Fgf4 maintains Hes7 levels critical for normal somite segmentation clock function
Abstract
During vertebrate development, the presomitic mesoderm (PSM) periodically segments into somites, which will form the segmented vertebral column and associated muscle, connective tissue, and dermis. The periodicity of somitogenesis is regulated by a segmentation clock of oscillating Notch activity. Here, we examined mouse mutants lacking only Fgf4 or Fgf8, which we previously demonstrated act redundantly to prevent PSM differentiation. Fgf8 is not required for somitogenesis, but Fgf4 mutants display a range of vertebral defects. We analyzed Fgf4 mutants by quantifying mRNAs fluorescently labeled by hybridization chain reaction within Imaris-based volumetric tissue subsets. These data indicate that FGF4 maintains Hes7 levels and normal oscillatory patterns. To support our hypothesis that FGF4 regulates somitogenesis through Hes7, we demonstrate genetic synergy between Hes7 and Fgf4, but not with Fgf8. Our data indicate that Fgf4 is potentially important in a spectrum of human Segmentation Defects of the Vertebrae caused by defective Notch oscillations.
Introduction
A common developmental mode employed by many embryos is a segmentation clock that oscillates within a posterior growth zone; with each cycle, a segment forms. This stratagem has evolved independently within the three major bilaterian clades (annelids, arthropods, and chordates) as well as in plants (Clark et al., 2019; Richmond and Oates, 2012; Chipman, 2010; Ten Tusscher, 2020; Balavoine, 2014). In chordates, a segmentation clock oscillates in the presomitic mesoderm (PSM); with each cycle, a pair of somites form flanking the neural tube (Pourquié, 2011; Hubaud and Pourquié, 2014). Somites differentiate into dermis, skeletal muscle, tendons, as well as the vertebral column, which retains the segmented attribute of the somites (Christ et al., 2007).
In vertebrates, genes with an oscillatory pattern within the PSM include those encoding components or targets of the FGF, WNT, and Notch signaling pathways (Hubaud and Pourquié, 2014; Krol et al., 2011; Dequéant et al., 2006). However, which individual genes oscillate differs among species, with the exception of the Notch-responsive HES/HER transcription factors, suggesting that Notch signaling is at the core of the somitogenesis clock (Krol et al., 2011). Supporting this idea, genetic and pharmacological manipulations demonstrate that Notch pathway oscillation in the mouse embryo is essential for somitogenesis (Ferjentsik et al., 2009). In the mouse, oscillatory waves of the Notch1 receptor and Delta-like 1 (Dll1) ligand expression, as well as the activated Notch receptor (cleaved Notch intracellular domain, NICD) sweep from the posterior to anterior, where oscillations arrest (Bone et al., 2014; Shimojo et al., 2016). These oscillations are established through negative-feedback loops of Notch components such as the transcriptional repressor, HES7 (Niwa et al., 2011), and the glycosyltransferase, LFNG (Morimoto et al., 2005), both of which are encoded by oscillating genes under Notch pathway regulation (Niwa et al., 2011). Notch oscillations arrest in the anterior PSM, where NICD cooperates with TBX6 to periodically activate Mesp2 expression (Oginuma et al., 2008). MESP2 then regulates formation of the nascent somite as well as its rostro-caudal patterning, which is essential for normal patterning of the subsequent vertebral column (Takahashi et al., 2000; Saga et al., 1997).
Mutations in nearly all of the aforementioned Notch pathway genes have been identified in human patients where defective somitogenesis is thought to be the cause of Segmentation Defects of the Vertebrae (SDV) (Eckalbar et al., 2012; Giampietro et al., 2009). For example, frequently recessive mutations in DLL3, HES7, MESP2 and LFNG and a dominant mutation in TBX6 (Sparrow et al., 2013; Lefebvre et al., 2017) have been identified in patients with spondylocostal dysostosis (SCDO), which is characterized by severe vertebral malformations that include hemivertebrae, vertebral loss and fusion along the length of the axis (Takeda et al., 2018). Whereas SCDO is relatively rare, congenital scoliosis (CS), defined as a lateral curvature of the spine exceeding 10%, is much more common, with a frequency of 1:1000, which is suspected to be an underestimation because asymptomatic individuals do not seek medical care (Giampietro et al., 2013). Mutated alleles of HES7, LFNG, MESP2, and TBX6 are all associated with CS (Lefebvre et al., 2017; Takeda et al., 2018; Giampietro et al., 2013; Sparrow et al., 2012).
The position in the anterior PSM where Notch oscillations arrest and Mesp2 expression establishes the future somite boundary is called the determination front (Morimoto et al., 2005; Saga et al., 1997; Dubrulle et al., 2001). This region is also the anterior limit of the wavefront in the classical clock-and-wavefront, a theoretical model proposed over 40 years ago to explain the precipitous and periodic formation of segments in the PSM (Cooke and Zeeman, 1976). In this model, wavefront activity prevents the PSM from responding to the segmentation clock; hence somites form only anteriorly, where the wavefront ends. In chick or zebrafish embryos, exogenously added FGF protein or pharmacological inhibition of FGF signaling will shift the determination front rostrally or caudally, respectively. In the mouse, Cre-mediated inactivation of Fgf4 and Fgf8 specifically in the PSM results in an initial expansion of Mesp2 expression, followed by the premature expression of somite markers throughout the PSM (Naiche et al., 2011). Canonical WNT signaling is also a wavefront candidate, mostly because ectopic activation of βcatenin in the PSM results in an expansion of this tissue and a rostral shift of Mesp2 expression (Aulehla et al., 2008; Dunty et al., 2008). However, this expansion observed in βcatenin ‘gain-of-function’ mutants requires FGF4 and FGF8 activity, suggesting that these FGF signals are synonymous with the wavefront (Naiche et al., 2011).
In addition to its role in preventing somite differentiation, FGF signaling is also implicated in the regulation of key Notch components of the segmentation clock. Several studies have demonstrated that pharmacological inhibition of FGF disrupts Notch oscillations (Wahl et al., 2007; Niwa et al., 2007). In mouse embryos with PSM-specific loss of FGF receptor one or both Fgf4 and Fgf8, Notch pathway genes such as Hes7, are downregulated, although this analysis can be complicated due to the loss of PSM tissue in these mutants (Naiche et al., 2011; Niwa et al., 2007). Here, we focus on FGF-Notch interactions by analyzing mouse mutants that lack only one of the wavefront Fgf genes, Fgf8 or Fgf4. An indispensable technique in our analysis is whole mount in situ hybridization chain reaction (HCR), which allows us to multiplex different gene expression domains and quantify mRNA levels within specific embryonic tissues (Choi et al., 2014; Choi et al., 2018; Trivedi et al., 2018). We demonstrate that, while Fgf8 is not required for somitogenesis, Fgf4 is required for normal Notch oscillatory patterns and patterning of the vertebral column.
Results
FGF4 activity is required in the PSM for normal segmentation of rostral somites
To analyze the role of Fgf4 or Fgf8 expression in the primitive streak and PSM (Figure 1A - B), we inactivated each gene specifically within these tissues using the transgenic mouse line, Tg(T-cre)1Lwd (hereafter 'TCre’), in which Cre is controlled by Brachyury regulatory elements (Perantoni et al., 2005). Thus, we generated ‘Fgf4 mutants’ (TCre; Fgf4 flox/Δ; ‘Δ' = ‘deleted’ or null) or ‘Fgf8 mutants’ (TCre; Fgf8 flox/Δ; see Table 1). Whereas Fgf8 mutants do not survive much beyond birth due to kidney agenesis (Perantoni et al., 2005), Fgf4 mutants are viable and found at Mendelian ratios at weaning (n = 18 controls and n = 21 mutants). Skeleton preparations of Fgf8 mutant embryos at E18.5 (n = 22) revealed all vertebral bodies were present and normally patterned. Fgf8 mutants also presented with minor cervical and/or lumbar homeotic transformations, each with incomplete penetrance and expressivity: small ribs were sometimes present in the most posterior cervical vertebra (8/22, 4 bilateral and 4 unilateral) or on the most anterior lumbar vertebra (8/22, 4 bilateral and 4 unilateral). On the other hand, Fgf4 mutants display a variety of segmentation defects in the cervical and thoracic vertebrae with 100% penetrance (Compare Figure 1D,D’ with C). An average of 7.9 defects occurred per mutant and consisted of hemivertebrae, and misshapen and deleted vertebrae (Figure 1E).
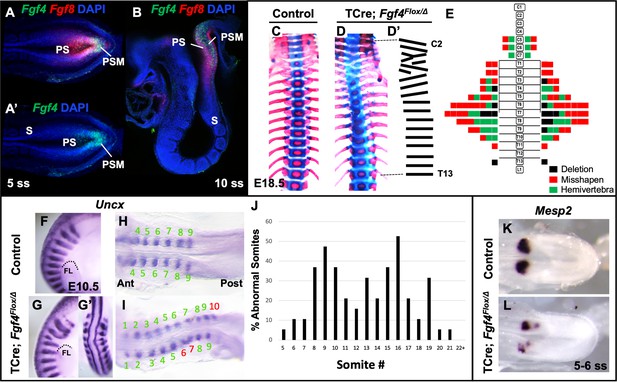
Fgf4 mutants have defects in vertebral and somite patterning.
(A, A’) Max intensity projection (MIP) of HCR staining of Fgf4 and Fgf8 mRNA expression (A) or Fgf4 only (A’) in the PSM of a five somite stage wildtype embryo (dorsal view). PS: primitive streak, PSM: presomitic mesoderm, S: somite. (B) MIP of 4 parasagittal z-sections (approximately 5 µm each) of HCR staining of Fgf4 and Fgf8 mRNA expression, of a 10 somite stage wildtype embryo (lateral view, mediolateral to the midline). (C, D) Skeletal preparations (ventral view after removing ribcage) of E18.5 control and Fgf4 mutants. (D’) Tracing of the Fgf4 mutant vertebral pattern in D. (E) Histograms representing the vertebral column and associated ribs (C = cervical, T = thoracic, L = lumbar), showing variety and location of vertebral defects in E18.5 Fgf4 mutants (n = 12). Each block in the histogram represents a single defect as indicated in the key, bottom right. (F–I) Wholemount in situ hybridization (WISH) detection of Uncx mRNA expression in control and Fgf4 mutants at E10.5 (F, G lateral views, anterior top; G’ dorsal view of embryo in G) and at 9–10 somite stage (H, I dorsal view, anterior left); dotted line in F and G marks the anterior boundary of the forelimb (FL). (J) Graph depicting the percentage of abnormal somites scored with a mispatterned Uncx mRNA WISH pattern (F–I) in Fgf4 mutants at the somite position listed on the x-axis. Somites were scored in 26–30 somite stage Fgf4 mutants (n = 19). Note that posterior to somite 21 the Uncx pattern is normal. (K, L) Dorsal view (anterior left) of 5–6 somite stage control (K) and Fgf4 mutant (L) embryos WISH-stained for Mesp2 mRNA expression. In all control embryos (n = 7), the Mesp2 pattern was normal; in 5/8 mutant embryos, the pattern was abnormal.
Experimental Crosses.
Experimental cross | Experimental genotype (Freq) | Control genotype (Freq) |
---|---|---|
Fgf4flox/flox x TCreTG/TG; Fgf4Δ/wt | TCreTG/0; Fgf4flox/Δ (1/2) | TCreTG/0; Fgf4flox/wt (1/2) |
Fgf8flox/flox x TCreTG/TG; Fgf8Δ/wt | TCreTG/0; Fgf8flox/Δ (1/2) | TCreTG/0; Fgf8flox/wt (1/2) |
Hes7Δ/wt x Hes7Δ/wt | Hes7Δ/Δ (1/4) | Hes7wt/wt (1/4) |
Fgf4flox/flox; Hes7Δ/wt x TCreTG/TG; Fgf4Δ/wt | TCreTG/0; Fgf4flox/Δ; Hes7Δ/wt (1/4) | TCreTG/0; Fgf4flox/wt (1/4) TCreTG/0; Fgf4flox/wt; Hes7Δ/wt (1/4) TCreTG/0; Fgf4flox/Δ (1/4) |
Fgf8flox/flox; Hes7Δ/wt x TCreTG/TG; Fgf8Δ/wt | TCreTG/0; Fgf8flox/Δ; Hes7Δ/wt (1/4) | TCreTG/0; Fgf8flox/wt (1/4) TCreTG/0; Fgf8flox/wt; Hes7Δ/wt (1/4) TCreTG/0; Fgf8flox/Δ (1/4) |
-
Experimental cross is always written ‘female x male’.
Δ = ‘deletion’ or null.
We examined gross somite patterning in Fgf4 mutants by staining embryos at various stages for Uncx mRNA, which marks the posterior somite compartment (Mansouri et al., 1997; Neidhardt et al., 1997). Analysis of 19 E9.5 Fgf4 mutants revealed a frequency of irregular Uncx expression specifically in future cervical and thoracic somites with full penetrance (Figure 1F–J). We did not score the most rostral somites in these samples because Uncx expression is absent in these early somites due to the differentiation of somites 1–4 to form the occipital bones (Spörle and Schughart, 1997). To examine these rostral somites we scored 20 Fgf4 mutants at somite stage seven and found defective Uncx patterning beginning at somite three in this sample set (black bars, Figure 1—figure supplement 1). Interestingly, a majority of Uncx patterning defects in somite 5 and 6 had apparently corrected by somite stage 25 (gray bars, Figure 1—figure supplement 1). Hence, these somites partially corrected or regulated their pattern and may account for the relatively low level of rostral vertebral defects at E18.5 (Figure 1E). This correction of rostral somite patterning is found in embryos lacking Hes7 expression (Bessho, 2001), which, as we show below, is reduced in Fgf4 mutants.
To determine if these malformed somites were due to an error in segmentation, we examined Mesp2 expression, which occurs in the anterior PSM and is required for normal somite segmentation and rostral-caudal somite identity (Saga et al., 1997). At the stages when irregular somites are emerging from Fgf4 mutant PSM, we detected aberrant Mesp2 expression, whether we analyzed gene expression by traditional wholemount in situ hybridization (WISH) staining (Figure 1K–L) or by fluorescent HCR analysis (Figure 2A,B). However, at later stages (22–24 somite stages), when properly segmented somites are emerging from Fgf4 mutant PSM, Mesp2 expression appears normal (Figure 1—figure supplement 2).

Fgf4 mutants maintain a normal wavefront.
(A, B) HCR staining of 5–6 somite stage control (A, n = 8) and Fgf4 mutant embryos (B, n = 11). In mutants, Msgn1 expression was similar between mutants and controls, but Mesp2 expression was abnormal (6/11). (C–D’’) HCR staining of 5–6 somite stage control and Fgf4 mutant embryos showing normal expression of Meox1 (control, n = 4; mutant, n = 4), Msgn1 (control, n = 9; mutant, n = 8), and Tbx6 (control, n = 10; mutant, n = 8). (E) There is no significant difference in the anterior-posterior length of Tbx6 and Msgn1 domains in control (n = 9) and mutant embryos (n = 8; green and red bars in C and C’). (F) Quantification of Tbx6 and Msgn1 mRNA expression, determined by measurement of fluorescence intensity per cubic micrometer within the volume of the Msgn1 or Tbx6 domain of expression, respectively. There is no significant difference between control and mutant embryos. In E and F, data are mean ± s.e.m, significance determined by a Student’s t-test. Msgn1: control n = 7; mutant n = 8. Tbx6: control, n = 9; mutant, n = 8. All images: MIP, dorsal view, anterior left. Same embryo shown in C-C’’ and D-D’’.
We considered whether this normal Mesp2 expression (Figure 1—figure supplement 2) and the resulting proper somite patterning caudal to somite 22 that occurs in Fgf4 mutants (Figure 1J), might reflect an absence of PSM-specific Fgf4 expression in normal development at later stages. However, we detected Fgf4 expression throughout the PSM at somite stage 22 through E12.5 (Figure 1—figure supplement 3C,C’, E–G’). We then considered whether TCre-mediated inactivation of Fgf4 resulted in loss of Fgf4 expression in the PSM throughout development. We expected this would be the case because TCre is active in the primitive streak at E7.5 (Perantoni et al., 2005) and, in prior studies, has been shown to inactivate Fgf4 and Fgf8 in the primitive streak and PSM, prior to formation of the first somite (Naiche et al., 2011; Perantoni et al., 2005). Indeed, we detect no Fgf4 expression in the PSM at somite stage 6 (Figure 1—figure supplement 3B,B’), when aberrant somites form, nor at somite stage 24 (Figure 1—figure supplement 3D,D’), when normal somites form. Therefore, we conclude that Fgf4 is expressed within the PSM throughout development, but is required for normal somitogenesis only at early stages. When Fgf4 is inactivated early, in the caudal embryo, abnormally patterned Mesp2 expression occurs only at these early stages, causing the observed vertebral defects in Fgf4 mutants.
Wavefront gene expression is normal in Fgf4 mutants
For the rest of our mRNA expression analysis, we mostly relied on whole mount in situ HCR in conjunction with Ce3D+ tissue clearing. Because the fluorescent signal intensity corresponds proportionally to the number of mRNA molecules (Trivedi et al., 2018), HCR allows for quantitative and multiplexed mRNA detection (Choi et al., 2018). Accurate quantification of the HCR signal requires a method such as Ce3D+ tissue clearing (see Materials and methods), which allows for imaging in deep tissues without signal loss.
We first addressed why a distinct and symmetrical band of Mesp2 expression fails to form in Fgf4 mutants. Mesp2 expression is normally triggered by activated Notch (NICD) and TBX6 at the determination front in the anterior PSM, where cells fall below a critical threshold of wavefront FGF signaling (Oginuma et al., 2008). We previously showed that this FGF activity was encoded by Fgf4 or Fgf8; if we inactivated both Fgfs with TCre (Fgf4/Fgf8 double mutant), Mesp2 expression is transiently activated throughout the PSM, which then aberrantly expresses the paraxial mesoderm marker, Meox1 (Naiche et al., 2011). To determine if the wavefront position is altered in Fgf4 mutants we examined the anterior-posterior length and expression levels of genes responsive to this activity at early somite stages when aberrant Mesp2 expression occurs. Both Msgn1 and Tbx6 are required to specify the paraxial mesoderm (Chapman and Papaioannou, 1998; Nowotschin et al., 2012; Chalamalasetty et al., 2011), and are silenced in the absence of wavefront activity in Fgf4/Fgf8 double mutants (Naiche et al., 2011). Neither the length nor level of expression of Tbx6 and Msgn1 were significantly altered in Fgf4 mutants (Figure 2C,C”, D,D”, E,F). Consistent with this observation, the paraxial differentiation marker, Meox1, was not expanded into the PSM (Figure 2C’ and D’), as occurs in Fgf4/Fgf8 double mutants (Naiche et al., 2011).
Therefore, we conclude that wavefront activity is normal in Fgf4 mutants, an insight consistent with the observation that normal axis extension occurs in these mutants, with no loss of caudal vertebrae (Anderson et al., 2016a). We surmise that this normal wavefront position and signaling in Fgf4 mutants are likely maintained by Fgf8, which is expressed at normal levels (Figure 3A–C) and is sufficient for maintaining normal expression levels of the FGF target genes, Spry2, Etv4, and Spry4 (Figure 3D–F’’’); these FGF targets are silenced in Fgf4/Fgf8 double mutants (Naiche et al., 2011). Therefore, neither a change in wavefront activity nor a change in determination front position explains the aberrant pattern of Mesp2 expression in Fgf4 mutants.
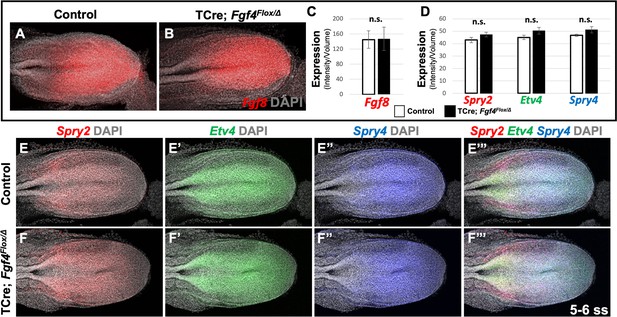
Fgf8 is sufficient for maintaining canonical FGF-responsive gene expression in Fgf4 mutants.
(A, B) HCR staining of 5–6 somite stage control (n = 6) and Fgf4 mutant (n = 3) embryos showing comparable expression of Fgf8, quantified in (C). (C) Quantification of Fgf8 mRNA expression, determined by measurement of fluorescent intensity per cubic micrometer within the volume the mesoderm- specific Tbx6 expression domain. Note, there is no significant difference between control and mutant embryos. (D) Quantification of HCR analysis of Spry2, Etv4, and Spry4 expression in 5–6 somite stage control (n = 4) and Fgf4 mutant (n = 4) embryos imaged in (E–F’’’). Data in C and D are mean ± s.e.m, significance determined by a Student’s t-test. All images: MIP, dorsal view, left. Same embryo shown in E- E’’’ and F- F’’’.
Oscillation of Notch family components is altered in the PSM of Fgf4 mutants
We then examined the pattern of activated Notch in Fgf4 mutants by immunostaining for Notch intracellular domain (NICD), which is an obligate factor in the transcriptional complex that activates Mesp2 (Takahashi et al., 2000). Overall NICD levels were unchanged between Fgf4 mutants and littermate controls at the embryonic stages when somitogenesis was abnormal (Figure 4A). At these stages, we always observed two to three distinct stripes of NICD along the anterior-posterior axis in both mutant and littermate control PSM (Figure 4B–C), demonstrating the oscillatory nature of Notch activation (Bone et al., 2014; Morimoto et al., 2005; Huppert et al., 2005). However, mutant oscillatory stripes of NICD were always less ordered, compared to controls. This was more evident when the relative intensities of NICD immunostaining signals were modeled using Imaris software. In controls, cells medial-lateral to each other had similar levels of activated NICD, whereas in mutants, this organization was less distinct (Figure 4B’–C’). Importantly, this blurred pattern occurred in the anterior PSM, where NICD activates Mesp2 expression at the determination front (Figure 4B’–C’, brackets).
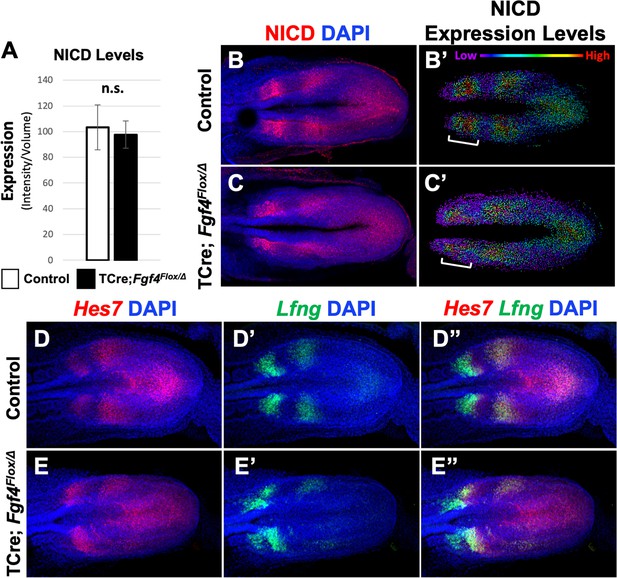
Pattern of Notch signaling is abnormal in Fgf4 mutants.
(A) Quantification of immunostained NICD fluorescent signal specifically in the mesoderm of 5–6 somite stage embryos. Note, there is no significant difference between Fgf4 mutants (n = 5) and littermate controls (n = 5). Data are mean ± s.e.m, significance determined by a Student’s t-test. (B, C) 5–6 somite stage control (B) or Fgf4 mutant (C) embryos immunostained for NICD. (B’ C’) Same images as in (B,C) but visualized using the Imaris spot modeling function where each fluorescent signal is represented as a colored sphere according to pixel intensity (lowest value is purple and highest value is red, as indicated). Note that the pattern in the Fgf4 mutant (C’) is less distinct compared to littermate control (B’); brackets indicate anterior PSM. (D–E’’) HCR staining of representative 5–6 somite stage control (n = 10) and Fgf4 mutant (n = 8) embryos for the indicated genes. Note Fgf4 mutant expression of Hes7 and Lfng in mutants is indistinct. All images: MIPs, dorsal view, anterior left. Same embryo shown in B, B’; C, C’; D-D’’; E-E’’.
A synchronous oscillatory NICD pattern is achieved through negative-feedback loops controlled by Lfng Morimoto et al., 2005) and Hes7 (Niwa et al., 2011). Lfng encodes a glycosyltransferase that represses Notch signaling in the PSM (Ferjentsik et al., 2009; Dale et al., 2003; Okubo et al., 2012), possibly via glycosylation of DLL1 and/or DLL3 (Panin et al., 2002; Serth et al., 2015). Hes7 is a basic helix-loop-helix transcription factor that is essential for repression of a number of Notch target genes (Niwa et al., 2007; Bessho, 2001; Chen et al., 2005). Hes7 and Lfng are themselves targets of activated Notch and therefore their expression oscillates (Niwa et al., 2011). Disruption of either gene causes uncoordinated Notch signaling in the PSM (Niwa et al., 2011).
Although oscillatory expression of Hes7 and Lfng are apparent in both Fgf4 mutants and littermate controls (Figure 4D–E’’), the mutant patterns are indistinct, similar to the mutant NICD pattern (Figure 4D–E’’). Lfng expression is particularly disordered in the anterior PSM (Figure 4E’ and E’’; Figure 4—figure supplement 1), where both NICD and Mesp2 also show aberrant patterning (Figure 4C,C’ and Figures 1L and 2B). Hes7 expression is likewise disordered in the mutant PSM at these stages (Figure 4E,E’’). Together, these data suggest the aberrant Mesp2 pattern in Fgf4 mutants can be explained by loss of coordinated Notch signaling at the differentiation front.
Hes7 expression is reduced in the PSM of Fgf4 mutants
We proceeded to focus on the expression of Hes7 in greater detail in Fgf4 mutants. The transcriptional repressor encoded by Hes7 is considered to be a fundamental pacemaker of the segmentation clock that controls somitogenesis (Kageyama et al., 2012). Hes7 is within the Hes/Her class of transcriptional repressors that are the only oscillating clock genes conserved among mouse, chicken, and zebrafish (Krol et al., 2011). In the mouse, mutations that accelerate HES7 production will accelerate the tempo of the segmentation clock (Harima et al., 2013). Moreover, HES7 directly represses Lfng transcription (Chen et al., 2005) and is required for normal Lfng oscillations within the PSM (Ferjentsik et al., 2009; Bessho, 2001; Dale et al., 2003; Okubo et al., 2012). Therefore, defective Hes7 expression could account for the abnormal NICD and Mesp2 patterning in Fgf4 mutants, possibly by causing aberrations in Lfng expression.
Analysis of Hes7 mRNA in Fgf4 mutants at 5–6 somite stages, using conventional WISH, revealed a reduced and aberrant expression pattern in Fgf4 mutants (Figure 5—figure supplement 1). Analysis with HCR was much more informative, allowing unambiguous pattern analysis (Figure 4) and quantification (Figure 5, Figure 5—figure supplement 2, Figure 5—figure supplement 3). Characterization of an oscillatory gene expression pattern in the PSM is achieved by classifying static expression patterns into three phases (Pourquié and Tam, 2001). This approach has been used to characterize Hes7 expression at E9.5-E10.5, when one to two stripes of expression are observed (Bessho, 2001; Bessho et al., 2003). However, in the E8.5 PSM, when aberrant Notch signaling occurs in Fgf4 mutants, there are two to three Hes7 expression stripes in both controls and Fgf4 mutants (Figures 4 and 5). Therefore, we generated criteria for classification of phases at E8.5 as follows. Phase I: three stripes with the most posterior stripe limited to the posterior midline and the most anterior stripe having reached the anterior boundary of the PSM (Figure 5A). Phase II: two stripes with the posterior stripe having expanded laterally compared to phase I and the anterior stripe having not reached the anterior limit of the PSM (Figure 5B). Phase III: two distinct stripes and a third stripe initiating at the posterior midline (Figure 5C). Control embryos are distributed nearly equally between each phase (Figure 5G) as is the case for Hes7 expression in older embryos (Bessho, 2001; Bessho et al., 2003). It is challenging to place Fgf4 mutants in phases, emphasizing the aberrant Hes7 expression pattern. However, in our assessment, we allocated Fgf4 mutants within all three phases (Figure 5D–F), with most found in oscillation phase II (Figure 5G).
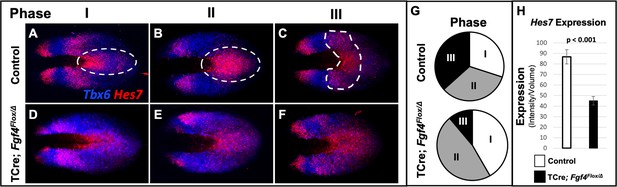
Hes7 expression is abnormally patterned and reduced in Fgf4 mutants.
(A–F) HCR staining for Hes7 expression in 5–6 somite stage control and Fgf4 mutant embryos sorted by phase of oscillation, showing abnormal expression of Hes7 in mutants. (G) Distribution of phases in control and Fgf4 mutants shown in A-F; controls: phase I, n = 9; phase II, n = 10; phase III, n = 11. Fgf4 mutants: phase I, n = 6; phase II, n = 9; phase III, n = 2. (H) Quantification of Hes7 expression specifically within the PSM (all phases combined) in 5–6 somite stage control (n = 11) and Fgf4 mutant (n = 8) embryos shows significantly decreased expression in the Fgf4 mutant. Quantification of Hes7 mRNA expression, determined by measurement of fluorescence intensity per cubic micrometer within the volume the mesoderm-specific Tbx6 expression domain. Data in H are mean ± s.e.m, significance determined by a Student’s t-test. All images are MIPs, dorsal view, anterior to left.
Hes7 is expressed in both the PSM and neural ectoderm. To quantify expression only in the PSM, we used Imaris software to create a volumetric model of the PSM, based on Tbx6 expression, which is limited to the PSM at these stages (Chapman et al., 1996). By measuring the intensity of the Hes7 HCR signal within this volume, we obtained a PSM-specific Hes7 quantification for each embryo (see Figure 5—figure supplement 3 and Material and methods). PSM-specific Hes7 expression is reduced in Fgf4 mutants to 51.8% of that expressed in litter-mate controls (Figure 5H). A comparison between mutant and control Hes7 expression levels within each phase reveals that the reduction in Fgf4 mutants is not due to the difference in phase allocation between Fgf4 mutants and littermate controls (Figure 5—figure supplement 4). Moreover, after the 22 somite stage, when forming somites are normally segmented in mutants (Figure 1J), there is no significant difference in Hes7 PSM expression levels between Fgf4 mutants and controls (Figure 5—figure supplement 5). Therefore, we conclude that a reduction of Hes7 expression may account for the defective segmentation during rostral somitogenesis in Fgf4 mutants.
To correlate Hes7 expression levels with pattern, we created Imaris-generated spot models of the HCR signal, which correspond to a single mRNA molecule or clusters of mRNA molecules within a subcellular-volume (see Materials and methods). We then colored-coded individual spots to reflect the intensity of the Hes7 HCR signal, using a linear series of intensity-based cutoffs every 20% to generate five colors (quintiles) (Figure 6A–B). In controls, the lowest intensity quintile (blue) contains the most spots (40%) and each higher-intensity group contains 10% fewer spots (Figure 6A, white bars in C). The distribution of spots in the Fgf4 mutant, compared to controls, is significantly skewed towards the lowest quintile at the cost of higher-level expression spots (Figure 6B, black bars in C). This modeling provides an effective illustration of the pattern of Hes7 expression.
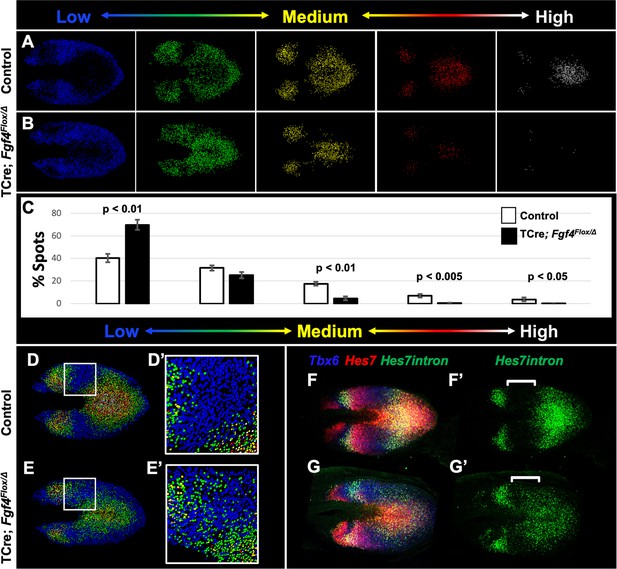
Reduced Hes7 expression with less distinct peaks and troughs in the Fgf4 mutant PSM.
(A, B) Spot models based on HCR analysis of Hes7 expression, in 5–6 somite stage control and Fgf4 mutant embryos within the PSM, as defined by the Tbx6 expression domain. Localized Hes7 expression is colored by level of expression from low (blue) to high expression (white), as indicated. Note there are less high expressors (yellow, red and white) and more low expressors (blue). This insight is quantified in (C), where the percentage of spots found in each expression-level group is graphed. Data are mean ± s.e.m, significance determined by a Student’s t-test; control, n = 6; mutant, n = 5. (D–E’) Composite of spot models from A and B. Note that the Hes7 expression trough (boxed region, expanded in D’ and E’) between anterior and posterior oscillatory peaks is less distinct in mutant (E’) with more higher expressors (green) than in control embryos (D’). (F–G’) MIP of HCR staining of 5–6 somite stage control and Fgf4 mutant embryos using probes against Tbx6, Hes7, and Hes7intron which specifically labels active Hes7 transcription. Note the trough of Hes7intron signal between peaks (brackets) in mutants is less pronounced than controls, indicating a failure of transcriptional repression of Hes7 in this region. Representative embryos are shown; control, n = 6; mutant, n = 5. All images are dorsal views, anterior left. Same embryo shown in F, F’; G, G’.
In control embryos, each stripe of Hes7 expression contains a concentric gradient of signal intensity with highest expression at the center (white spots) and lowest expression at the outside (blue spots) (Figure 6A,D). Fgf4 mutants maintain some of this concentric organization, but with a greater variability of expression between neighboring cells, the boundaries between groups is less clear (Figure 6B,E). In particular the trough between peaks of Hes7 expression is more shallow, and contains more higher-intensity spots (mostly second quintile, green) than littermate controls (Figure 6D’ and E’). To determine if this indicated a failure to repress Hes7 transcription in these regions, we performed HCR using a probe that hybridizes to the Hes7 intronic sequences, and therefore specific to newly transcribed pre-mRNA. This analysis revealed that transcription of Hes7 is reduced in the posterior PSM and is more widespread, extending into the trough between Hes7 mRNA peaks in the Fgf4 mutant (white brackets in Figure 6F’, G’). Therefore, we hypothesized that FGF4 is required to maintain Hes7 transcription in the PSM above a threshold required for normal patterns of Notch gene oscillation. The reduced level of HES7 in Fgf4 mutants is insufficient to fully autorepress its own expression, resulting in an aberrant pattern of expression.
A synergistic defect in Fgf4/Hes7 mutants reveals that Fgf4 is required to maintain Hes7 above a critical threshold
To test our hypothesis that a reduction of Hes7 expression causes the Fgf4 mutant vertebral defects, we asked if these defects worsen if we further reduce Hes7 expression by removing one gene copy. We compared such mutant to littermate controls that were simple Fgf4 mutants (with two wildtype Hes7 alleles) or Hes7 heterozygotes. These Hes7 heterozygotes also carried TCre and one floxed Fgf4 allele (see Table 1, and Figure 7) resulting in Fgf4 heterozygosity in the TCre expression domain. However, Fgf4 heterozygosity had no effect on the phenotype because the defects we observed were similar to the Hes7 heterozygous defects reported by the Dunwoodie lab (Sparrow et al., 2012). About 50% of our Hes7 heterozygotes had defective lower thoracic vertebrae with a frequency of 2.8 defects per animal. In littermate Fgf4 mutants, vertebral defects were completely penetrant, with an average of 7.7 defects per animal, a frequency similar to that of progeny in our original genetic cross (Figure 1). However, compound Fgf4/Hes7 mutants displayed a large set of defects with 25 defects per animal (Figure 7C–E), a frequency significantly greater than littermate Fgf4 mutants (3-fold greater, p<0.005) or Hes7 heterozygotes (9-fold greater, p<0.001). These data indicate a synergistic, as opposed to additive, effect of loss of one Hes7 allele in the Fgf4/Hes7 compound mutant.
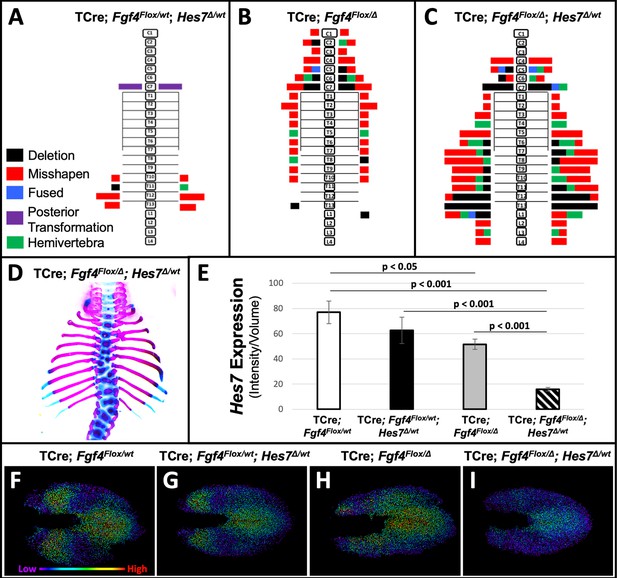
Removal of one Hes7 allele exacerbates vertebral defects in Fgf4 mutants.
(A–C) Histograms representing the vertebral column and associated ribs (C = cervical, T = thoracic, L = lumbar), showing variety and location of vertebral defects at E18.5 in the genotypes indicated (A and B, n = 7; C, n = 6). Each block in the histogram represents a single defect as indicated in the key in A. (D) Skeletal preparation of a representative E18.5 compound Fgf4 mutant- Hes7 heterozygote (ventral view). (E) Quantification of Hes7 expression within the PSM in 5–6 somite stage embryos of the indicated genotype: control (n = 10), Fgf4-Hes7 double heterozygotes (n = 6), Fgf4 mutants (n = 10), and compound Fgf4 mutant- Hes7 heterozygotes (n = 7). Quantification of Hes7 mRNA expression, determined by measurement of fluorescent intensity per cubic micrometer within the volume of the mesoderm-specific Tbx6 expression domain. Data in E are mean ± s.e.m, significance determined by a Student’s t-test. (F–I) Spot models based on HCR analysis of Hes7 expression, in embryos of the indicated genotype, within the PSM, as defined by the Tbx6 expression domain. Localized Hes7 expression is colored by level of expression from low (purple) to high expression (red), as indicated. Images F-I are dorsal views, anterior left.
We performed a similar genetic analysis where we determined if Hes7 heterozygosity likewise affects Fgf8 mutant defects. We examined littermates of the last cross described in Table 1 (Figure 7—figure supplement 1). Fgf8 mutants in this cohort displayed the same homeotic transformations we previously observed with incomplete penetrance and expressivity: small ribs in the posterior cervical vertebra (3/5, 2 bilateral and 1 unilateral) or anterior lumbar vertebra (3/5, 1 bilateral and 2 unilateral). Hes7 heterozygotes (also heterozygous for a floxed Fgf8 allele) displayed a nearly identical frequency (2.7 defects per animal) as observed in the Fgf4/Hes7 experiment (Figure 7A). Vertebral defects in compound Fgf8/Hes7 mutants were not synergistic (four defects per animal) but were clearly the Hes7 heterozygous defects added to the relatively mild Fgf8 defects (1.8 defects per animal, Figure 7—figure supplement 1). Therefore, we demonstrate that the relationship between Hes7 and Fgf4 is unique in that there is no genetic interaction between Fgf8 and Hes7.
We then examined levels and spatial patterns of Hes7 mRNA expression in each genotype from the Fgf4/Hes7 mutant littermates, specifically within a volumetric model of the Tbx6 expression domain, as we had in Fgf4 mutants (Figures 5 and 6). We observed a reduction in Hes7 expression that correlated with the severity of vertebral defects within each genotype (Figure 7E). Hes7 null heterozygotes had only a 19% reduction in expression, presumably because a loss of HES7 auto-repression results in enhanced transcription from the remaining wildtype allele. Such compensatory upregulation fails in compound Fgf4/Hes7 mutants because we observed an 80% Hes7 reduction to occur in these embryos (Figure 7E). Analysis of intensity-colored spot models of PSMs of these genotypes suggests a threshold effect of Hes7 levels on oscillatory gene expression (Figure 7F–I). Oscillations appear relatively normal if Hes7 levels are reduced 19% (in Hes7 heterozygotes, Figure 7G) but are disordered with a 33% reduction (in Fgf4 mutants, Figure 7H). The synergistic 80% reduction of Hes7 that occurs in compound Fgf4/Hes7 mutants causes a severe dampening of oscillatory gene expression (Figure 7I). The resulting vertebral defects, though relatively severe (Figure 7A–D), are not as extreme as occurs in Hes7 null homozygotes (Bessho, 2001), indicating that this reduced level of Hes7 supports limited patterning during segmentation. Together, our data support our hypothesis that FGF4 acts to maintain Hes7 above a necessary threshold for normal somitogenesis. In Fgf4 mutants, a reduction in Hes7 levels, leads to abnormal patterns of activated Notch. Abnormal Notch oscillations initiate Mesp2 unevenly in the anterior PSM leading to improperly shaped somites and subsequently, malformed vertebrae.
Discussion
We describe mutants with only Fgf4 or Fgf8 inactivated specifically in the primitive streak and PSM. In previous work, we reported that somites form in such mutants (Naiche et al., 2011; Perantoni et al., 2005); here we examine the patterning of these somites, the Notch pathway oscillations that control their formation, as well as the vertebral elements that they eventually form. We found that Fgf4 mutants display a range of cervical and thoracic vertebral defects that are caused by aberrant Notch oscillations during somitogenesis. In contrast, the vertebral columns of Fgf8 mutants are normally segmented, with about 30% displaying minor homeotic transformations; such alternations in vertebral identity are not likely due to defects in somitogenesis per se. Previously, a role for FGF signaling in wavefront activity in chick and zebrafish embryos was supported by pharmacological manipulation (Dubrulle et al., 2001; Sawada et al., 2001) and in mouse mutants where both Fgf4 and Fgf8 are simultaneously inactivated using the same TCre activity that we use in this study (Naiche et al., 2011). These double Fgf4/Fgf8 mutants as well as mutants with tissue-specific inactivation of Fgfr1 support a role for FGF signaling in clock oscillations, but these conclusions were complicated by a loss of PSM tissue due to potential wavefront defects (Oginuma et al., 2008; Naiche et al., 2011; Wahl et al., 2007; Niwa et al., 2007). Compared to these Fgf pathway mutants, the phenotype of Fgf4 mutants is relatively subtle, with no wavefront defect, as indicated by no loss of caudal vertebrae (Anderson et al., 2016a) and no quantitative change in wavefront gene markers (Figure 2). This lack of any overt axis extension defect allows us to unambiguously identify Fgf4 as an Fgf ligand gene required for a normal segmentation clock. This insight is supported by recently published work showing that FGF signaling regulates the dynamics of gene expression oscillations in PSM cells cultured in vitro (Diaz-Cuadros et al., 2020).
Our mutants model human Segmentation Defects of the Vertebrae (SDV); Fgf4 mutants resemble CS and the synergistic phenotype resulting from the additional removal of one Hes7 copy in these mutants resembles human SCDO. Both of these diseases are caused by mutations in Notch signaling components that we find are misexpressed in Fgf4 mutants, such as HES7, MESP2, and LFNG (Eckalbar et al., 2012; Giampietro et al., 2009). With regard to the human FGF pathway and SDV, a straightforward gene-disorder relationship has not been uncovered, probably because of the pleiotropic phenotypes of such putative mutations would preclude embryonic survival. Sparrow et al found that gestational hypoxia in mice results in an increase in the severity and penetrance of CS in Notch1, Mesp2, and Hes7 heterozygotes (Sparrow et al., 2012). Intriguingly, CS is more severe in children living at high altitudes (Hou et al., 2018), suggesting a similar environmental effect may affect human development. In mice, hypoxia was found to diminish FGF signaling components, but expression of Fgf8 was unchanged, and Fgf4 was unexamined (Sparrow et al., 2012). Our data suggest that FGF4 may be part of the system that is responsive to this environmental insult. However, if this is the case, reduced FGF4 activity cannot be the only response to reduced oxygen levels because gestational hypoxia reduces expression of the FGF target gene Spry4 in the PSM, whereas we found no change in expression in this or other such canonical FGF target genes in Fgf4 mutants.
Rather, as is the case for hypoxia-treated embryos (Sparrow et al., 2012), Hes7 expression is reduced in Fgf4 mutants and we propose that this leads to aberrant segmentation. An indispensable tool to this insight was the use of multiplex HCR imaging (Choi et al., 2014; Choi et al., 2018; Trivedi et al., 2018). The simultaneous fluorescent imaging of multiple mRNA domains with this technique is particularly useful in a complex embryonic process, such as somitogenesis, where many complex and dynamic gene expression patterns need to be analyzed. HCR is a relatively new tool for mutant embryo analysis, just beginning to be used to analyze mutant embryos (Lignell et al., 2017; Kim et al., 2019; Attardi et al., 2018; McKinney et al., 2016). We used HCR to examine the expression of multiple genes in a single tissue by combining it with Imaris image analysis software. This combination of HCR and Imaris-modeling is broadly applicable for in situ quantification of gene expression within any complex embryonic or clinical sample. With distinct molecular markers, one can use this approach to quantify gene expression in any cell population with a precision that heretofore was not possible and still retain the intact whole mount embryo or tissue. Here, we generated and analyzed Imaris-based volumetric models of the PSM, based on Tbx6 expression, and determined that Hes7 levels are reduced by about 40–50% specifically in the PSM of Fgf4 mutants during early somite stages when the progenitors of aberrant vertebrae are segmenting.
We propose that this reduction in Hes7 levels is the primary defect in Fgf4 mutants, and subsequently causes an irregular activated Notch (NICD) pattern, misexpression of Mesp2, and ultimately leads to vertebral defects. A reduction in Hes7 levels, due to heterozygosity or hypomorphic mutations, has been shown to cause vertebral defects (Sparrow et al., 2012; Fujimuro et al., 2015; Stauber et al., 2009). The concept that Hes7 is generally regulated by FGF signaling is supported by numerous studies (Naiche et al., 2011; Niwa et al., 2007; Harima and Kageyama, 2013). Future work will determine whether Hes7 is a direct FGF4 signaling target, possibly through putative ETS binding sites in the Hes7 promoter (González et al., 2013). Alternatively, FGF4 may indirectly regulate Hes7 transcription by affecting the activity of TBX6, NICD or MESOGENIN, all shown to regulate Hes7 expression in the PSM (González et al., 2013; Hayashi et al., 2018). If so, we speculate such regulation would be post-transcriptional because we find no significant difference in levels of the genes encoding these factors. Additionally, it is possible that FGF4 signaling is interfacing with WNT signaling, which also has been shown to regulate Hes7 Aulehla et al., 2008; Dunty et al., 2008; González et al., 2013. Given the molecular feedbacks during genetic oscillations in the PSM, we considered whether FGF4 might have a primary role on Lfng expression, whose pattern is abnormal in our mutants. However, we suggest this is not the case, because, although embryos completely lacking Lfng also display aberrant Hes7 oscillations (Niwa et al., 2007; Stauber et al., 2009), overall Hes7 mRNA levels are not reduced (Ferjentsik et al., 2009), unlike the case in our Fgf4 mutants.
We support our proposal that the observed 50% reduction in Hes7 is the cause of the Fgf4 mutant with the synergistic worsening of the vertebral segmentation defect that occurs when we further reduce Hes7 levels by removal of one wildtype allele. Notably, such synergy in defects does not occur when a copy of Hes7 is removed from Fgf8 mutants (Figure 7—figure supplement 1). From another perspective, the mild CS caused by loss of one Hes7 allele in a wildtype background (Sparrow et al., 2012) is worsened 9-fold by removing Fgf4 (Figure 7). Therefore, Fgf4 is a robustness-conferring gene that buffers somitogenesis against a perturbation in Hes7 gene dosage (Félix and Barkoulas, 2015).
We observed that in Fgf4 mutants Hes7 mRNA levels are reduced during rostral somitogenesis at E8.5 (5–6 somite stages), when defective somitogenesis occurs. At about E9.5 (24–26 somite stages), Hes7 levels are unaffected, and the Fgf4 mutant embryo is beginning to generate correctly patterned somites that will differentiate into normally patterned vertebrae. Tam, 1981 showed that between these two stages of mouse development, somites more than double in size. Such an increase in segment size can be due to a slower segmentation clock and/or faster regression of the determination front (Oates et al., 2012). Gomez et al., 2008 found that the caudal movement of the wavefront does not significantly change at these stages, suggesting a slowing clock between E8.5 and E9.5. Consistent with a slower clock, we observed at least 2 and frequently 3 bands of Hes7 expression at the Fgf4-sensitive stages (5–6 somite stages; Figures 4 and 5), but only 1 to 2 bands when Fgf4 loss has no effect on Hes7 expression (24–26 somite stages; Figure 5—figure supplement 5). In future experiments, we will test this hypothesis directly by measuring the clock rate, using recently generated fluorescent transgenic reporters of Hes7 (Yoshioka-Kobayashi et al., 2020). Thus, it appears that FGF4 activity is required to maintain Hes7 mRNA levels above a certain threshold when the segmentation clock is faster; when Notch oscillations slow, they may become FGF-independent, or other FGFs may be at play. If this is the case in all vertebrates, we might expect embryos with faster segmentation clocks, such as snakes (Gomez et al., 2008), to have a longer window of FGF4-dependence for normal somitogenesis.
This study and past work indicate that FGF4 and FGF8 have both shared and unique roles in the vertebrate embryo’s posterior growth zone. They redundantly encode wavefront activities, preventing PSM differentiation (Naiche et al., 2011; Boulet and Capecchi, 2012). In this capacity, when signaling together, they activate downstream canonical FGF target genes, such as Spry2, Spry4, and Etv4 (Naiche et al., 2011). Here, we show the role that FGF4 signals play in maintaining Hes7 mRNA levels cannot be replaced by FGF8. Future efforts will explore how the signaling machinery within the PSM discriminates between these two FGF ligands. For example, our work argues for a reassessment of FGFR function in the PSM; prior work, in which the same Cre driver used here (TCre) inactivates Fgfr1 (Wahl et al., 2007), thought to be the only FGF receptor gene expressed in the PSM, does not exactly phenocopy the defects due to TCre-mediated loss of either Fgf4 (this study) or Fgf4/Fgf8 (Naiche et al., 2011).
Our insights regarding the roles of FGF4 and FGF8 in the caudal embryonic axis are intriguing in the context of the evolution of the vertebrate body plan. Vertebrates, together with Cephalochordates and Urochordates make up the phylum Chordata (Putnam et al., 2008). Somitogenesis predates vertebrate evolution as it occurs in Cephalochordata, the most basal living chordates (Holland and Onai, 2012). In both Cephalochordates and Urochordates, the FGF essential for embryonic axis extension is an Fgf8 ortholog (Bertrand et al., 2015; Pasini et al., 2012), suggesting that this Fgf (‘Fgf8/17/18’) may be the ancestral gene in this process. However, Fgf8/17/18 activity in these invertebrate chordates does not control gene oscillations as these embryos apparently lack a segmentation clock (Bertrand et al., 2015; Onai et al., 2015). Therefore, we speculate that the recruitment of an Fgf4 role during the evolution of vertebrate axis extension may have coincided with the development of gene oscillations that control somitogenesis.
Materials and methods
Reagent type (species) or resource | Designation | Source or reference | Identifiers | Additional information |
---|---|---|---|---|
Strain, strain background Mus musculus | Fgf4Flox/delta | PMID:10802662 | MGI:95518 | |
Strain, strain background Mus musculus | Fgf8flox/delta | PMID:9462741 | MGI:99604 | |
Strain, strain background Mus musculus | Hes7delta | PMID:11641270 | MGI:2135679 | |
Strain, strain background Mus musculus | TCre | PMID:16049111 | MGI:3605847 | |
Chemical compound, drug | Alcian Blue 8GX | Sigma | A5268-25G | |
Chemical compound, drug | Alizarin Red | Sigma | A-5533 | |
Chemical compound, drug | BM Purple | Roche | 11 442 074 001 | |
Chemical compound, drug | Dapi | Life Technologies | D21490 | |
Chemical compound, drug | Iohexol (Nycodenz) | Accurate Chemical and Scientific Corp | AN1002424 | |
Chemical compound, drug | Ultra-low gelling temperature agarose | Sigma | A5030 | |
Chemical compound, drug | Hydrogen peroxide | Sigma | 516813–500 ML | |
Chemical compound, drug | Ammonium Chloride | Mallinckrodt | 3384 | |
Chemical compound, drug | N-Methylacetamide | Sigma | AN1002424 | |
Chemical compound, drug | Triton-X-100 | Sigma | T8787 | |
Chemical compound, drug | fluorescein | Sigma | 46960 | |
Chemical compound, drug | acid blue 9 | TCI | CI42090 | |
Chemical compound, drug | rose bengal | Sigma | 198250 | |
Commercial assay or kit | V3.0 HCR Kit | Molecular Instruments | Wholemount Mouse Embryo | |
Software, algorithm | Imaris Software | Bitplane Inc | Imaris V9.2.1 | |
Antibody | Anti-NICD (rabbit polyclonal) | CST | #4147 | (1:100) |
Antibody | Anti-rabbit alexa-647 (goat polyclonal) | Thermo Fisher Scientific | A32733 | (1:100) |
Antibody | Anti-Dig (sheep FAB fragments) | Roche | 11 093 274 910 | (1:4000) |
Other | Fetal calf serum | Thermo Fisher Scientific | 16140071 |
Alleles, Breeding and Genotyping
Request a detailed protocolAll mice were kept on a mixed background. All genetic crosses are shown in Table 1, with the female genotype shown first and the male genotype shown second. PCR-genotyping for each allele was performed using the following primer combinations, Fgf4flox (Sun et al., 2000) (5’- CAGACTGAGGCTGGACTTGAGG and 5’- CCTCTTGGGATCTCGATGCTGG), Fgf4Δ (Sun et al., 2000) (5’- CTCAGGAACTCTGAGGTAGATGGGG and 5’-ATCGGATTCCACCTGCAGGTGC), Fgf8flox (Meyers et al., 1998) (5’-GGTCTTTCTTAGGGCTATCCAAC and 5’-GCTCACCTTGGCAATTAGCTTC) Fgf8Δ (Meyers et al., 1998) (5’-CCAGAGGTGGAGTCTCAGGTCC and 5’-GCACAACTAGAAGGCAGCTCCC), Hes7Δ (Bessho, 2001) (5’-AGAAAGGGCAGGGAGAAGTGGGCGAGCCAC, and 5’-TTGGCTGCAGCCCGGGGGATCCACTAGTTC), TCre (Perantoni et al., 2005) (5’- GGGACCCATTTTTCTCTTCC, and 5’-CCATGAGTGAACGAACCTGG).
HCR
View detailed protocolHCR fluorescent in situ analysis where carried out as described (Choi et al., 2018) with the modification of using 60 pmol of each hairpin per 0.5 mL of amplification buffer. Hairpins where left 12–14 hr at room temperature for saturation of amplification to achieve highest levels of signal to noise (Trivedi et al., 2018). Stained embryos were soaked in DAPI solution (0.5 µg/mL DAPI in 5x SSC with 0.1% TritonX-100, 1% Tween20) overnight at room temperature. Split initiator probes (V3.0) were designed by Molecular Instruments, Inc.
Whole mount immunohistochemistry, colorimetric WISH, and skeletal staining
View detailed protocolNotch Intracellular Domain staining (NICD) was performed following a significantly modified protocol (Geffers et al., 2007); briefly, embryos were dissected in cold PBS, briefly fixed for 5 min in 4% PFA, then fixed for 35 min in equal parts DMSO:Methanol:30% Hydrogen Peroxide at room temperature. They were then rinsed 3 × 5 min in 50 mM Ammonium Chloride at room temperature, blocked in TS-PBS (PBS, 1% Triton-X-100, 10% Fetal Calf Serum) for 30 min at room temperature, then incubated overnight in 1:100 anti-NICD (CST #4147) in TS-PBS at 4° C rocking. The next day, embryos were washed 4 × 10 min in TS-PBS, then incubated overnight in anti-rabbit-alexa647 (ThermoFisher A32733) 1:100 in TS-PBS at 4°C rocking. Embryos were then washed 4 × 10 min in TS-PBS then soaked overnight in DAPI, as described above in the HCR section, and embedded and cleared as described below. WISH and skeletal staining were performed as previously described (Anderson et al., 2016b).
Embedding and clearing
Embedding
Request a detailed protocolStained embryos where mounted in coverslip bottomed dishes suspended in ultra-low gelling temperature agarose (Sigma, A5030) that had been cooled to room temperature. Once correct positioning of embryos was achieved the dishes were moved to ice to complete gelling.
Clearing
View detailed protocolFor tissue clearing we utilized Ce3D+, a modified version of Ce3D (Li et al., 2017), in which the concentration of iohexol (Nycodenz, AN1002424, Accurate Chemical and Scientific Corp) is increased to match the refractive index (RI) of the mounting solution to that of standard microscopy oils (nD = 1.515; not utilized in this study) and 1-thioglycerol is omitted to reduce toxic compounds within the solution (thereby making the solution safer to handle) and increase shelf life. To account for dilution by water from a sample (including agarose volume) we used Ce3D++ solution, in which the iohexol concentration is further increased. Ce3D++ was designed to produce desired RI after two incubations - detailed protocol is available upon request. The protocol for preparing these solutions is as follows: in a 50 mL tube, add iohexol powder (20 g for Ce3D+ or 20.83 g for Ce3D++), then 10.5 g of 40% v/v solution of N-Methylacetamide (M26305, Sigma) in 1X PBS, and then 22.5 mg Triton-X-100 (T8787, Sigma) for a final concentration of 0.1%. The solution is mixed overnight on an orbital rocker then stored at room temperature. Embedded embryos were cleared using 2 changes of Ce3D++ solution while rocking at room temperature for a twenty-four-hour period.
Imaging
Request a detailed protocolAll images were obtained on an Olympus FV1000 confocal microscope, with an image size of 1620 × 1200 pixels and with a Kalman averaging of 3 frames. To capture the entire tissue a 10x UPlanApo objective (NA = 0.4) was used achieving a pixel size of 0.9 x 0.9 µm. Tissues were oriented in the same way with the anterior-posterior axis of the tissue oriented from left-right within the center of the field. Microscope settings were kept consistent between imaging. Intensity calibration and shading correction was performed as previously described (Model, 2006), however fluorophore dye concentrations used for shading correction were 0.05 mg/L for fluorescein (Sigma 46960), 1 mg/L for acid blue 9 (TCI CI42090), and 2 mg/L for rose bengal (Sigma 198250). Dyes were placed in coverslip bottomed dishes. The Shading Correction plugin within Fiji was then used with the median flat field images acquired using the dye solutions.
Image processing
Request a detailed protocolImages within figures were processed using Fiji (Schindelin et al., 2012) and represent max projections of z-stacks. Compared images are presented with identical intensity ranges for each channel. Orthogonal projections were made using Imaris software (Imaris V9.2.1, Bitplane Inc).
Statistical analysis
Request a detailed protocolFor all analysis at least three embryos were used unless otherwise stated in the text or caption. Significance was determined using a Students two-tailed t-test.
Imaris fluorescence quantification and modeling
HCR data
View detailed protocolFlat-fielded image stacks were imported from Fiji into Imaris (Imaris V9.2.1, Bitplane Inc). A baseline subtraction was then performed for each probe, using a cutoff value specific for each probe and fluorophore combination. For all probes, except Hes7, the baseline cutoff was adjusted until signal from a tissue known not to express the gene was no longer detectable (e.g. neural epithelium for Tbx6; Nowotschin et al., 2012; Chapman et al., 1996). For Hes7, this cutoff was determined by using embryos that were homozygous for a Hes7 null allele (Bessho, 2001) that lacked sequences complementary to the HCR probes; the cutoff was chosen that resulted in no signal in these mutants (Figure 5—figure supplement 5).
The Surface model tool was used to build surfaces for each expression domain to be quantified except for Fgf8 and Hes7, which were quantified using a surface derived from the Tbx6 expression domain. Quantification of Spry2, Spry4 and Etv4 were determined by measurement of fluorescence intensity per cubic micrometer within the volume the respective gene expression domain. Surface models were generated using a surface detail value of 3 µM, absolute intensity setting, and an absolute intensity threshold cutoff that was set to exclude background signal in tissues known not to express the gene being modeled. Once established for each probe, the same intensity threshold cutoff values were used for generating surfaces for all embryos. For generating Tbx6 surfaces, a range of absolute intensity threshold cutoffs were used to achieve a final surface volume between 9.0e6 and 1.1e7 µm3. Values for mRNA expression represent the intensity sum within the volumetric model divided by the volume of the model.
Immunostaining data
Request a detailed protocolIn images where NICD was immunostained, the surface creation tool was used. The mesoderm was selected, eliminating the ectodermal and endodermal tissue, on alternating z-planes through the entirety of the z-stack. The resulting pattern was interpolated for intervening planes. Values for NICD expression represent the intensity sum within the volumetric model divided by the volume of the model.
Spot modeling
Request a detailed protocolSpot diameter was set to 4 µM with a point spread function value of 8 µM, local background subtraction was used, and sum intensity of the channel being modeled was used to threshold. Threshold cutoff values for spot models were set at 1500 for all figures except Figure 6. In Figure 6 the thresholds values (a.u.) for the sum intensity are: low (blue), 1,000–6,000; low-medium (green), 6,000–11,000; medium (yellow), 11,000–16,000; medium-high (red), 16,000–21,000; high (white), 21,000 or greater. ‘Heat maps’ in Figures 4 and 7 were generated within the Imaris software using a linear color scale; the scale in Figure 4 ranged from intensity values of 7000 (blue) to 20,000 (red), the scale in Figure 7 ranged from 1000 (blue) to 15,000 (red).
Data availability
All data generated or analyzed during this study are included in the manuscript and supporting files.
References
-
A β-catenin gradient links the clock and wavefront systems in mouse embryo segmentationNature Cell Biology 10:186–193.https://doi.org/10.1038/ncb1679
-
Segment formation in Annelids: patterns, processes and evolutionThe International Journal of Developmental Biology 58:469–483.https://doi.org/10.1387/ijdb.140148gb
-
Dynamic expression and essential functions of Hes7 in somite segmentationGenes & Development 15:2642–2647.https://doi.org/10.1101/gad.930601
-
Signaling by FGF4 and FGF8 is required for axial elongation of the mouse embryoDevelopmental Biology 371:235–245.https://doi.org/10.1016/j.ydbio.2012.08.017
-
Tbx6, a mouse T-Box gene implicated in paraxial mesoderm formation at GastrulationDevelopmental Biology 180:534–542.https://doi.org/10.1006/dbio.1996.0326
-
A clock and wavefront model for control of the number of repeated structures during animal morphogenesisJournal of Theoretical Biology 58:455–476.https://doi.org/10.1016/S0022-5193(76)80131-2
-
Scoliosis and segmentation defects of the vertebraeWiley Interdisciplinary Reviews: Developmental Biology 1:401–423.https://doi.org/10.1002/wdev.34
-
Pervasive robustness in biological systemsNature Reviews Genetics 16:483–496.https://doi.org/10.1038/nrg3949
-
Hes7 3′UTR is required for somite segmentation functionScientific Reports 4:6462.https://doi.org/10.1038/srep06462
-
Divergent functions and distinct localization of the notch ligands DLL1 and DLL3 in vivoJournal of Cell Biology 178:465–476.https://doi.org/10.1083/jcb.200702009
-
Progress in the understanding of the genetic etiology of vertebral segmentation disorders in humansAnnals of the New York Academy of Sciences 1151:38–67.https://doi.org/10.1111/j.1749-6632.2008.03452.x
-
Oscillatory links of fgf signaling and Hes7 in the segmentation clockCurrent Opinion in Genetics & Development 23:484–490.https://doi.org/10.1016/j.gde.2013.02.005
-
Presomitic mesoderm-specific expression of the transcriptional repressor Hes7 is controlled by E-box, T-box, and notch signaling pathwaysJournal of Biological Chemistry 293:12167–12176.https://doi.org/10.1074/jbc.RA118.003728
-
Early development of cephalochordates (amphioxus)Wiley Interdisciplinary Reviews: Developmental Biology 1:167–183.https://doi.org/10.1002/wdev.11
-
Abnormalities associated with congenital scoliosis in high-altitude geographic regionsInternational Orthopaedics 42:575–581.https://doi.org/10.1007/s00264-018-3805-2
-
Signalling dynamics in vertebrate segmentationNature Reviews Molecular Cell Biology 15:709–721.https://doi.org/10.1038/nrm3891
-
Oscillatory gene expression and somitogenesisWiley Interdisciplinary Reviews: Developmental Biology 1:629–641.https://doi.org/10.1002/wdev.46
-
Evolutionary plasticity of segmentation clock networksDevelopment 138:2783–2792.https://doi.org/10.1242/dev.063834
-
Intensity calibration and shading correction for fluorescence microscopesCurrent Protocols in Cytometry 14:cy1014s37.https://doi.org/10.1002/0471142956.cy1014s37
-
On the origin of vertebrate somitesZoological Letters 1:33.https://doi.org/10.1186/s40851-015-0033-0
-
Notch ligands are substrates for protein O-fucosyltransferase-1 and fringeThe Journal of Biological Chemistry 277:29945–29952.https://doi.org/10.1074/jbc.M204445200
-
The segmentation clock: inherited trait or universal design principle?Current Opinion in Genetics & Development 22:600–606.https://doi.org/10.1016/j.gde.2012.10.003
-
Fgf/MAPK signalling is a crucial positional cue in somite boundary formationDevelopment 128:4873–4880.
-
Fiji: an open-source platform for biological-image analysisNature Methods 9:676–682.https://doi.org/10.1038/nmeth.2019
-
Autosomal dominant spondylocostal dysostosis is caused by mutation in TBX6Human Molecular Genetics 22:1625–1631.https://doi.org/10.1093/hmg/ddt012
-
Mesp2 initiates somite segmentation through the notch signalling pathwayNature Genetics 25:390–396.https://doi.org/10.1038/78062
-
Screening of known disease genes in congenital scoliosisMolecular Genetics & Genomic Medicine 6:966–974.https://doi.org/10.1002/mgg3.466
-
The control of somitogenesis in mouse embryosJournal of Embryology and Experimental Morphology 65 Suppl:103–128.
-
Of mice and plants: comparative developmental systems biologyDevelopmental Biology 460:32–39.https://doi.org/10.1016/j.ydbio.2018.10.024
Article and author information
Author details
Funding
National Cancer Institute (ZIA BC010338-19)
- Mark Lewandoski
The funders had no role in study design, data collection and interpretation, or the decision to submit the work for publication.
Acknowledgements
We thank Hector Escriva for helpful discussion. We also thank E Kamiya and W Heinz of the NCI Optical Microscopy and Image Analysis Lab, for their assistance with clearing using Ce3D+ and Imaris, respectively. We are grateful for technical assistance from C Bonatto, T Kuruppu, C Elder, E Truffer, and M Boylan. We thank M Kaltcheva, P Abete-Luzi and A Perantoni for critical reading of the manuscript.
Ethics
All work with mice was approved and monitored by the NCI-Frederick Animal Care and Use Committee (ACUC). Mice were grouply housed in AAALAC (Association for Assessment and Accreditation of Laboratory Animal Care International) accredited facilities and in accordance with ACUC guidelines (Animal Study Protocol 17–069).
Copyright
This is an open-access article, free of all copyright, and may be freely reproduced, distributed, transmitted, modified, built upon, or otherwise used by anyone for any lawful purpose. The work is made available under the Creative Commons CC0 public domain dedication.
Metrics
-
- 3,687
- views
-
- 441
- downloads
-
- 48
- citations
Views, downloads and citations are aggregated across all versions of this paper published by eLife.
Citations by DOI
-
- 48
- citations for umbrella DOI https://doi.org/10.7554/eLife.55608