The antimicrobial peptide defensin cooperates with tumour necrosis factor to drive tumour cell death in Drosophila
Abstract
Antimicrobial peptides (AMPs) are small cationic molecules best known as mediators of the innate defence against microbial infection. While in vitro and ex vivo evidence suggest AMPs’ capacity to kill cancer cells, in vivo demonstration of an anti-tumour role of endogenous AMPs is lacking. Using a Drosophila model of tumourigenesis, we demonstrate a role for the AMP Defensin in the control of tumour progression. Our results reveal that Tumour Necrosis Factor mediates exposure of phosphatidylserine (PS), which makes tumour cells selectively sensitive to the action of Defensin remotely secreted from tracheal and fat tissues. Defensin binds tumour cells in PS-enriched areas, provoking cell death and tumour regression. Altogether, our results provide the first in vivo demonstration for a role of an endogenous AMP as an anti-cancer agent, as well as a mechanism that explains tumour cell sensitivity to the action of AMPs.
https://doi.org/10.7554/eLife.45061.001eLife digest
Animals have a natural defence system – the immune system – that is needed to fight off disease-causing microbes, known as pathogens. One way the immune system attacks pathogens is by producing small microbe-killing molecules called antimicrobial peptides. These antimicrobial peptides carry a positive charge, which allows them to interact with and disrupt the negatively charged cell surfaces of many microbes. Healthy animal cells do not have these negatively charged components on their cell surface, which means they are invisible to antimicrobial peptides. Studies have reported that antimicrobial peptides can attack cancer cells grown in a dish. However, it was unclear whether they could fight cancer cells in a live animal.
Parvy et al. have now addressed this issue by studying tumours in the larvae of fruit flies. Flies with tumours made an antimicrobial peptide called Defensin, which normally helps to fight infections. When Parvy et al. deleted the gene coding for Defensin, less tumour cells were dying and the tumours became bigger. This result indicated that Defensin was protecting the fruit flies from tumours. Examining the tumours under the microscope showed that Defensin protein interacted with the membranes of tumour cells. Defensin was not, however, interacting with healthy cells.
Further analysis revealed that a negatively charged component of cell membranes called phosphatidylserine, which normally faces the inside of healthy cells, is exposed to the outer surface of tumour cells. This negatively charged molecule renders cancer cells visible to positively charged Defensin. Importantly, the exposure of the phosphatidylserine is mediated by the fly equivalent of a protein called Tumour Necrosis Factor, a key player in cancer biology. Defensin binding to tumour cells leads to their death.
These experiments in the fruit fly highlight key molecular mechanisms that allow antimicrobial peptides to fight cancer cells in a living organism. Because human tumour cells can also expose phosphatidylserine, these latest findings may open up the possibility of a new kind of anti-cancer therapy for patients.
https://doi.org/10.7554/eLife.45061.002Introduction
Vast amount of evidence demonstrates the key role of systemic immunity in tumour progression and patient outcome. Efforts to manipulate the immune response to tumours are at the core of basic and translational cancer research. In mammals and flies, tumourigenesis triggers inflammation and activation of the immune system, leading to tumour cell death (Cordero et al., 2010; Parameswaran and Patial, 2010; Parisi et al., 2014; Teng et al., 2008). Tumour Necrosis Factor (TNF) is an important player in these tumour/immune interactions and has pleiotropic effects on tumours, including induction of cell death (Ham et al., 2016; Parameswaran and Patial, 2010). This function is conserved in Drosophila, where the single TNF homolog Eiger (Egr), produced by tumour-associated macrophages (TAMs), has been shown to drive cell death of neoplastic tumours, generated in larval imaginal discs by the loss of apico-basal complex components such as Disc large 1, Scribble or Lethal giant larvae (Cordero et al., 2010; Parisi et al., 2014; Parvy et al., 2018). Moreover, we have previously reported that tumours from disc large 1 Drosophila mutant larvae (dlg40.2 hereafter referred to as dlg), activate Toll pathway in the Drosophila fat body in an Egr-dependent manner, and this immune activation is necessary for TNF-dependent tumour cell death (Parisi et al., 2014). However, the mechanisms by which activation of an immune response in the fat body executes tumour cell death remain unknown (Figure 1A).
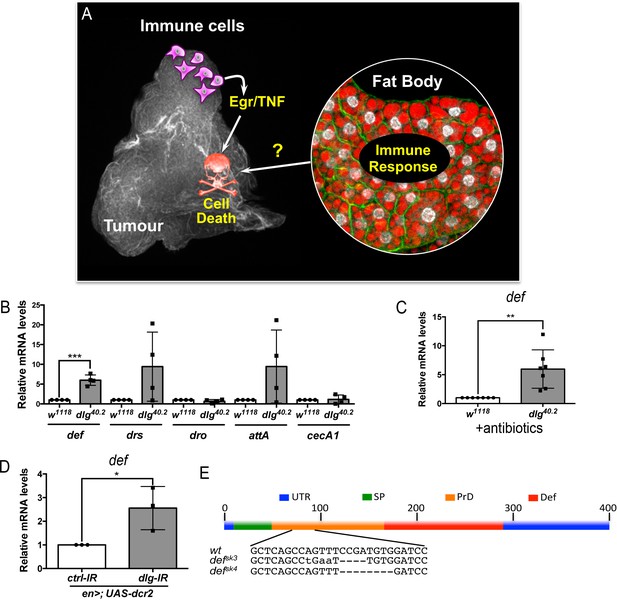
def is induced in dlg mutant tumour bearing animals.
(A) Working model showing the cooperation between haemocyte-derived TNF and the immune response in the fat body in tumour cell death (Parisi et al., 2014). (B) RT-qPCR analyses showing expression of several AMPs in the fat body of dlg40.2 mutant tumour bearing larvae compared to wild-type (w1118) larvae (n = 4). (C) RT-qPCR analysis of def expression in w1118 and dlg40.2 whole larvae reared on antibiotics (n = 7). (D) RT-qPCR analysis showing def expression in larvae expressing a ctrl-IR or a dlg-IR in the posterior part of the wing disc (en>;UAS-dcr2) (n = 3). (E) Schematic representation of the def gene locus showing mutant alleles generated (UTR: Untranslated Regions, SP: Signal Peptide, PrD: Pro-Domain, Def: Mature Defensin). Statistical analysis: B-D, Student t-test, B, ***p=0.0003, C, **p=0.0074, D, *p=0.042.
In Drosophila as in mammals, Toll pathway is well known to play a central role in the innate immune response to infection (Lemaitre et al., 1996). Downstream effectors of the Toll pathway include antimicrobial peptides (AMPs), which possess microbicidal activities against various pathogens. They display potent antimicrobial activity in vitro by disrupting negatively-charged microbial membranes. While intracellular activities have been reported, many AMPs kill pathogens by inserting into the lipid bilayer and disrupting the membrane integrity (Brogden, 2005). Host cells are instead protected from AMP as they are positively charged and contain cholesterol (Brender et al., 2012). In vitro studies have revealed AMPs capacity to kill cancer cells (Deslouches and Di, 2017). However, whether this cancer-killing activity is a natural function of AMPs is unknown, as there are no reports on an in vivo paradigm addressing such question. Since the Toll pathway is activated in dlg mutant tumour bearing larvae and is required for optimal TNF-induced tumour cell death (Parisi et al., 2014), we hypothesised that AMPs may be involved in this process.
Here we show that Drosophila defensin is induced in the fat body and tracheal system of dlg mutant tumour bearing larvae. We find Defensin consistently associated to dying tumour cells. Critically, systemic and tissue specific knockdown of Defensin demonstrates a non-redundant role of the AMP in controlling tumour growth through the induction of tumour cell death. Anti-tumoural Defensin production relies on TNF-dependent activation of both Toll and Imd pathway. Our results demonstrate that dlg mutant tumours expose PS in response to haemocyte-derived TNF and that Defensin is present in PS enriched area on the tumour surface. Finally, we find that lack of TNF prevents PS exposure in tumours and makes them insensitive to the action of Defensin. Collectively, our results reveal an anti-tumoural role for Defensin in vivo and provide insights into the molecular mechanisms, which make tumours sensitive to the killing action of an endogenous AMP.
Results
dlg tumour bearing larvae express the AMP defensin
In order to assess expression of several AMPs we performed RT-qPCR analysis on fat bodies dissected from wild type controls (w1118) or dlg mutant larvae (Figure 1B). Results showed consistent and statistically significant upregulation of defensin in fat bodies of dlg larvae compared to wild-type ones (Figure 1B). Other Toll-dependent AMPs display a trend to be increased (drosomycin and attacin A), even though data were highly variable amongst biological replicates, while other AMPs were not transcriptionally regulated (drosocyn, cecropin A1) (Figure 1B). Interestingly, human β-Defensin-1 displays anticancer activity in vitro (Bullard et al., 2008; Sun et al., 2006), and deletion of human def appears prevalent in some cancer types (Ye et al., 2018). This prompted us to explore the role of Drosophila Defensin in dlg mutant tumours. Using dlg larvae reared on antibiotics, we confirmed that defensin upregulation was independent of the presence of microbes (Figure 1C). Moreover, larvae bearing dlg imaginal discs tumours induced by RNAi (en >UAS-dcr2; UAS-dlg-IR) also displayed increased defensin expression, confirming def gene induction as a consequence of dlg-driven epithelial transformation rather than whole body dl loss (Figure 1D). We conclude that Defensin, an AMP known for its activity against microbes, is induced, in a sterile environment, by the presence of tumours.
Defensin restrains dlg tumour growth and promotes tumour cell death
We next hypothesised that Defensin may be an important mediator of anti-tumour immunity in vivo. To test this hypothesis, we generated null mutant alleles for the defensin (def) gene using the CRISPR/Cas9 system (defsk3 and defsk4) (Figure 1E) (Hanson et al., 2019). Survival analysis of defsk3 flies confirmed that this AMP contribute to resist systemic infection to certain Gram-positive bacteria (Figure 1—figure supplement 1) (Levashina et al., 1995). To evaluate the effect of Defensin on tumour development, we combined def and dlg loss of function alleles. Compared to dlg mutant animals, dlg;def double mutants displayed a significant increase in tumour size (Figure 2A). Tumour growth is limited by apoptotic tumour cell death as revealed by Dcp1 staining (Parisi et al., 2014). Interestingly, tumours from dlg;def double mutants display a very strong decrease in apoptosis (Figure 2B–E’), suggesting that increased tumour size in absence of Defensin is due to a decrease in tumour cell death. This was further supported by the similar proliferation rates measured in dlg and dlg,def mutant tumours as per quantification of anti-phophoHistone H3 staining (Figure 2—figure supplement 1A–E’). Importantly, the effect of Def on tumour size and cell death were still observed when dlg and dlg,def larvae were reared in sterile conditions (Figure 2—figure supplement 1F–J’) and further confirmed upon ubiquitous knock down of def using RNA interference (IR) (Figure 2F–J’). Furthermore, fat body overexpression of def significantly rescued tumour volume and tumour cell death of dlg;defsk3 double mutant animals (Figure 2K and L). Additionally, larval injection of a synthetic Defensin peptide increased tumour cell death of dlg or dlg;defsk3 imaginal discs (Figure 2M), while it had no effect on tissues from wild-type larvae, indicating that Defensin can selectively promote cell death of tumour cells. Altogether, these results demonstrate that Defensin is required to control dlg-dependent tumourigenesis in vivo through induction of tumour cell death.
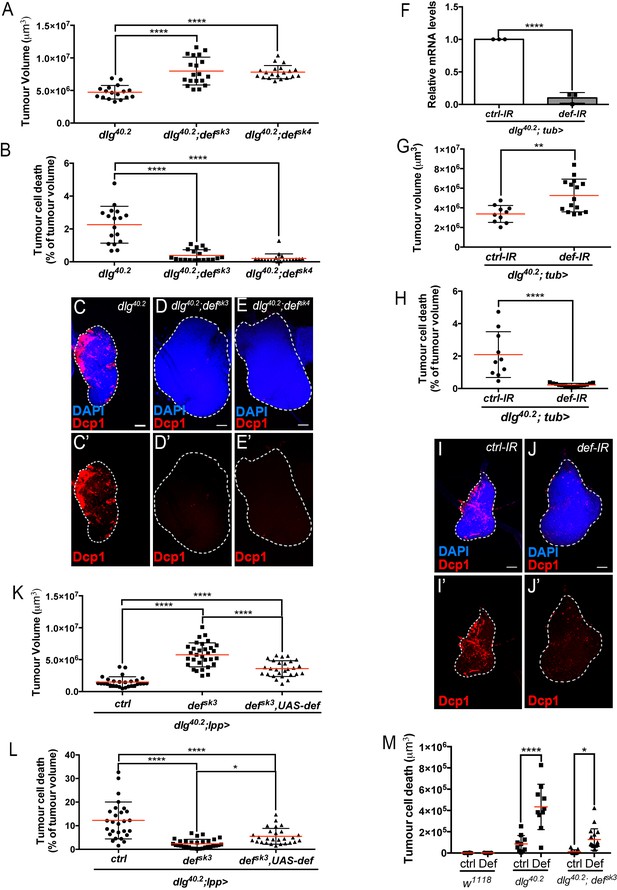
Def restricts tumour growth and promotes tumour cell death.
(A-E’) Quantification of tumour volume (TV) (A) and tumour cell death (TCD) (B) in wing imaginal discs from dlg40.2 (n = 17), dlg40.2;defsk3 (n = 19) and dlg40.2;defsk4 (n = 20) mutant larvae and representative immunofluorescence images of tissues quantified (C–E’). F, RT-qPCR analysis showing def expression upon ubiquitous knockdown (dlg40.2; tub>) (n = 3). G-J’, Quantification of TV (G) and TCD (H) in wing imaginal discs from larvae ubiquitously expressing a control-IR (ctrl-IR: n = 10) or a def-IR (n = 15) and representative immunofluorescence images of tissues quantified (I–J’). K-L, Quantification of TV (K) and TCD (L) in wing imaginal discs from dlg mutant controls (dlg40.2;lpp>: n = 28), dlg;defsk3 mutants controls (dlg40.2;defsk3;lpp>: n = 31) or dlg;defsk3 mutants expressing def in the fat body (dlg40.2;defsk3,UAS-def;lpp>: n = 27). M, Effect of PBS (ctrl) or synthetic Def injection on TCD from wild-type larvae (w1118, ctrl: n = 10, Def: n = 9), dlg40.2 (ctrl: n = 10, Def: n = 10) and dlg40.2;defsk3 (ctrl: n = 11, Def: n = 18) mutant larvae. Tissues were stained with 4’,6-diamidino-2-phenylindole (DAPI, blue) for nuclei visualisation and with anti-cleaved Decapping protein 1 (Dcp1) antibody (red) to detect apoptotic cell death. Scale bars = 50 μm. Statistical analysis: A, B, K, L, One way ANOVA, *p<0.05, ****p<0.0001; F, Student t-test, ****p<0.0001, G-H, Mann-Whitney test, G, **p=0.0054, H, ****p<0.0001; M, Two way ANOVA (only relevant significant statistics are indicated), *p<0.05, ****p<0.0001.
Defensin remotely produced from immune tissues bind to tumour cells
Having shown that Defensin restrict tumour growth, we sought to determine the tissues that produced endogenous Defensin in the context of tumour bearing. Previous studies have shown that Defensin is not produced by imaginal discs or tumours (Bunker et al., 2015; Külshammer et al., 2015). In the context of infection, Defensin can be produced by the fat body as well the tracheal and gut epithelium (Tzou et al., 2000). We monitored defensin expression by RT-qPCR in various immune tissues of tumour bearing animals. We observed that the fat body, homologue to the mammalian liver and adipose tissue, and the trachea, a network of tubes transporting oxygen to cells that resembles the mammalian vasculature, were the main sources of defensin in these animals (Figure 3A). This was also supported by Defensin immunostaining, (Figure 3B and C). Transcript assessment upon targeted IR knock down of defensin in the respective tissues further confirmed the domains of endogenous gene expression (Figure 3D and I). Importantly, knocking down defensin expression specifically in the fat body or the trachea of dlg animals, resulted in increased tumour size and decreased tumour cell death (Figure 3E–H’ and J–M’) confirming the non-redundant functional requirement of defensin in both tissues to efficiently promote tumour cell death.
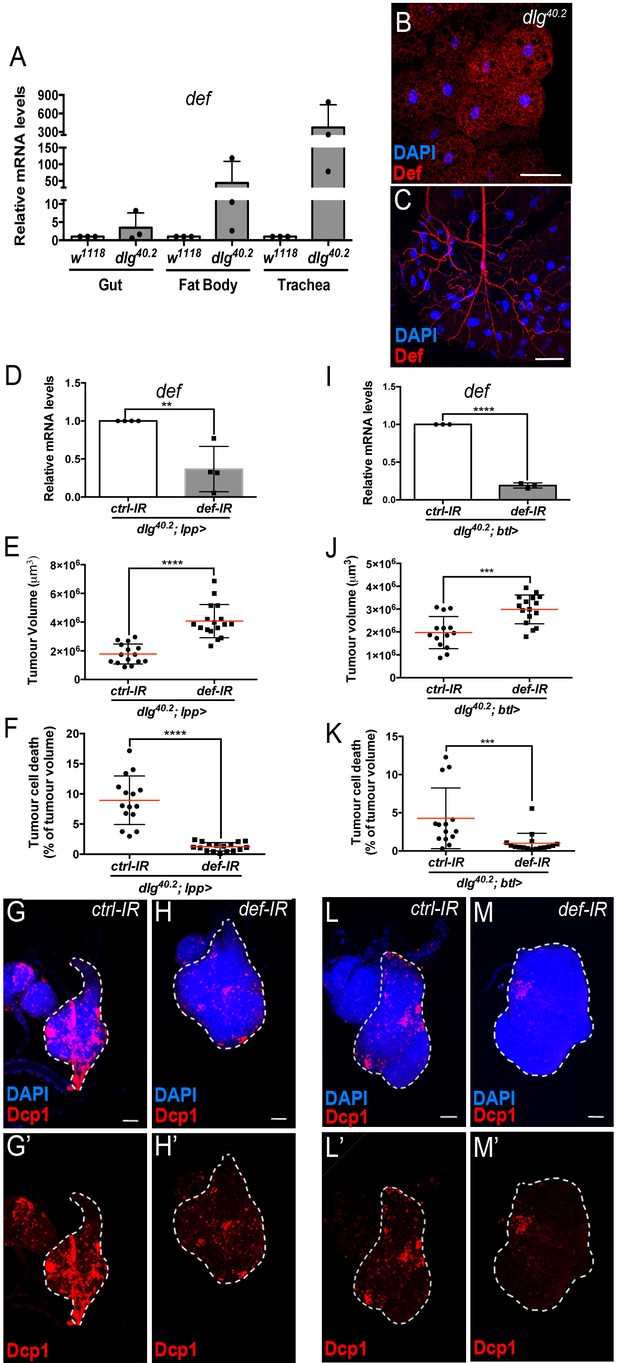
Def from the trachea and the fat body mediates tumour cell death.
(A) RT-qPCR analysis of def expression in gut, fat body and trachea dissected from w1118 or dlg40.2 mutant larvae (n = 3). B-C, Fat body (B) and trachea (C) from dlg40.2 mutant larvae stained with DAPI (blue) and anti-Def antibody (red). D, RT-qPCR analysis of def expression in dlg40.2 mutant larvae (dlg40.2;lpp>) expressing a ctrl-IR or def-IR in the fat body (n = 4). E-H’, Quantification of TV (E) and TCD (F) in wing imaginal discs from dlg40.2 mutant larvae (dlg40.2;lpp>) expressing a ctrl-IR (n = 15) or def-IR (n = 17) in the fat body and representative immunofluorescence images of tissues quantified (G–H’). I, RT-qPCR analysis of def expression in dissected trachea from dlg40.2 mutant larvae (dlg40.2;btl>) expressing a ctrl-IR or def-IR in the trachea (n = 3). J-M’, Quantification of TV (J) and TCD (K) in wing imaginal discs from dlg40.2 mutant larvae (dlg40.2;btl>) expressing a ctrl-IR (n = 14) or def-IR (n = 16) in the trachea and representative immunofluorescence images of tissues quantified (L–M’). Tumours were stained with DAPI (blue) and anti-Dcp1 antibody (red). Scale bars = 50 μm. Statistical analysis: A, D, I, Student t-test, D, **p=0.0054, I, ****p<0.0001; E, F, J, K, Mann-Whitney test, E, F, ****p<0.0001, J, ***p=0.0009, K, ***p=0.0004.
To investigate the possibility that Defensin produced by the fat body and the trachea can specifically target tumour cells, we made use of an inducible haemagglutinin (HA) tagged form of Defensin (UAS-def-HA). We noticed leaky HA protein expression in the tracheal system (Figure 4—figure supplement 1A) but not in the fat body (Figure 4—figure supplement 1B) of animals carrying the UAS-def-HA construct only. Therefore, we overexpressed UAS-def-HA in the fat body using a Gal4 specific driver (lpp >def HA), which resulted in significant upregulation of Defensin in the fat body (Figure 4—figure supplement 1D) when compared to control conditions (Figure 4—figure supplement 1B), but also maintained the leaky expression of the transgene in the trachea (Figure 4—figure supplement 1C). Strikingly, overexpression of UAS-def-HA in the fat body resulted in Defensin-HA immunostaining in transformed imaginal discs from dlg mutant animals (Figure 4A–A’’ and B–B’’) but not in normal tissues from dlg heterozygous animals (Figure 4C and C’).
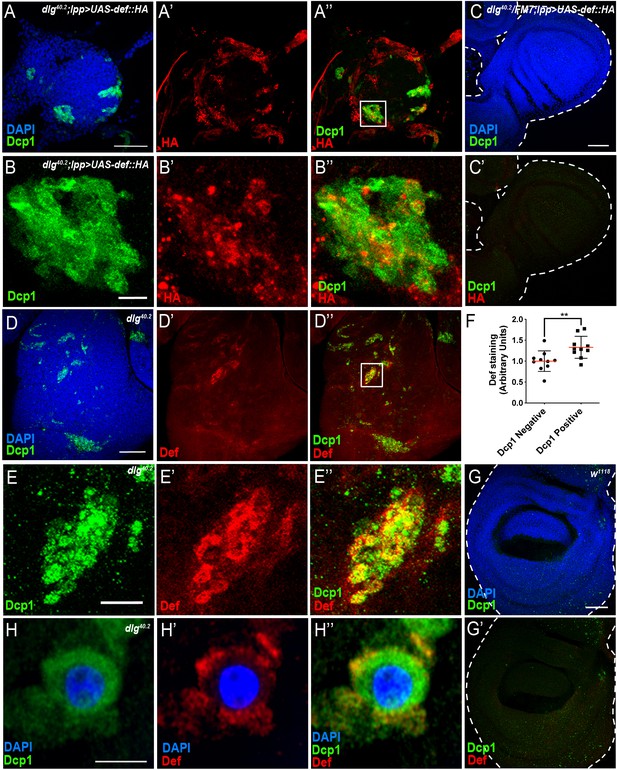
Def produced by immune tissues specifically targets tumour cells.
(A-A’’) DAPI (blue), anti-Dcp1 (green) and anti-HA antibody (red) staining of dlg40.2 mutant tumour from larvae overexpressing a def-HA construct in the fat body and the trachea (dlg40.2;lpp >UAS-def-HA). B-B’’, Enlargement of inset from A’’ (white outline) showing Dcp1 (B), Def-HA (B’) and merged channels (B’’). C-C’, DAPI (blue), anti-Dcp1 (green) and anti-HA antibody (red) staining of dlg40.2 heterozygous wing disc from larvae overexpressing def-HA (dlg40.2/FM7;lpp >UAS-def-HA). D-D’’, dlg40.2 mutant tumour stained with DAPI (blue), anti-Def (red) and anti-Dcp1 (green) antibodies. E-E’’, Enlargement of inset from D’’ (white outline) showing Dcp1 (E), Def (E’) and merged channels (E’’). F, Quantification of colocalization between Def and Dcp1 staining (n = 10). G-G’, wild type (w1118) wing imaginal disc stained with DAPI (blue), anti-Def (red) and anti-Dcp1 (green) antibodies. H-H’’, High-resolution imaging of a single dying tumour cell stained with DAPI (blue), anti-Def (red) and anti-Dcp1 (green) antibodies. A, C, D, G, Scale bars = 50 μm; B, E, Scale bars = 10 μm; H, Scale bar = 2.5 μm. Statistical analysis: F, Student t-test, **p=0.0093.
Consistently, using anti-Defensin antibody, we observed endogenous Defensin staining on dlg mutant tumour (Figure 4D–D’’ and E–E’’) but not in wild-type discs (Figure 4G,G’). Interestingly, we observed Defensin preferentially bound to tumour areas enriched with apoptotic cells (Figure 4D–D’’ and E–E’’). This was confirmed by quantification of the amount of Def staining colocalising with Dcp1 staining (Figure 4F). High-resolution imaging showed Defensin enrichment at the membrane of these dying cells (Figure 4H–H’’).
Altogether, these results show that Defensin produced by immune tissues selectively binds tumour cells to target them for apoptosis.
Toll and Imd pathway contribute to Defensin expression in dlg mutant larvae
defensin expression upon systemic infection relies on both Toll and Imd pathways (Lemaitre et al., 1996; Leulier et al., 2000). While we previously showed that Toll pathway activation in dlg mutant larvae is required to achieve maximal tumour cell death (Parisi et al., 2014), the involvement of the Imd pathway in tumour bearing animals was still elusive. To assess the contribution of the Imd pathway to both defensin expression and tumour burden, we analysed these two phenotypes in larvae deficient for the Imd-pathway. We observed a 55–60% decrease in defensin expression in dlg mutants carrying a loss of function allele affecting imd (dlg;imd1) (Figure 5A) or the gene encoding the downstream transcription factor Relish (dlg;relE20) (Figure 5F). Consistently, analysis of tumour phenotypes revealed increased tumour volume and decreased tumour cell death in dlg;imd1 and dlg;relE20 animals when compared with dlg counterparts (Figure 5B–E’ and G–J’). Altogether, these data demonstrate that the Imd pathway is required for defensin upregulation, impairment of tumour growth and induction of tumour cell death in dlg mutant larvae.
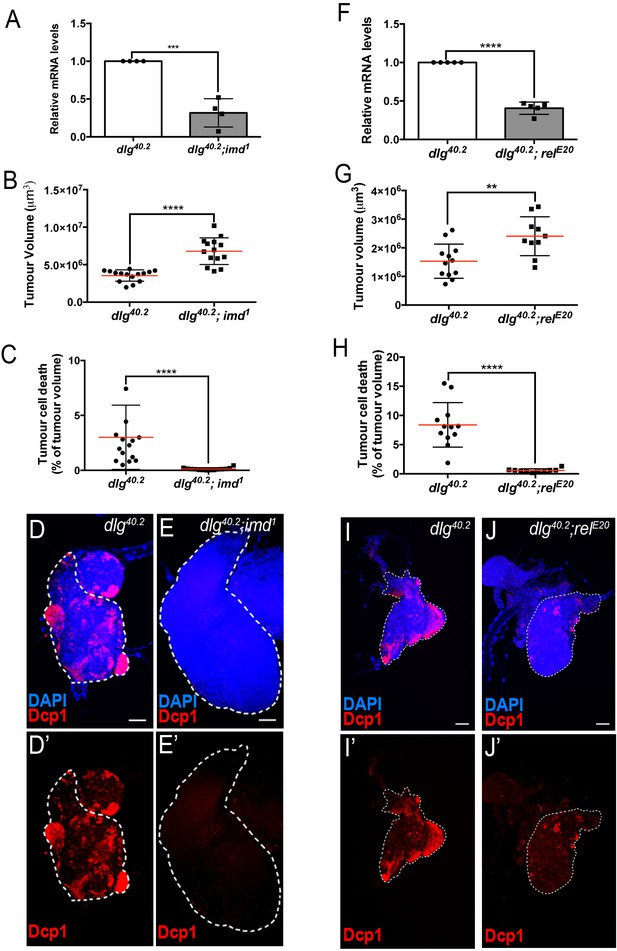
Imd pathway activation is required for def expression and Def-mediated tumour cell death.
(A) RT-qPCR analysis of def expression from dlg40.2 and dlg40.2;imd1 mutant larvae (n = 4). B-E’, Quantification of TV (B) and TCD (C) in wing imaginal discs from dlg40.2 (n = 15) and dlg40.2;imd1 (n = 14) mutants larvae and representative immunofluorescence images of tissues quantified stained with DAPI (blue) and anti-Dcp1 antibody (red) (D–E’). F, RT-qPCR analysis showing def expression in dlg40.2 and dlg40.2; relE20 mutant animals (n = 5). G-J’, Quantification of TV (G) and TCD (H) from dlg40.2 (n = 12) and dlg40.2;relE20 (n = 10) mutant larvae and representative pictures of the corresponding tumours (I–J’). Scale bars = 50 μm. Statistical analysis: A, F, Student t-test, A, ***p<0.0003, F, ****p<0.0001; B, C, G, H, Mann-Whitney test, B, C, H, ****p<0.0001, G, **p=0.009.
Upon infection, AMP expression in the trachea exclusively relies on the Imd pathway (Tzou et al., 2000). We therefore looked at defensin expression and tumour phenotype in dlg mutant larvae where imd expression had been knocked down specifically within tracheal cells (dlg;btl >imd-IR). In this setting, defensin expression was significantly reduced in the whole larvae (Figure 6A). Consistently, tumour volume was increased while tumour cell death was decreased (Figure 6B–E’) showing the requirement of Imd pathway in the tracheal system to control tumour burden.
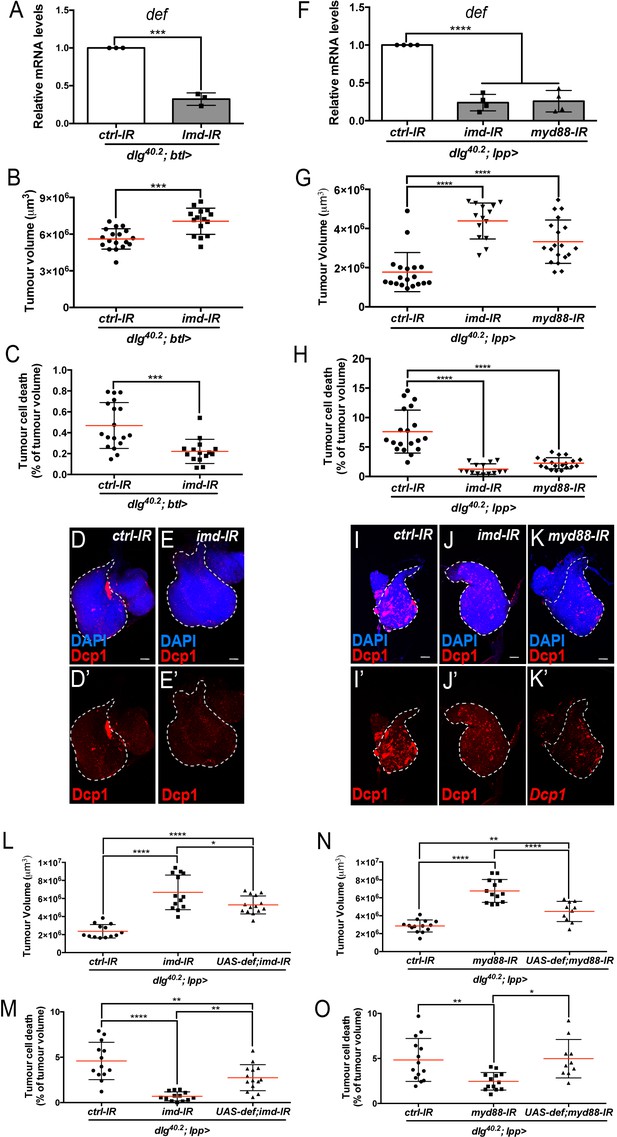
Imd and Toll pathway activation are required in the trachea and the fat body to promote Defensin-dependent tumour cell death.
(A) RT-qPCR analysis of def expression from dlg40.2 mutant larvae (dlg40.2;btl>) expressing a ctrl-IR or imd-IR in the trachea (n = 3). B-E’, Quantification of TV (B) and TCD (C) from dlg40.2 mutant larvae (dlg40.2;btl>) expressing a ctrl-IR (n = 18) or imd-IR (n = 15) in the trachea and representative immunofluorescence images of tissues quantified stained with DAPI (blue) and anti-Dcp1 antibody (red) (D–E’). F, RT-qPCR analysis of def expression from dlg40.2 mutant larvae (dlg40.2;lpp>) expressing a ctrl-IR, an imd-IR or a myd88-IR in the fat body. G-K’, Quantification of TV (G) and TCD (H) from dlg40.2 mutant larvae (dlg40.2;lpp>) expressing a ctrl-IR, an imd-IR or a myd88-IR in the fat body and representative immunofluorescence images of tissues quantified (I–K’). L-O, Quantification of TV (L, N) and TCD (M, O) from dlg40.2 mutant larvae (dlg40.2;lpp>) expressing a ctrl-IR, an imd-IR alone or in combination with a UAS-def in the fat body (L, M) and from dlg40.2 mutant larvae (dlg40.2;lpp>) expressing a ctrl-IR, a myd88-IR alone or in combination with a UAS-def in the fat body (N, O). Scale bars = 50 μm. Statistical analysis: A, Student t-test, A, ***p=0.0001; B, C, Mann-Whitney test, B, ***p=0.0003, C, ***p=0.0002; F, G, H, L-O, One way ANOVA, *p<0.05, **p<0.01, ****p<0.0001.
In the fat body, both Imd and Toll pathways contribute to AMPs expression during infection (Tzou et al., 2002). To evaluate the contribution of Toll and Imd pathways in fat body in tumour bearing larvae, we monitored defensin expression and tumour phenotype in dlg animals in which Toll or Imd pathways have been selectively knocked down in the fat body. Knocking down myd88, which encodes a common adaptor to Toll receptors (Imler and Zheng, 2004), or imd in the fat body resulted in a significant decrease in defensin expression (Figure 6F) and, consistently, increased tumour volume and decreased tumour cell death (Figure 6G–K’). Moreover, concomitant overexpression of defensin and Myd88-IR or Imd-IR in the fat body of dlg larvae was sufficient to significantly rescue the tumour volume and tumour cell death phenotypes resulting from fat body knockdown of Myd88 or Imd in dlg animals (Figure 6L–O).
We conclude that, in dlg mutant animals, Toll and Imd pathways have non-redundant roles in restricting tumour growth and promoting tumour cell death through the control of defensin expression. Importantly, forced defensin expression in an otherwise immune-compromised animal is sufficient to reduce tumour growth and to promote tumour cell death.
Defensin is enriched in tumour areas exposing phosphatidylserine
AMPs targeting of pathogens, involves the recognition of negatively charged molecules exposed on the cell surface (Yeaman and Yount, 2003). A key question raised by our study is how Defensin can selectively bind and kill tumour cells (Figure 4 and Figure 2M). Selective action of cationic AMP is attributed to their ability to interact with negatively charged membrane such as those found in bacteria. We hypothesised that the membrane of tumour cells from dlg mutant larvae might change their electrostatic properties, making them sensitive to the action of Defensin. Phosphatidylserine (PS) is a negatively charged phospholipid, normally restricted to the inner leaflet of the cell membrane. However, PS can be exposed on the outer leaflet for example in apoptotic cells, which tags these cells for phagocytosis (Birge et al., 2016; Shklyar et al., 2013; Tung et al., 2013). Moreover, PS exposure has been shown to occur independently of apoptosis in many cancer cell types (Riedl et al., 2011). Therefore, we investigated whether PS externalisation could be a factor allowing specific targeting of tumour cells by Defensin. Our data revealed that dlg but not wild-type tissues, displayed high levels of Annexin V staining (Figure 7A and F; compare to E), indicating increased exposure of PS by dlg tumours. We next looked at the ability of Defensin to specifically associate with tumour cells exposing PS and found that Defensin was enriched in Annexin V+ve areas on dlg tumours (Figure 7A–A’’ and B–B’’). This was confirmed by quantification of the amount of Def staining colocalising with Annexin V staining (Figure 7C). Thus, Defensin produced by immune responsive tissues binds specifically to tumour cells and this ability correlated with their exposure of PS.
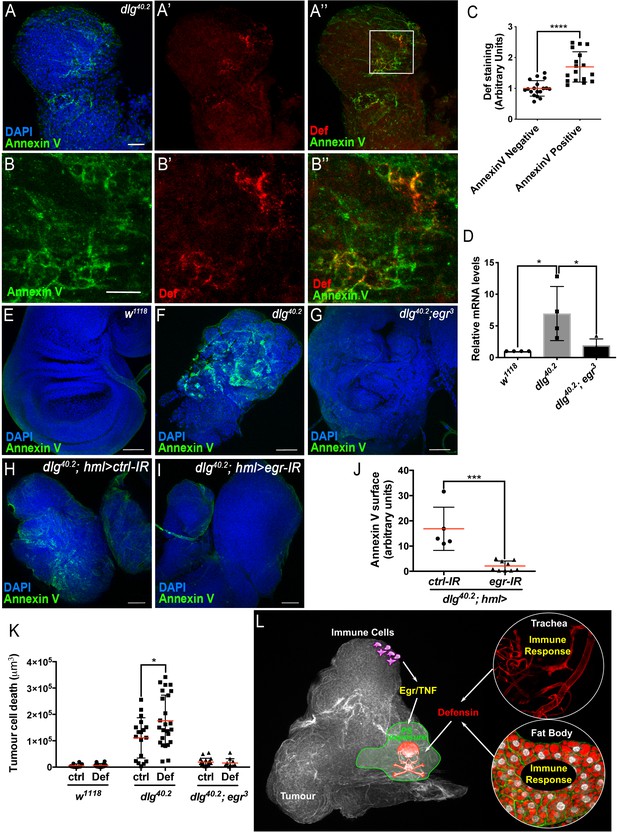
TNF is required for PS exposure and Defensin-driven tumour cell death.
(A-A’’) DAPI (blue), Annexin V (green) and anti-Def (red) staining of dlg40.2 mutant tumours. (B-B’’) Enlargement of inset from A’’ (white outline) showing Annexin V (B), Def (B’) and merged channels (B’’). (C) Quantification of colocalisation between Def and Annexin V staining (n = 18). (D) RT-qPCR analysis showing def expression in wild-type, dlg40.2 or dlg40.2;egr3 mutants. (E- G) Annexin V (green) and DAPI (blue) staining of wing imaginal discs from larvae of the indicated genotypes. (H, I) Annexin V (green) and DAPI (blue) staining of wing imaginal discs from dlg mutant larvae (dlg40.2,hml>) expressing a ctrl-IR (n = 5) or an egr-IR (n = 9) in the haemocytes. (J) Quantification of Annexin V signal on tumours from the corresponding genotypes. (K) Quantification of TCD upon PBS (ctrl) or Def injection in wild-type (w1118, ctrl: n = 10, Def: n = 14), dlg40.2 (ctrl/Def: n = 18) or dlg40.2;egr3 (ctrl: n = 20, Def: n = 13) mutant larvae. (L) A model for Def antitumoural activity. A, E-I, Scale bars = 50 μm; B, Scale bars = 20 μm. Statistical analysis: C, Student t-test, ****p<0.0001; D, One way ANOVA, *p<0.05; J, Mann-Whitney test, ***p=0.001; K, Two way ANOVA (only relevant significant statistics are indicated), *p<0.05.
The TNF homolog Eiger is required for PS exposure and defensin anti-tumoural activity
Previous studies have shown that circulating macrophage-like cells in Drosophila, called haemocytes, bind to dlg mutant tumours and contribute to cell death (Parisi et al., 2014). This process is mediated by the release of Egr from haemocytes (Cordero et al., 2010; Parisi et al., 2014), which then activates the JNK pathway in target cells to promote apoptosis (Igaki et al., 2009). Moreover, Toll pathway activation in tumour bearing animals also requires Egr (Parisi et al., 2014). Accordingly, we found that defensin upregulation observed in dlg animals was lost in dlg;egr3 double mutants (Figure 7D). We next tested whether PS exposure in tumours was dependent on Eiger, and observed an almost complete loss of cell-surface PS in dlg;egr3 tumours (Figure 7E–G). Therefore, Egr is required for PS exposure in these tumours. We then analysed PS exposure in dlg mutant tumours, upon specific egr knockdown in haemocytes (dlg;hml >egr-IR), and observed a strong decrease in cell surface-exposed PS compared to control tumours (dlg;hml >ctrl-IR) (Figure 7H–J). This indicates that PS exposure in tumour cells is likely triggered by the release of Egr from haemocytes. Finally, to further test the hypothesis that Def requires PS to bind to tumours, we assessed the ability of overexpressed Def-HA to bind to dlg,egr3 mutant tumours. Consistently with the observed basal and overexpressed Def-HA expression pattern in wild type tissues (Figure 4—figure supplement 1), Def-HA was expressed in the trachea and fat body of dlg,egr3 mutant animals (Figure 7—figure supplement 1A,B). However, we did not find any detectable association of Def-HA to the surface of dlg,egr3 tumours (Figure 7—figure supplement 1C,C’).
Importantly, Egr-dependent signalling and PS exposure were intact in dlg;def and dlg;imd mutants (Figure 7—figure supplement 2) indicating that both events precede the action of Defensin in dlg tumours. To further assess whether Egr was required for Defensin-induced tumour cell death, we injected synthetic Defensin peptide into control, dlg or dlg;egr3 mutant larvae. While, Defensin injection was able to robustly promote tumour cell death in dlg mutant tumours, it was unable to affect tumours derived from Egr-deficient animals, further demonstrating the requirement of Egr for tumour cell death induced by Defensin (Figure 7K).
Finally, we tested whether the antitumoral action of Def extended to other tumour models. We observed that tumours induced by the loss of scribble (scrib), another member of the same apico-basal complex as dlg, showed significant cell surface PS exposure, which was Egr dependent (Figure 7—figure supplement 3A,A’; compare to Figure 7—figure supplement 3B,B’). Moreover, we detected Def enrichment in areas positive for Dcp1 staining on scrib tumours (Figure 7—figure supplement 3C–C’’ and D–D’’). Consistently, removing def from scrib mutant animals led to increase in tumour volume and decrease in tumour cell death (Figure 7—figure supplement 3E–H’). Altogether, these results show that the sensitivity to Def is not restricted to dlg mutant tumours but might rather be a general feature of neoplastic growth induced by loss of cell polarity.
Discussion
While the role of antimicrobial peptides in innate immune defense has been well-recognised for decades (Bahar and Ren, 2013), recent reports revealed potential additional physiological functions of AMPs, including ageing and neurodegeneration (Cao et al., 2014; Kounatidis et al., 2017; Lezi et al., 2018), wound-healing (Chung et al., 2017; Tokumaru et al., 2005), resistance to oxidative stress (Mergaert et al., 2017; Zhao et al., 2011), immune signaling (Tjabringa et al., 2003; van Wetering et al., 2002) and anti-cancer activity (Deslouches and Di, 2017). However, due to the absence of AMP mutants, most of these studies have relied on the use of exogenous sources of AMPs or genetic modification of upstream regulators of AMP expression. Recently, the use of loss of function alleles of Drosophila Diptericin (DptB), allowed to establish a role for the AMP in long-term memory (Barajas-Azpeleta et al., 2018). Pioneer work reporting systematic deletion of multiple AMPs in Drosophila, opens the door for in-depth analysis of the endogenous functions of these molecules (Hanson et al., 2019). Using such tools and a genetically defined in vivo tumour model, our study demonstrates a role for Defensin in the control of tumours and deciphers the molecular mechanism allowing specific tumour cell targeting by the AMP. Collectively, our results suggest that the two branches of Drosophila innate immunity contribute to tumour elimination (Figure 7L). The cellular immune response to tumours involves the binding of haemocytes to tumour cells and activation of TNF pathway leading to PS exposure (Figure 7L). This is followed by a humoral response in distant tissues (fat body and trachea) that triggers tumour cell death through the action of Defensin (Figure 7L).
A role for a Drosophila AMP in tumour control
Using mutants as well as ubiquitous or targeted knockdowns, our study reveals that Defensin is non-redundantly required to drive tumour cell death and restrict tumour growth in neoplastic tumours generated by loss the apico-basal determinant Dlg and Scrib. This was reinforced by detection of Defensin on dying tumour cells as well as genetic rescue and injection experiments showing that Defensin can actively drive tumour cell death. Several in vitro studies in mammals have pointed to AMPs anti-tumoural potential (Deslouches and Di, 2017). Amongst these suggested anticancer peptides, Human β-Defensin-1 (hBD1) appears downregulated in 82% of prostate cancer and 90% of renal clear cell carcinomas (Donald et al., 2003). Furthermore, expression of hBD1 induces cell death in prostate and renal cancer cells in vitro (Bullard et al., 2008; Sun et al., 2006). Moreover, a recent report shows prevalent deletion of Human defensin gene cluster in some tumour types including prostate, lung and colorectal cancers, as well as a decrease overall survival in patients carrying these deletions (Ye et al., 2018). However, since the classification of a molecule as AMP is based on structural protein features, it is important to mention that Human Defensins are not homologous of, but rather have structural resemblances, to Drosophila AMPs due to convergent evolution (Shafee et al., 2017). Further studies are required to determine if anticancer activity displayed by Defensins from different species is linked to their structural properties.
As upon infection (Tzou et al., 2000), we show that the fat body and the trachea are the main sources of Defensin in tumour bearing larvae. Moreover, our results indicated that Defensin’s maximal expression and anti-tumour properties rely on Imd and Toll pathways activation. As expected from previous studies on anti-pathogenic immunity, Imd appears to play a critical role in the tracheal system, while both Imd and Toll drive Defensin expression in the fat body (Hoffmann and Reichhart, 2002; Tzou et al., 2002). Consistently, overexpressing Defensin partially rescued the effect of Imd or Toll knockdown on dlg tumours. However, Toll and Imd pathways are major regulators of multiple AMPs in the Drosophila fat body. Then, it is conceivable that AMPs other than Defensin may possess similar anti-tumoural activity. In fact, a recent study in Drosophila shows that ectopic expression of several antimicrobial peptides, including Defensin, can increase cell death in a haematopoietic tumour model (Araki et al., 2019). Authors of that study also reported activation of Toll and Imd pathways together with increased expression of several AMPs in those tumour bearing animals. Together with our study, this suggests potentially general anti-tumoural properties of Drosophila AMPs.
Defensin specifically targets tumour cells
Importantly, we show that Defensin targets tumour cells for apoptosis while sparing normal cells. As their human counterparts, we show that Drosophila tumours can expose PS independently of cell death (Riedl et al., 2011). Interestingly, an in vitro study showed selective anticancer activity of some synthetic peptides derived from beetle Defensin, against cancer cells exposing PS (Iwasaki et al., 2009). A similar targeting mechanism has also been proposed to explain temporin-1CEa or L-K6 anticancer activities towards melanoma cells and breast cancer cells respectively (Wang et al., 2016; Wang et al., 2017). PS is a mark of apoptotic cells, which is used as an ‘eat me’ signal by phagocytes (Shklyar et al., 2013; Tung et al., 2013). In Drosophila, the phagocytic receptor Simu together with Draper contribute to the elimination of apoptotic cells through recognition of PS (Shklyar et al., 2013; Tung et al., 2013). It would be interesting to test the role of these receptors in the control of tumour progression in Drosophila.
Our results indicate that PS exposure precedes Defensin action and is not just an ‘eat-me’ signal but likely contributes to changing the membrane of tumour cells making them sensitive to the action of AMPs. It is tempting to speculate that the deleterious effect of AMPs observed upon ageing or neurodegeneration may involve a similar targeting mechanism of ‘foreign-looking’ or unfit cells (Cao et al., 2013; Kounatidis et al., 2017; Lezi et al., 2018).
Noteworthy, other negatively charged molecules enriched on tumour surface such as heparan sulfates and O-glycosylated mucins may also contribute to the targeting by AMPs (Hollingsworth and Swanson, 2004; Knelson et al., 2014).
Defensin action requires TNF
TNF is a major player in the tumour microenvironment by exerting pleiotropic effects on both the tumour and its surroundings (Ham et al., 2016; Parameswaran and Patial, 2010). In our dlg mutant model, we observed that defensin induction requires TNF, supporting previous observations that induction of innate immune response in tumour bearing animal relied on Egr produced by the tumour (Parisi et al., 2014). Indeed, Toll activation in the fat body has been proposed to be a consequence of tumour-derived TNF, which drives haemocyte proliferation leading to an increase in Toll ligand Spatzle (Parisi et al., 2014). Whether Imd pathway is also indirectly activated by the changes in immune cell activity in response to tumour or by alternative mechanisms remains an open question.
Our results show that haemocyte-derived TNF is required for PS exposure by the tumour, a key process for tumour targeting by Defensin. Consistently, haemocyte-derived TNF is shown to drive cell death in dlg mutant tumours (Parisi et al., 2014). The precise molecular mechanisms driving PS exposure downstream of TNF remain unknown. However, haemocyte-derived TNF, activates JNK pathway, which triggers many changes in tumour cells including apoptosis (Igaki et al., 2002; Moreno et al., 2002), ROS production (Fogarty et al., 2016; Santabárbara-Ruiz et al., 2015), loss of cell polarity (Zhu et al., 2010), modification of extracellular matrix (Uhlirova and Bohmann, 2006), proliferation and cell migration (Beaucher et al., 2007; Igaki et al., 2006; Pastor-Pareja et al., 2004; Srivastava et al., 2007). Mild activation of JNK on its own is insufficient to drive PS exposure and tissue sensitivity to Defensin-induced cell death (data not shown). While a JNK-independent role of Egr in PS exposure and sensitivity to the AMP cannot be ruled out, TNF might also sensitise cells through activation of the JNK pathway (Cordero et al., 2010) and PS exposure, thus providing a secondary sensitisation mechanism of tumour cells to the action of Defensin. Further studies are needed to explore a potential link between JNK activation and PS exposure.
Another consequence of TNF-dependent JNK activation in tumours is the increased expression of matrix metalloproteases (Mmps) by tumour cells (Uhlirova and Bohmann, 2006). Importantly, we show that Mmp-1 induction and then JNK pathway activation are still present in tumour from larvae devoided of Defensin. This demonstrates that TNF signalling acts upstream of Defensin. Mmps degrade the basal membrane facilitating metastasis of primary tumour cells (Beaucher et al., 2007; Pastor-Pareja et al., 2004; Srivastava et al., 2007). Interestingly, a study reported that human Mmp-7 can cleave immature Defensins, promoting their activation (Wilson et al., 1999; Wilson et al., 2009). Indeed, the pro-domain present in AMPs is thought to keep their in vivo activity silent, allowing local activation of AMP upon cleavage. While the mechanisms of Drosophila Defensin pro-domain cleavage remain unknown, it would be interesting to explore whether the changes in the tumour microenvironment could also affect Defensin activation.
In conclusion, our study provides the first direct in vivo demonstration of the role of an endogenous AMP as an anti-cancer agent in Drosophila. Our data point to a conserved mechanism of tumour control by AMPs, a potent arm of the innate immune system. Importantly, we identify cellular features within tumours, which may be predictive of their sensitivity to be targeted by AMPs. This study provides a new paradigm to decipher the molecular mechanisms influencing anti-tumoural functions of an AMP, which may extend to other non-canonical roles of AMPs, such as in ageing, long-term memory and wound healing. Moreover, together with the new genetic tools allowing targeting of all Drosophila AMPs (Hanson et al., 2019), our study establishes new bases to explore in vivo a potential important natural mechanism of defence against tumours.
Materials and methods
Reagent type (species) or resource | Designation | Source or reference | Identifiers | Additional information |
---|---|---|---|---|
Genetic reagent (Drosophila melanogaster) | w1118 | (Dewey et al., 2004) | BDSC: 3605; RRID:BDSC_3605 | |
Genetic reagent (Drosophila melanogaster) | w1118 iso | (Ferreira et al., 2014) | N/A | |
Genetic reagent (Drosophila melanogaster) | dlg40.2/FM7 | (Mendoza-Topaz et al., 2008) | Flybase_FBal0240608 | |
Genetic reagent (Drosophila melanogaster) | FRT82B,scrib1/TM6 | (Bilder et al., 2000) | Flybase_FBal0103577 | |
Genetic reagent (Drosophila melanogaster) | egr3 | (Igaki et al., 2002) | Flybase_FBal0147163 | |
Genetic reagent (Drosophila melanogaster) | imd1 | (Leulier et al., 2000) | Flybase_ FBal0045906 | |
Genetic reagent (Drosophila melanogaster) | relE20 | (Leulier et al., 2000) | DGGR: 109927; RRID:DGGR_109927 | |
Genetic reagent (Drosophila melanogaster) | defsk3 | (Hanson et al., 2019) | N/A | |
Genetic reagent (Drosophila melanogaster) | defsk4 | (Hanson et al., 2019) | N/A | |
Genetic reagent (Drosophila melanogaster) | btl-gal4,UAS-RFP/CyO | Irene Miguel-Aliaga | N/A | |
Genetic reagent (Drosophila melanogaster) | lpp-gal4/TM6B | (Brankatschk and Eaton, 2010) | N/A | |
Genetic reagent (Drosophila melanogaster) | tub-gal4 | Bloomington Drosophila Stock Center | BDSC: 5138; RRID:BDSC_5138 | y(1) w[*]; P{w[+mC]=tubP-GAL4}LL7/TM3, Sb(4) Ser(1) |
Genetic reagent (Drosophila melanogaster) | hmlΔ-gal4,UAS-gfp | Bruno Lemaitre | N/A | |
Genetic reagent (Drosophila melanogaster) | en-gal4 | Bloomington Drosophila Stock Center | BDSC: 30564; RRID:BDSC_30564 | y1 w*; P{w + mW.hs=en2.4 GAL4}e16E |
Genetic reagent (Drosophila melanogaster) | UAS-def IR | Vienna Drosophila Resource Centre | VDRC: 102437; RRID:Flybase_FBst0474306 | P{KK111656}VIE-260B |
Genetic reagent (Drosophila melanogaster) | UAS-imd IR | Vienna Drosophila Resource Centre | VDRC: 101834; RRID:Flybase_FBst0473707 | P{KK109863}VIE-260B |
Genetic reagent (Drosophila melanogaster) | UAS-myd88 IR | Vienna Drosophila Resource Centre | VDRC: 25402; RRID:Flybase_FBst0455868 | w1118; P{GD9716}v25402 |
Genetic reagent (Drosophila melanogaster) | UAS-dlg IR | Vienna Drosophila Resource Centre | VDRC: 41136; RRID:Flybase_FBst0463952 | w1118; P{GD4689}v41136/TM3 |
Genetic reagent (Drosophila melanogaster) | UAS-egr IR | Vienna Drosophila Resource Centre | VDRC: 108814; RRID:Flybase_FBst0480608 | P{KK103432}VIE-260B |
Genetic reagent (Drosophila melanogaster) | UAS-w IR | Bloomington Drosophila Stock Center | BDSC: 25785; RRID:BDSC_25785 | y(1) v(1); P{y[+t7.7] v[+t1.8]=TRiP.JF01786}attP2 |
Genetic reagent (Drosophila melanogaster) | UAS-def | (Tzou et al., 2000) | Flybase_FBal0145092 | |
Genetic reagent (Drosophila melanogaster) | UAS-def-3xHA | FlyORF | FlyORF: F002467; RRID:Flybase_FBal0298643 | M{UAS-Def.ORF.3xHA.GW}ZH-86Fb |
Genetic reagent (Drosophila melanogaster) | UAS-dcr2 | Bloomington Drosophila Stock Center | BDSC: 24650; RRID:BDSC_24650 | w[1118]; P{w[+mC]=UAS-Dcr-2.D}2 |
Genetic reagent (Drosophila melanogaster) | spzM7 | (Neyen et al., 2014) | N/A | |
Antibody | Anti-GFP (Chicken polyclonal) | Abcam | Cat# ab13970; RRID:AB_300798 | IF(1:4000) |
Antibody | Anti-Def (Mouse polyclonal) | Dahua Chen (Ji et al., 2014) | N/A | IF(1:100) |
Antibody | Anti-HA (Mouse monoclonal) | Cell Signaling Technology | Cat# 2367, RRID:AB_10691311 | IF(1:1000) |
Antibody | Anti-dcp1 (Rabbit polyclonal) | Cell Signaling Technology | Cat# 9578, RRID:AB_2721060 | IF(1:100) |
Antibody | Anti-phospho-Histone H3 (Ser10) (Rabbit polyclonal) | Cell Signaling Technology | Cat# 9701, RRID:AB_331535 | IF(1:100) |
Antibody | Anti-phospho-Histone H3 (Ser28) (Rabbit polyclonal) | Cell Signaling Technology | Cat# 9713, RRID:AB_823532 | IF(1:100) |
Antibody | Anti-Mmp1 (Mouse clonality unknown) | Developmental Studies Hybridoma Bank | Cat# 3B8D12, RRID:AB_579781 | IF(1:10) |
Antibody | Anti-Chicken IgY Alexa 488 (Goat polyclonal) | Molecular Probes | Cat# A-11039, RRID:AB_142924 | IF(1:500) |
Antibody | Anti-Mouse IgG Alexa 488 (Goat polyclonal) | Molecular Probes | Cat# A-11029, RRID:AB_138404 | IF(1:500) |
Antibody | Anti-Mouse IgG Alexa 594 (Goat polyclonal) | Molecular Probes | Cat# A-11032, RRID:AB_141672 | IF(1:500) |
Antibody | Anti-Rabbit IgG Alexa 488 (Goat polyclonal) | Molecular Probes | Cat# A-11008, RRID:AB_143165 | IF(1:500) |
Antibody | Anti-Rabbit IgG Alexa 594 (Goat polyclonal) | Thermo Fischer Scientific | Cat# A-11037, RRID:AB_2534095 | IF(1:500) |
Sequence-based reagent | rpl32-fwd | This paper | PCR primers | AGGCCCAAGATCGTGAAGAA |
Sequence-based reagent | rpl32-rev | This paper | PCR primers | TGTGCACCAGGAACTTCTTGA |
Sequence-based reagent | def-fwd | This paper | PCR primers | CTTCGTTCTCGTGGCTATCG |
Sequence-based reagent | def-rev | This paper | PCR primers | ATCCTCATGCACCAGGACAT |
Sequence-based reagent | def-PCR-fwd | This paper | PCR primers | TTATTGCAGAAACGGGCTCT |
Sequence-based reagent | def-PCR-rev | This paper | PCR primers | ATGGTAAGTCGCTAACGCTAATG |
Sequence-based reagent | def-seq | This paper | Sequencing primers | CGTGTCTTCCTGCACAGAAA |
Sequence-based reagent | attA-fwd | This paper | PCR primers | ATGCTCGTTTGGATCTGACC |
Sequence-based reagent | attA-rev | This paper | PCR primers | TCAAAGAGGCACCATGACCAG |
Sequence-based reagent | cecA1-fwd | This paper | PCR primers | CTCAGACCTCACTGCAATAT |
Sequence-based reagent | cecA1-rev | This paper | PCR primers | CCAACGCGTTCGATTTTCTT |
Sequence-based reagent | dro-fwd | This paper | PCR primers | CGTTTTCCTGCTGCTTGCTT |
Sequence-based reagent | dro-rev | This paper | PCR primers | GGCAGCTTGAGTCAGGTGAT |
Sequence-based reagent | drs-fwd | This paper | PCR primers | CTCTTCGCTGTCCTGATGCT |
Sequence-based reagent | drs-rev | This paper | PCR primers | ACAGGTCTCGTTGTCCCAGA |
Peptide, recombinant protein | Drosophila endogenous Defensin | Bulet EIRL | N/A | |
Peptide, recombinant protein | Drosophila Synthetic Defensin | Genepep | N/A | ATCDLLSKWNWNHTACAGHCIAKGFKGGYCNDKAVCVCRN |
Commercial assay or kit | High Capacity cDNA Reverse Transcription Kit | Applied Biosystems | Cat# 4368813 | |
Commercial assay or kit | PerfeCTa SYBR Green FastMix (Low ROX) | Quanta Bio | Cat# 95074–012 | |
Commercial assay or kit | TRIzol Reagent | Thermo Fisher Scientific | Cat# 15596018 | |
Commercial assay or kit | Turbo DNA free Kit | Life Technologies LTD | Cat# AM1907 | |
Commercial assay or kit | High Capacity cDNA Reverse Transcription Kit | Applied Biosystems | Cat# 4368813 | |
Chemical compound, drug | 4′,6-Diamidine-2′-phenylindole dihydrochloride (DAPI) | Sigma | Cat# D9542 | 1 μg/mL |
Software, algorithm | Fiji | NIH | https://fiji.sc/ | |
Software, algorithm | GraphPad Prism 6 | GraphPad | RRID:SCR_002798 | |
Software, algorithm | 7500 Real-Time PCR Software | Applied Biosystems | RRID:SCR_014596 | |
Software, algorithm | BatchQuantify | (Johansson et al., 2019) | https://github.com/emltwc/2018-Cell-Stem-Cell | |
Software, algorithm | GraphPad Prism 6 | GraphPad | RRID:SCR_002798 | |
Software, algorithm | Volocity 3D Image Analysis Software | Perkin Elmer | RRID:SCR_002668 | |
Software, algorithm | ZEN two lite | Zeiss | RRID:SCR_013672 | |
Other | RNasine Plus RNase Inhibitor | Promega | Cat# N261 | |
Other | Vectashield mounting media | Vector Laboratories, Inc. | Cat# H-1000–10 | |
Other | Annexin V, Alexa Fluor 568 conjugate | Life Technologies LTD | Cat# A13202 | 1:20 |
Other | Annexin V, Alexa Fluor 488 conjugate | Life Technologies LTD | Cat# A13201 | 1:20 |
Other | Penicillin-Streptomycin (10,000 U/mL) | Thermo Fisher Scientific | Cat# 15140122 | Pen: 100 IU/mL Strep: 100 mg/mL |
Other | PfuUltra II Fusion HS DNA Polymerase | Agilent | Cat# 600670 | |
Other | AnnexinV binding buffer | Fisher Scientific | Cat# BDB556454 |
Drosophila housekeeping and rearing
Request a detailed protocolFlies were reared at 25°C under 12 hr/12 hr light/dark cycles on standard oatmeal and molasses medium (Fernandez-Ayala et al., 2009). Details on fly strains used in this study are presented in the key resources table and detailed genotypes are included in corresponding figure legends.
For assessment of tumour volume and tumour cell death, flies were transferred to medium embryo collection cages and allowed to lay eggs for 8 hr at room temperature (RT) on agar/grape juice plates (2.1% agar, 25% grape juice, 1.25% sucrose, 0.2% methyl 4-hydroxybenzoate) supplemented with yeast paste. Plates were kept at 25°C until hatching. 200 larvae hatched within a 4 hr time window were collected and transferred to rearing bottles containing 45 mL of fly food to avoid any developmental delay and/or starvation effects due to overcrowding conditions. The tumour phenotype was analysed close to the normal developmental time, i.e. 7 days after hatching. Tumours were dissected and stained for further analysis (see below).
Generation of defensin mutants
Request a detailed protocoldefsk3 and defsk4 mutants were generated using CRISPR/cas9 technology (Hanson et al., 2019). Mutants were isogenised through backcrosses with a w1118 iso line as previously described in Ferreira et al. (2014).
Survival assays
Request a detailed protocolSurvival of defSK3 animals was compared to wild type controls (isogenised background, iso). Male flies (5–7 days old) were pricked in the thorax with a needle dipped in a concentrated pellet of fresh overnight bacterial culture. Infected flies were kept at 29°C. Listeria innocua was cultured in Brain heart infusion medium at 37°C and used at optical density of 200 nm (OD600). Erwinia carotovora carotovora 15 was cultured in Luria-Bertani broth at 29°C and used at OD600 200. Experiments were repeated three times independently and one representative experiment is shown.
Immunohistochemistry
Request a detailed protocolTissues were dissected in Phosphate Buffer Saline (PBS) and fixed for 20 min in 4% formaldehyde. Fat body samples were fixed for 30 min using the same protocol. Tissues were washed three times in PBS containing 1% of Triton-X100 (PBT) and incubated overnight at 4°C with primary antibodies. Tissues were washed five times in PBT and incubated for 2 hr at RT with secondary antibodies and 4’,6-Diamidine-2′-phenylindole dihydrochloride (DAPI). After three washes in PBT, tissues were mounted in Vectashield using Secured-Seal spacers (Thermo Fisher Scientific).
Confocal images were captured using a Zeiss 710 or Zeiss 880 with Airyscan confocal microscope and processed with Fiji 2.0.0 or Adobe Photoshop C.S5.1.
Quantification of tumour volume and tumour cell death
Request a detailed protocolFor analysing tumour phenotype, images were acquired using optimal slice parameter and 12bits using a Zeiss 710 confocal microscope. Quantifications were made as previously described (Parisi et al., 2014). Briefly, we used Volocity 3D imaging analysis software to quantify the total volume of tumour identified by DAPI staining and cell death visualised with anti-Dcp1 staining. When haltere or leg discs tumours were still associated with the wing disc, they were not considered for quantification. Quantifications were done in at least three independent biological replicates for each genetic setting. Single representative experiments are presented except for dlg;def double mutant rescue experiments (Figure 2K–L) for which replicates are pooled due to the few larvae available. In all cases the difference between control and mutant tumour were strongly reproducible between replicates.
Quantification of colocalisation between Def and Dcp1 or Annexin V
Request a detailed protocolTo quantify Def/Dcp-1 and Def/Annexin V colocalisation, we determined the intensity of Def staining in areas of positive versus negative staining for the second marker using the ImageJ macro, BatchQuantify (Johansson et al., 2019).
Sterile rearing conditions
Request a detailed protocolTo rule out any effect of infection on the tumour phenotypes, Drosophila larvae were reared on standard food supplemented with penicillin and streptomycin when indicated in the figure.
qRT-PCR analysis
Request a detailed protocolTotal RNA from 7 to 10 whole larvae or tissues collected from 10 to 20 larvae (10 larvae for fat body, 20 larvae for trachea) was extracted using TRIzol according to the manufacturer’s instructions. RNA was treated with Turbo DNA free Kit and RNA concentration and quality were monitored using a Nanodrop (Thermo Fisher Scientific). The same amount of total RNA (1 μg for whole larvae and fat body or 100 ng for trachea) was used to perform the three independent reverse-transcriptions using the High-Capacity cDNA Reverse Transcription Kit. cDNAs were pooled and qPCR was performed using PerfeCTa SYBR green following the manufacturer’s instructions. cDNA amplification was monitored with Applied Biosystems 7500 fast instruments. Serial 10-fold dilutions of an external standard were used to produce a standard curve, and RNA samples were included to control for the absence of DNA contamination. rpl32 expression was used to normalise expression levels of target genes. Data was analysed using the Ct method (2-∆∆ct). All qPCR experiments were carried out on three to seven independent biological replicates (see figure legends for details) and, larvae were sampled from the same bottle used to analyse the tumour phenotype. GraphPad Prism7 software was used for graphical representation and statistical analysis. Primer targets and sequences are presented in key resources table.
PCR amplification of genomic DNA
Request a detailed protocolTo verify the sequence of defsk3 and defsk4 mutants, genomic DNA was extracted from single flies by grinding whole animals with a 200 μL pipette tip containing 50 μL of squishing buffer (10 mM Tris-HCl pH 8.2, 1 mM EDTA, 25 mM NaCl, 200 μg proteinase K) followed by incubation at 37°C for 30 min. Proteinase K was heat-inactivated at 95°C for 2 min. 1 μL of genomic DNA was used for PCR amplification using PfuUltra II Fusion DNA Polymerase and Eppendorf Mastercycler Ep Gradient Thermal Cycler.
Annexin V staining and quantification
Request a detailed protocolTumours were dissected in PBS and incubated in Annexin V binding buffer containing 5% of Alexa Fluor (488 nm or 568 nm) conjugated Annexin V for 10 min. Tissues were washed quickly in Annexin V binding buffer and fixed in 4% formaldehyde for 20 min. Immunostaining, mounting and imaging were then carried out as described above.
We used Fiji 2.0.0 software to quantify Annexin V staining at the tumour surface. Maximal Z-projection of whole tumours were generated and the Colour Threshold tool was then used to detect the red pixels that represented Annexin V staining at the surface of tumours. The hue slider was set to include only the red signal, and the brightness slider was adjusted to exclude any background signal. This area was then selected and measured in pixels.
Synthetic defensin production and injections
Request a detailed protocolThe mature Defensin peptide was synthesised (over 90% purity) and the purity controlled by Genepep using Ultra Performance Liquid Chromatography/Mass Spectrometry. We subsequently compared the integrity of synthetic Defensin with authentic Defensin purified from Drosophila hemolymph using two complementary approaches, namely Matrix-Assisted Laser Desorption/Ionisation-Mass Spectrometry (MALDI-MS) for molecular mass determination and trypsin digestion followed by nanoLiquid Chromatography/Electrospray Ionisation tandem Mass Spectroscopy (nanoLC/ESI MS/MS) for molecular integrity confirmation. Those analyses confirmed the similar mass between natural and synthetic Defensin (respectively provided by Bulet EIRL and Genepep) as well the presence of disulphide bonds within synthetic Def, demonstrating it is the mature peptide (Data not shown).
For injection experiments, larvae were collected, washed three times in cold sterile PBS and injected on a fly pad upon CO2 anaesthesia using Nanoject II (Drummond) and glass capillaries 3.5 inch (Drummond). Larvae were injected with 69 nL of PBS containing 7.5 nmol of synthetic Defensin or 69 nL of PBS only as control. Larvae were then gently transferred into agar/grape juice plates and kept at 25°C. Tumours were dissected 4 hr after injection and stained for visualisation of nuclei and tumour cell death as describe above.
Statistical analyses
Request a detailed protocolAll data are presented as mean ± SD and n values are indicated in the figure legends. Statistical analyses were carried out using GraphPad Prism7 software and only significant differences are indicated in dot-plots. Survival upon infection was analysed using Log-rank test. qPCR data were analysed using unpaired t-test with a two-tailed p value. Tumour volume and tumour cell death comparing two samples were analysed using Mann-Whitney test with a two-tailed p value and the one comparing three genotypes were analysed using One-way ANOVA followed by Turkey’s multiple comparisons test. Tumour volume from injected larvae was analysed using Two-way ANOVA followed by Turkey’s multiple comparisons test and only significant statistical differences between ctrl and Defensin injected larvae for each genotype tested are indicated.
Data and material availability
Request a detailed protocolAll raw data is available through http://dx.doi.org/10.5525/gla.researchdata.834.
Data availability
All raw data are available through http://dx.doi.org/10.5525/gla.researchdata.834. Owing to the size of the dataset, it can be requested through the link.
References
-
Antimicrobial peptides modulate long-term memoryPLOS Genetics 14:e1007440.https://doi.org/10.1371/journal.pgen.1007440
-
Metastatic ability of Drosophila tumors depends on MMP activityDevelopmental Biology 303:625–634.https://doi.org/10.1016/j.ydbio.2006.12.001
-
Phosphatidylserine is a global immunosuppressive signal in Efferocytosis, infectious disease, and cancerCell Death & Differentiation 23:962–978.https://doi.org/10.1038/cdd.2016.11
-
Lipoprotein particles cross the blood-brain barrier in DrosophilaJournal of Neuroscience 30:10441–10447.https://doi.org/10.1523/JNEUROSCI.5943-09.2010
-
Antimicrobial peptides: pore formers or metabolic inhibitors in bacteria?Nature Reviews Microbiology 3:238–250.https://doi.org/10.1038/nrmicro1098
-
Cancer-specific loss of beta-defensin 1 in renal and prostatic carcinomasLaboratory Investigation 83:501–505.https://doi.org/10.1097/01.LAB.0000063929.61760.F6
-
The diverse roles of the TNF axis in cancer progression and metastasisTrends in Cancer Research 11:1–27.
-
Drosophila innate immunity: an evolutionary perspectiveNature Immunology 3:121–126.https://doi.org/10.1038/ni0202-121
-
Mucins in cancer: protection and control of the cell surfaceNature Reviews Cancer 4:45–60.https://doi.org/10.1038/nrc1251
-
Eiger, a TNF superfamily ligand that triggers the Drosophila JNK pathwayThe EMBO Journal 21:3009–3018.https://doi.org/10.1093/emboj/cdf306
-
Biology of toll receptors: lessons from insects and mammalsJournal of Leukocyte Biology 75:18–26.https://doi.org/10.1189/jlb.0403160
-
Heparan sulfate signaling in cancerTrends in Biochemical Sciences 39:277–288.https://doi.org/10.1016/j.tibs.2014.03.001
-
Interplay among Drosophila transcription factors Ets21c, fos and Ftz-F1 drives JNK-mediated tumor malignancyDisease Models & Mechanisms 8:1279–1293.https://doi.org/10.1242/dmm.020719
-
Metchnikowin, a novel immune-inducible proline-rich peptide from Drosophila with antibacterial and antifungal propertiesEuropean Journal of Biochemistry 233:694–700.https://doi.org/10.1111/j.1432-1033.1995.694_2.x
-
Metabolic integration of bacterial endosymbionts through antimicrobial peptidesTrends in Microbiology 25:703–712.https://doi.org/10.1016/j.tim.2017.04.007
-
Tumor necrosis Factor-α signaling in macrophagesCritical Reviews in Eukaryotic Gene Expression 20:87–103.https://doi.org/10.1615/CritRevEukarGeneExpr.v20.i2.10
-
Drosophila as a Model System to Study Nonautonomous Mechanisms Affecting Tumour Growth and Cell DeathBioMed Research International 2018:1–13.https://doi.org/10.1155/2018/7152962
-
In search of a novel target — phosphatidylserine exposed by non-apoptotic tumor cells and metastases of malignancies with poor treatment efficacyBiochimica Et Biophysica Acta (BBA) - Biomembranes 1808:2638–2645.https://doi.org/10.1016/j.bbamem.2011.07.026
-
Convergent evolution of defensin sequence, structure and functionCellular and Molecular Life Sciences 74:663–682.https://doi.org/10.1007/s00018-016-2344-5
-
Caspase activity is required for engulfment of apoptotic cellsMolecular and Cellular Biology 33:3191–3201.https://doi.org/10.1128/MCB.00233-13
-
Immune-mediated dormancy: an equilibrium with cancerJournal of Leukocyte Biology 84:988–993.https://doi.org/10.1189/jlb.1107774
-
Phosphatidylserine recognition and induction of apoptotic cell clearance by Drosophila engulfment receptor draperThe Journal of Biochemistry 153:483–491.https://doi.org/10.1093/jb/mvt014
-
How Drosophila combats microbial infection: a model to study innate immunity and host-pathogen interactionsCurrent Opinion in Microbiology 5:102–110.https://doi.org/10.1016/S1369-5274(02)00294-1
-
Differential processing of {alpha}- and {beta}-Defensin Precursors by Matrix Metalloproteinase-7 (MMP-7)Journal of Biological Chemistry 284:8301–8311.https://doi.org/10.1074/jbc.M809744200
-
Mechanisms of antimicrobial peptide action and resistancePharmacological Reviews 55:27–55.https://doi.org/10.1124/pr.55.1.2
-
Antimicrobial peptides increase tolerance to oxidant stress in Drosophila melanogasterJournal of Biological Chemistry 286:6211–6218.https://doi.org/10.1074/jbc.M110.181206
Article and author information
Author details
Funding
Cancer Research UK (A17196)
- Julia B Cordero
Wellcome (104103/Z/14/Z)
- Julia B Cordero
The funders had no role in study design, data collection and interpretation, or the decision to submit the work for publication.
Acknowledgements
We would like to thank the Vienna Drosophila Resource Centre, the Bloomington Drosophila Stock Centre and Mirka Uhlirova for fly stocks. We are thankful to Core Services and Advanced Technologies at the Cancer Research UK Beatson Institute (C596/A17196), with particular thanks to Beatson Advanced Imaging Resource. We are very grateful to Dr. Dahua Chen for sharing the anti-Defensin antibody, to Dr. Sébastien Voisin for his critical contribution in mass spectrometry analyses and to Dr. Mate Naszai for critical help in the quantification of staining co-localization. JPP and YY are supported by Cancer Research UK core funding through the CRUK Beatson Institute (A17196). JBC is a Sir Henry Dale Fellow jointly funded by the Wellcome Trust and the Royal Society (grant number 104103/Z/14/Z). In memory of Marcos Vidal (1974–2016).
Copyright
© 2019, Parvy et al.
This article is distributed under the terms of the Creative Commons Attribution License, which permits unrestricted use and redistribution provided that the original author and source are credited.
Metrics
-
- 6,003
- views
-
- 875
- downloads
-
- 79
- citations
Views, downloads and citations are aggregated across all versions of this paper published by eLife.
Citations by DOI
-
- 79
- citations for umbrella DOI https://doi.org/10.7554/eLife.45061