration.
(A) Representative micrographs of severed wild type, parp-1(lf), and parp-2(lf) GABA motor neurons. (B) Axon regeneration in parp-1(lf) and parp-2(lf) mutants compared to wild type animals (*p<0.05, Fisher’s exact test, n = 84, 26, 61). (C) Cut axons (1) are scored for the distance they extend towards their targets in the dorsal nerve cord (2, 3, 4, 5). (D) Axon regeneration to at least 3/4 of the distance to the dorsal cord (4) is significantly increased in parp-1(lf) and parp-2(lf) mutants relative to wild type animals (*p<0.01, Fisher’s exact test, n = 61, 43, 38). (E) Axon regeneration from the cell body (2) is seen in parp-2(lf) mutants (n = 47, 32, 34). (F) Representative micrographs of injured cortical neurons exposed to negative control shRNA or PARP1 shRNA. (G) Axon regeneration is increased in murine cortical neurons lacking PARP1 (***p<0.001, ****p<0.0001, Anova with Tukey’s multiple comparisons test, n = 108, 8, 8). Axon regeneration was measured in injured cortical neurons exposed to non-coding negative control shRNA (shNC) or either of two unique PARP1 shRNAs. (H, I) Exposure to either shPARP significantly reduced PARP levels in cortical neurons relative to PARP levels in cortical neurons exposed to negative control (shNC) lentivirus (*p<0.05, **p<0.005, Anova with Tukey’s multiple comparisons test). (J) parg-1 and parg-2 loss of function incompletely suppress the increase in regeneration conferred by dlk-1(OE) (*p<0.05, relative to wild type, §p<0.05, relative to dlk-1(OE), Fisher’s exact test, n = 24, 21, 48, 62), indicating the PARGs regulate regeneration downstream of dlk-1 with at least one parallel pathway.
PARG and PARP function are well-conserved between C.elegans and mammals (Gagnon et al., 2002; St-Laurent et al., 2007). Therefore, we hypothesized that blocking PARP function might be sufficient to improve regeneration of mammalian CNS neurons. To test this hypothesis, we assessed the effect of PARP knockdown on mouse cortical neuron regeneration. We cultured primary cortical mouse neurons in 96-well plates (Huebner et al., 2011). We subsequently added lentiviral control or one of two unique PARP1 shRNAs at three days in vitro (DIV), and injured the neurons with a custom pin-replicator five days later (Huebner et al., 2011). Three days after injury, we fixed the neurons and assessed regeneration. We found that axons exposed to PARP1 shRNA regenerated significantly better than axons exposed to control shRNA (Figure 2F,G). To confirm the shRNA clones targeted PARP1, we performed western blots on cortical neurons exposed to the negative control shRNA or to the two unique shRNA that target PARP1. In each case PARP was detected in the insoluble fraction and not in the lysate, in agreement with previously reported localization to the nucleus (reviewed in Bai, 2015). PARP was significantly reduced in neurons exposed to either of the PARP1-targeting shRNAs compared to negative control shRNA (Figure 2H,I). PARP levels were normalized to actin levels in each sample of neurons. Therefore, PARP-1 and PARylation are conserved inhibitors of axon regeneration after injury, and reducing their function improves axon regeneration across species.
Having established that PARGs are novel regulators of axon regeneration, we sought to determine the extent to which DLK function is mediated by PARGs. We assessed regeneration in animals with activated DLK signaling (dlk-1(OE)), but lacking both parg-1 and parg-2. We found that loss of both parg-1 and parg-2 function reduced regeneration in the dlk-1(OE) background, just as loss of parg-1 and parg-2 reduces regeneration in animals with wild type levels of DLK signaling (Figure 2J and Figure 2—figure supplement 1). In both cases, regeneration is reduced but not eliminated, and the amount of regeneration that remains is higher than complete loss of dlk-1 signaling (0% regeneration) or loss of the downstream pmk-3 in the dlk-1(OE) background (7% regeneration) (Nakata et al., 2005; Hammarlund et al., 2009). Thus, DLK-dependent regeneration depends in part on parg-1 and parg-2.
In addition to controlling axon regeneration, DLK signaling regulates presynaptic development (Nakata et al., 2005). To test whether presynaptic development is regulated by PARylation, we quantified synapses at the GABA neuromuscular junction with the pre-synaptic reporter hpIs3. The hpIs3 reporter expresses GFP tagged SYD-2 (alpha-liprin) in presynaptic active zones of GABA motor neurons (Zhen and Jin, 1999). In control animals, SYD-2::GFP is distributed in a punctate pattern at regularly interspaced intervals along the dorsal nerve cord (Figure 3) (Yeh et al., 2005). Loss of all PARG activity did not affect synapse morphology. Increased DLK signaling in dlk-1(OE) animals causes synapse morphology defects (Figure 3) (Nakata et al., 2005). In dlk-1(OE) animals, SYD-2::GFP is diffuse along the dorsal nerve cord, which increases the average baseline fluorescence along a line scan (Figure 3). However, loss of PARG activity did not suppress these defects. Together, these data indicate that in contrast to its role in axon regeneration, PARylation does not regulate synapse formation, even when DLK signaling is activated.
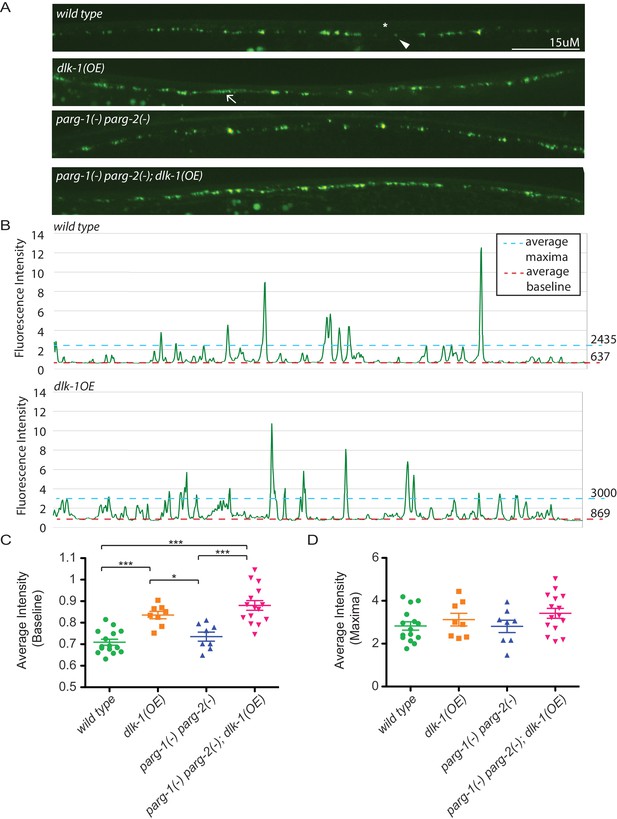
Loss of parg-1 and parg-2 function does not suppress mislocalizion of presynaptic active zones caused by dlk-1 overexpression.
(A) Dorsal nerve cords of wild type, dlk-1(OE), parg-1(lf) parg-2(lf), and dlk-1(OE); parg-1(lf) parg-2(lf) animals. All animals express the presynaptic active zone marker SYD-2::GFP in their GABA neurons. SYD-2::GFP is expressed in discrete puncta (arrowhead) in wild type animals and is not expressed continuously along the dorsal cord (asterisk). Conversely, SYD-2::GFP is expressed in a diffuse pattern (arrow) in dlk-1(OE) animals. (B) Average maxima and average baseline fluorescence are calculated along line scans of each dorsal cord and represented in (C). (C) dlk-1 overexpression disrupts SYD-2::GFP expression in GABA neurons and results in higher baseline fluorescence compared to wild type. Loss of parg function does not affect localization of SYD-2::GFP nor does it suppress the mislocalization caused by dlk-1(OE) (*p<0.05, ***p<0.01, multiple ANOVA, Bonferroni post-test). (D) There are no significant differences in average maxima between genotypes. Sample size is 15, 8, 8, and 15 animals for wild type, dlk-1(OE), parg-1(lf) parg-2(lf), and dlk-1(OE); parg-1(lf) parg-2(lf) animals, respectively.
Axon injury triggers an acute response that includes activation of DLK signaling (Yan and Jin, 2012). PARylation is a short-lived modification, and PAR levels are normally maintained by the continuous activity of PARP and PARG proteins (Schreiber et al., 2006; Gibson and Kraus, 2012). These data suggest a model in which increased PARG expression downstream of DLK signaling acutely reduces PAR levels in response to axon injury, thereby facilitating regeneration. We hypothesized that acute reduction of PAR levels by inhibition of PARP might also increase axon regeneration after injury, potentially similar to increased regeneration in PARP mutants (Figure 2). Multiple chemical PARP inhibitors are currently in preclinical and clinical trials for indications including cancer therapy and stroke (Ford and Lee, 2011; Anwar et al., 2015). We found that treatment with chemical PARP inhibitors after injury resulted in significantly enhanced axon regeneration in vivo in C. elegans GABA neurons and in vitro in murine cortical neurons (Figure 4A–C, and Figure 4—figure supplement 1). Drug treatment post-injury also improved behavioral recovery, demonstrating that enhanced regeneration after PARP inhibition results in functional reconnection (Figure 4D,E). Thus, acute poly(ADP-ribose) levels determine the response of neurons to axon injury, and inhibition of PARP after injury is sufficient to improve regeneration.
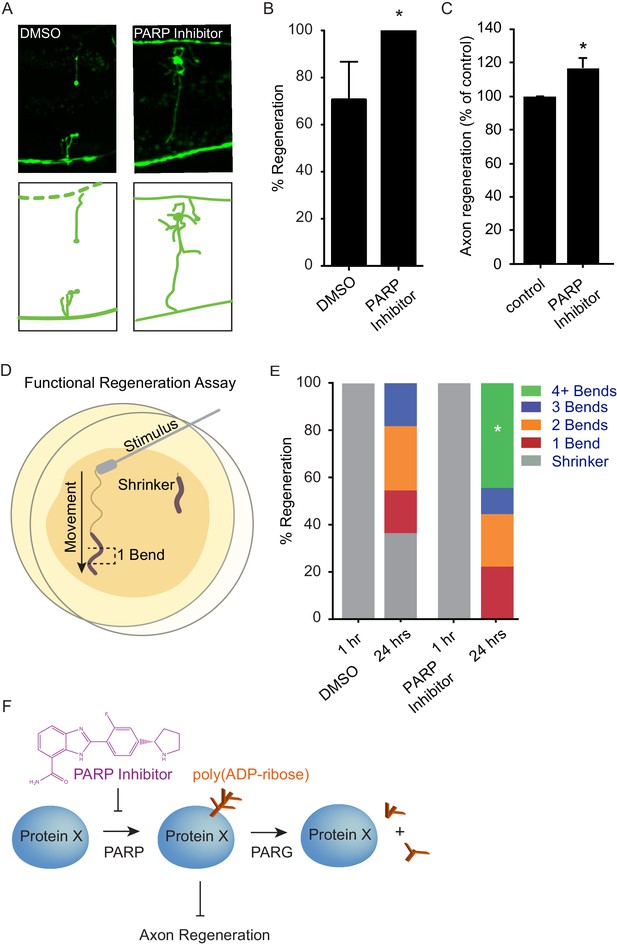
Chemical PARP inhibition enhances axon regeneration post-injury.
(A) Micrographs of regenerating axons placed on plates containing DMS0 or PARP inhibitor (A966492, Selleckchem, 100 µM) immediately after surgery. (B) Acute chemical inhibition of PARP function enhances regeneration (*p<0.05, Fisher’s exact test, n = 34 and 19 axons severed in animals exposed to DMSO or PARP inhibitor, respectively). (C) Axon regeneration is increased in murine cortical neurons exposed to chemical PARP inhibitor A966492 (*p=0.0149, Student’s t-test, n = 10). (D) To assess functional regeneration, all GABA neurons were severed and animals were assessed for their ability to reverse in response to a touch on the nose from a platinum wire. (E) One hour after all GABA neurons are severed, animals are incapable of reversing in response to a touch on the nose (shrinker). As functional connections are regenerated, animals recovered on PARP inhibitor displayed more backward movement than those recovered on DMSO (measured as number of body bends). Significantly more animals on PARP inhibitors recovered wild type function (4+ body bends, *p<0.05, Fisher’s exact test, n = 11 and 9 animals exposed to DMSO or PARP inhibitor, respectively). (F) The balance between PARP and PARG regulates axon regeneration and is altered by chemical PARP inhibitors.
Together, our findings suggest that regulation of PARylation is an important component of the DLK pathway role in the axon regeneration mechanism. Multiple lines of evidence suggest the parg genes are transcriptionally regulated downstream of DLK signaling to promote regeneration. First, we find both of the parg genes are upregulated in animals with activated DLK signaling. Second, we find that endogenous dlk-1 signaling drives parg-2 expression in an injury-dependent manner. Third, we find that loss of the parg genes reduces regeneration, both in animals with endogenous levels of dlk-1 activity and in animals with elevated DLK-1 signaling. These findings suggest a linear model in which DLK signaling induces parg expression, which in turn facilitates regeneration by removing PAR.
In addition to regulating PARylation, our data indicate that DLK signaling regulates regeneration upstream of multiple effectors. Although PARylation has a strong effect on regeneration in both animals with wild-type levels of dlk-1 and in animals that overexpress dlk-1, some GABA axons in parg-1(lf) parg-2(lf) mutants are still able to regenerate (Figure 1F), indicating high levels of PAR do not completely prevent DLK-mediated axon regeneration. By contrast, GABA axons do not regenerate in animals lacking DLK (Hammarlund et al., 2009; Yan et al., 2009). These data suggest that DLK activity has other functions besides regulating PARylation. Some of these functions may be mediated by other transcriptional outputs of DLK signaling (Watkins et al., 2013). Understanding these factors, as well as understanding the cellular effects of PAR on regeneration, await further study.
Besides shedding light on functional outputs of DLK signaling, our findings identify a novel pathway, involving control of poly(ADP-ribose) levels, that regulates axon regeneration (Figure 4F). Specifically, we find that PARG and PARP activity regulate the acute response of neurons to axon injury, and that chemical PARP inhibition after injury is sufficient to improve regeneration. The lack of additive phenotype in the double loss of function parg-1(-); parg-2(-) mutant suggests the two parg genes are not partially redundant. Rather, parg-1 and parg-2 may function together, for example as part of a complex. In Arabidopsis thaliana, the two PARG homologs physically interact (Song et al., 2015), suggesting the PARG homologs may function coordinately. Alternatively, concerted action of both PARGs may be required to maintain PAR levels below a threshold. In this model, loss of either single PARG results in a sufficient PAR increase to block regeneration, but increasing PAR beyond this threshold does not further reduce regeneration.
Previous investigations of the relationship between the two C. elegans parg homologs have been complicated by the physical proximity of the two genes in the genome. As a result, double loss of function mutants have been generated using RNAi. Since RNAi can result in incomplete knockdown of target genes, it has been difficult to determine the functional redundancy of the two genes using this approach. The parg-1; parg-2 mutant described here may be useful for further characterization of animals that completely lack PARG function.
In vivo, injured mammalian axons must overcome extrinsic growth inhibition to regenerate. PARP1 is upregulated in murine cortical neurons exposed to inhibitory growth molecules (myelin-associated glycoprotein, Nogo-A, Chondroitin sulfate proteoglycans) in vitro and in crushed optic nerves in vivo. Moreover, inhibiting PARP1 promotes neurite outgrowth on inhibitory substrates in vitro (Brochier et al., 2015). Since PARPs and PARGs have contrasting effects on PAR levels, NAD+ levels, which are substrates of PAR (Bai, 2015), and on axon regeneration, we conclude the balance between PARP and PARG function regulates axon regeneration, and present the hypothesis the PARG-PARP balance may determine axon regeneration by regulating PAR levels or by regulating NAD+ levels. Finally, the conservation of the role of PARP in mammalian axon regeneration may have important implications for nerve repair following injury or disease.
Materials and methods
C. elegans strains
Request a detailed protocolStrains were maintained as previously described at 20°C (Brenner, 1974). Some strains were provided by the CGC, which is funded by NIH Office of Research Infrastructure Programs (P40 OD010440). Specific mutations analyzed: parg-1(gk120), parg-2(ok980), parp-1(ok988), parp-2(ok344), wpIs9[Punc-47:DLK-1mini-GFP, ccGFP], pmk-3(ok169), hpIs3[punc-25::SYD-2::GFP; lin-15+]. To visualize GABA neurons in regeneration assays, mutants were crossed into the oxIs12 [Punc-47:GFP, lin-15+] background. XE1347 wpIs39[Punc-47:mCherry], XE1551 wpIs9[Punc-47:DLK-1mini-GFP, ccGFP]; wpIs39[Punc-47:mCherry], and XE1552 wpIs9[Punc-47:DLK-1mini-GFP, ccGFP]; pmk-3(ok169); wpIs39[Punc-47:mCherry] were analysed by RNA Seq.
RNA-Seq
Request a detailed protocolThe SeqCel method was used to generate RNA Seq profiles of larval GABA neurons (Spencer et al., 2014). Briefly, L4 stage larvae were dissociated and punc-47::mCherry-labeled GABA neurons were isolated by FACS (BD FACSaria) from wild-type (XE1347), dlk-1(OE) (XE1551) and dlk-1(OE); pmk-3(ok169) (XE1552) strains; dead and damaged cells were excluded by DAPI staining. Experiments were performed in triplicate for each genotype. For RNA-Seq analysis, total RNA (5–10 ng) was amplified by SMARTer cDNA synthesis (Clonetech) and libraries sequenced (PE-100) using the HiSeq 2500 system (Illumina). RNA-Seq data were analyzed with CLC Genomics Workbench software (Qiagen). A global comparison (EDGE test) (Robinson et al., 2010) of wild-type vs dlk-1(OE) GABA neuron RNA-Seq data sets detected 386 transcripts that are significantly up-regulated (>2 fold, p<0.05) in the dlk-1(OE) GABA neuron profile. A comprehensive analysis of these data sets will be presented elsewhere. The parg-2 transcript was significantly enriched (187x, p=1.6 e−14) in the dlk-1(OE) profile. The parg-1 transcript was 2.5 fold elevated in dlk-1(OE) but was excluded from this initial analysis due to a conservative p-value correction for multiple testing. We identified parg-1 as a likely false negative in this global analysis due to statistically significant elevation of the parg-1 signal in a direct comparison with wild-type and pmk-3(ok169) (XE1552) (see Figure 1A).
Expression analysis
Request a detailed protocolPparg-2::NLS::mCherry::NLS was constructed by combining Gateway plasmids encoding the parg-2 promoter sequence (obtained from GE Dharmacon Promoterome collection), NLS::mCherry::NLS coding sequence, the unc-54 UTR sequence, and pCFJ150. Pparg-2::NLS::mCherry::NLS was injected along with the Pmyo-2::GFP co-injection marker (expressed in the pharynx) into wpIs9[Punc-47:DLK-1mini-GFP, ccGFP]; oxIs12[Punc-47::GFP] animals. wpIs9 was outcrossed from transgenic lines with wild type N2 males. mCherry expression was compared between animals carrying the same extra-chromosomal array in the presence or absence of wpIs9. The posterior 7 VD/DD GABA neurons in 10 worms were analyzed for each genotype. Expression was analysed with an Olympus DSU mounted on an Olympus BX61 microscope, Andor Neo sCMOS camera, and Lumen light source. Error bars represent 95% confidence intervals. Significance is indicated with an asterisk (p<0.0001, Fisher exact test).
Axotomy experiments
Request a detailed protocolAxotomy experiments were carried out as previously described (Byrne et al., 2011). Post-axotomy images were acquired with an Olympus DSU mounted on an Olympus BX61 microscope, Andor Neo sCMOS camera, and Lumen light source. Error bars represent 95% confidence intervals. Significance is indicated with an asterisk (p<0.01, Fisher exact test).
CRISPR
Request a detailed protocolThe double parg-1(wp20) parg-2(ok980) mutant was created by injecting sgRNA targeting parg-1 sequence: aaagactacgaagactatgt and Cas9 into parg-2(ok980) animals. The resulting deletion of the 20th and 21st base pairs of the second parg-1 exon is a frameshift mutation that creates a truncated protein.
Transgenics
Request a detailed protocolPunc-47::parg-2 expressing animals were obtained by injecting parg-2(ok980); oxIs12 worms with pAB1019 DNA at 50 ng/μl along with Punc-25::mCherry at 10 ng/ul and Pmyo-2:mCherry at 2 ng/μl as a co-injection marker. 1 kb ladder was added at 50 ng/μl as carrier. The pAB1019 plasmid was constructed by combining Gateway plasmids encoding the unc-47 promoter sequence, parg-2 coding sequence (obtained from GE Dharmacon ORFeome collection), the unc-54 UTR sequence, and pCFJ150.
Cortical axon regeneration assay
Request a detailed protocolThe mouse cortical neuron axon regeneration assay was performed by scrape injury of confluent cultures, as described previously (Huebner et al., 2011). Primary cortical cultures were established from E17 C57BL/6 mice. Digested cells were plated on 96-well poly-D-lysine coated plates at a density of 25,000 cells per well in 200 µL of plating medium. Lentiviral particles encoding control non-targeting or PARP1 shRNA clones (Sigma) were added on DIV3 (Day In Vitro 3) as described for other shRNAs (Zou et al., 2015). On DIV8, 96-well cultures were scraped using a custom-fabricated 96-pin array and allowed to regenerate for another 72 hr before fixing with 4% paraformaldehyde. Regenerating axons in the scrape zone were visualized using an antibody against β3 tubulin (1:2000, mouse monoclonal; catalog #G712A; Promega). Growth cones were visualized by staining for F-actin using rhodamine-conjugated phalloidin (1:2000, catalog #R415, Life Technologies). Cell density was visualized using nuclear marker DAPI (0.1 μg/mL, catalog #4083, Cell Signaling Technology). Images were taken on a 10X objective in an automated high-throughput imager (ImageXpress Micro XLS, Molecular Devices) under identical conditions. Regeneration zone identification, image thresholding and quantitation were performed using an automated MATLAB script in a fully automated fashion.
Synapse analysis
Request a detailed protocolThe synapse marker hpIs3[punc-25::SYD-2::GFP; lin-15+] was crossed onto indicated combinations of parg(lf) and dlk-1(OE) backgrounds. The dorsal cords of the resulting animals were imaged with a 40X oil objective on an UltraVIEW Vox (PerkinElmer) spinning disc confocal microscope (Nikon Ti-E Eclipse inverted scope; Hamamatsu C9100-50 camera) with Volocity software (Improvision). Images were analyzed with ImageJ.
PARP inhibitors
Request a detailed protocolAll PARP inhibitors were acquired from Selleckchem. To assess the effects of PARP inhibitors on regeneration, GABA axons in L4 animals were axotomized and the worms placed on NGM plates (Brenner, 1974) containing 100 µM of the respective PARP inhibitor. Control plates were made with the same amount of DMSO as the plates containing inhibitor. Axon regeneration was scored 24 hr post-axotomy. Functional recovery was assessed by counting the number of body bends an animal made after being tapped on the nose with a platinum wire. Zero body bends is referred to as ‘shrinker’.
References
-
ADP-ribosylation of proteins. Enzymology and biological significanceMolecular Biology, Biochemistry, and Biophysics 37:1–237.https://doi.org/10.1002/abio.370090614
-
PARP inhibitorsHereditary Cancer in Clinical Practice 13:4.https://doi.org/10.1186/s13053-014-0024-8
-
In vivo laser axotomy in C. elegansJournal of Visualized Experiments 51:e2707.https://doi.org/10.3791/2707
-
Heritable genome editing in C. elegans via a CRISPR-Cas9 systemNature Methods 10:741–743.https://doi.org/10.1038/nmeth.2532
-
The genes pme-1 and pme-2 encode two poly(ADP-ribose) polymerases in Caenorhabditis elegansBiochemical Journal 368:263–271.https://doi.org/10.1042/bj20020669
-
New insights into the molecular and cellular functions of poly(ADP-ribose) and PARPsNature Reviews Molecular Cell Biology 13:411–424.https://doi.org/10.1038/nrm3376
-
A multi-domain fragment of Nogo-A protein is a potent inhibitor of cortical axon regeneration via Nogo receptor 1Journal of Biological Chemistry 286:18026–18036.https://doi.org/10.1074/jbc.M110.208108
-
Splitting of the ribose-ribose linkage of poly(adenosine diphosphate-robose) by a calf thymus extractThe Journal of Biological Chemistry 246:6362–6364.
-
Poly(ADP-ribose): novel functions for an old moleculeNature Reviews Molecular Cell Biology 7:517–528.https://doi.org/10.1038/nrm1963
-
Recovery from chronic spinal cord contusion after Nogo receptor interventionAnnals of Neurology 70:805–821.https://doi.org/10.1002/ana.22527
-
Protein turnover of the wallenda/DLK kinase regulates a retrograde response to axonal injuryThe Journal of Cell Biology 191:211–223.https://doi.org/10.1083/jcb.201006039
-
Glial inhibition of CNS axon regenerationNature Reviews Neuroscience 7:617–627.https://doi.org/10.1038/nrn1956
Article and author information
Author details
Funding
Craig H. Neilsen Foundation (279931)
- Alexandra B Byrne
National Institutes of Health (R21NS082667)
- David M Miller
- Marc Hammarlund
National Institutes of Health (R33NS079306)
- Stephen M Strittmatter
Falk Foundation
- Stephen M Strittmatter
The funders had no role in study design, data collection and interpretation, or the decision to submit the work for publication.
Acknowledgements
This work was supported by the Craig H Neilsen Foundation to ABB, by NIH R21NS082667 to MH and DMM, by NIH R33NS079306 to SMS and by the Falk Medical Research Trust to SMS.
Copyright
© 2016, Byrne et al.
This article is distributed under the terms of the Creative Commons Attribution License, which permits unrestricted use and redistribution provided that the original author and source are credited.
Metrics
-
- 2,118
- views
-
- 442
- downloads
-
- 42
- citations
Views, downloads and citations are aggregated across all versions of this paper published by eLife.
Citations by DOI
-
- 42
- citations for umbrella DOI https://doi.org/10.7554/eLife.12734