Centromere deletion in Cryptococcus deuterogattii leads to neocentromere formation and chromosome fusions
Abstract
The human fungal pathogen Cryptococcus deuterogattii is RNAi-deficient and lacks active transposons in its genome. C. deuterogattii has regional centromeres that contain only transposon relics. To investigate the impact of centromere loss on the C. deuterogattii genome, either centromere 9 or 10 was deleted. Deletion of either centromere resulted in neocentromere formation and interestingly, the genes covered by these neocentromeres maintained wild-type expression levels. In contrast to cen9∆ mutants, cen10∆ mutant strains exhibited growth defects and were aneuploid for chromosome 10. At an elevated growth temperature (37°C), the cen10∆ chromosome was found to have undergone fusion with another native chromosome in some isolates and this fusion restored wild-type growth. Following chromosomal fusion, the neocentromere was inactivated, and the native centromere of the fused chromosome served as the active centromere. The neocentromere formation and chromosomal fusion events observed in this study in C. deuterogattii may be similar to events that triggered genomic changes within the Cryptococcus/Kwoniella species complex and may contribute to speciation throughout the eukaryotic domain.
Introduction
Eukaryotic organisms have linear chromosomes with specialized regions: telomeres that cap the ends, origins of replication, and centromeres that are critical for chromosome segregation. During cell division, the centromere binds to a specialized protein complex known as the kinetochore (Cheeseman, 2014). Most centromeres are regional, sequence-independent, and defined by the replacement of the canonical histone H3 by the histone homolog CENP-A (CenH3 or Cse4) (Henikoff and Furuyama, 2010). In humans, centromeres contain higher-order α-satellite DNA arrays that span 0.1 to 4.8 Mb (McNulty and Sullivan, 2018), which is in contrast to most fungal centromeres, which contain transposable elements and repetitive sequences (Friedman and Freitag, 2017). Fungal regional centromeres range from the small centromeres of Candida albicans, (the CENP-A enriched regions range from 2 to 3.3 kb and are located in 4 to 18 kb open-reading frame ORF-free regions), to the large regional centromeres described in Neurospora crassa, (which range from 174 to 287 kb and consist mainly of truncated transposable elements) (Sanyal et al., 2004; Smith et al., 2011). Similar to mice, some fungi have pericentric regions (Guenatri et al., 2004). The most prominent examples are the centromeres of Schizosaccharomyces pombe, which have a CENP-A-enriched region comprised of a central core flanked by heterochromatic pericentric regions divided into outer and inner repeats (Ishii et al., 2008; Rhind et al., 2011). Budding yeast have sequence-dependent centromeres, which are short and have a conserved organization with three centromere DNA elements (consensus DNA elements (CDEs) I-III) (Kobayashi et al., 2015). However, the budding yeast Naumovozyma castellii has unique consensus DNA elements that differ from those of other budding yeast species (Kobayashi et al., 2015).
Infrequently, centromeres can be spontaneously inactivated, resulting in neocentromere formation (i.e., evolutionary new centromeres) (Ventura et al., 2007). Neocentromere formation can occur either while the native centromeric sequence is still present on the chromosome or when the native centromere has been mutated or deleted (e.g., from chromosomal rearrangements or γ irradiation damage Burrack and Berman, 2012; Tolomeo et al., 2017; Ventura et al., 2007). In addition, several studies have described neocentromere formation after deletion of native centromeres by molecular genetic engineering in fungi, chickens, and Drosophila (Alkan et al., 2007; Ishii et al., 2008; Ketel et al., 2009; Shang et al., 2013). In some organisms, the formation of neocentromeres can be deleterious, leading to disease, cancer, or infertility (Burrack and Berman, 2012; Garsed et al., 2014; Nergadze et al., 2018; Scott and Sullivan, 2014; Warburton, 2004). For example, human neocentromeres are often identified in liposarcomas (Garsed et al., 2014). However, neocentromere formation also can be beneficial, leading to speciation (Ventura et al., 2007).
Fungal neocentromeres are well described in the diploid yeast C. albicans and the haploid fission yeast S. pombe (Ishii et al., 2008; Ketel et al., 2009; Thakur and Sanyal, 2013). Deletion of C. albicans native centromere 5 or 7 has been shown to induce neocentromere formation and does not result in chromosome loss (Ketel et al., 2009; Thakur and Sanyal, 2013). In these cases, neocentromeres conferred chromosomal stability similar to the native centromere (Ketel et al., 2009; Mishra et al., 2007). Deletion of a native centromere in S. pombe led to either neocentromere formation or chromosome fusion (Ishii et al., 2008; Ohno et al., 2016). S. pombe neocentromeres formed in telomere-proximal regions near heterochromatin, and neocentromere organization featured a CENP-A-enriched core domain and heterochromatin at the subtelomeric (distal) side. Interestingly, neocentromere formation occurred at the same regions in both wild-type and heterochromatin-deficient strains, suggesting that heterochromatin is dispensable for neocentromere formation in S. pombe, although the rate of survival by chromosome fusion was significantly increased in heterochromatin-deficient mutants (Ishii et al., 2008). Deletion of kinetochore proteins (mhf1∆ and mhf2∆) led to a shift of CENP-A binding, resulting in a CENP-A-enriched region directly adjacent to the native centromere (Lu and He, 2019).
In some cases, neocentromeres span genes that are silenced, such as the neocentromeres in C. albicans. However the mechanisms that mediate silencing of neocentromeric genes are unknown in C. albicans, as proteins that are necessary for heterochromatin formation and gene silencing in other species (HP1, Clr4, and DNA methyltransferase) are absent in C. albicans (Ketel et al., 2009). Neocentromeres of S. pombe can also span genes. These genes are upregulated during nitrogen starvation and expressed at low levels during stationary growth in wild-type cells, but are silenced under all conditions tested when spanned by neocentromeres. In addition to neocentromeric genes, genes located within native centromeres have been identified in other fungi as well as rice and chicken (Nagaki et al., 2004; Schotanus et al., 2015; Shang et al., 2013).
Recently, the centromeres of the human pathogenic fungus Cryptococcus deuterogattii were characterized and compared to those of the closely related species Cryptococcus neoformans (centromeres ranging from 27 to 64 kb), revealing dramatically smaller centromeres in C. deuterogattii (ranging from 8.7 to 21 kb) (Janbon et al., 2014; Yadav et al., 2018). C. deuterogattii is responsible for an ongoing cryptococcosis outbreak in the Pacific Northwest regions of Canada and the United States (Fraser et al., 2005). In contrast to the sister species C. neoformans, C. deuterogattii commonly infects immunocompetent patients (Fraser et al., 2005). C. deuterogattii is a haploid basidiomycetous fungus with 14 chromosomes (D'Souza et al., 2011; Farrer et al., 2015; Yadav et al., 2018). The dramatic reduction in centromere size in C. deuterogattii may be attributable to loss of the RNAi pathway (Farrer et al., 2015; Yadav et al., 2018). The centromeres of C. deuterogattii consist of truncated transposable elements, and active transposable elements are missing throughout the genome (Yadav et al., 2018). This is in stark contrast to C. neoformans, which has active transposable elements in centromeric regions (Dumesic et al., 2015; Janbon et al., 2014; Yadav et al., 2018).
Neocentromeres are frequently formed near genomic repeats, yet C. deuterogattii lacks active transposons that might seed neocentromere formation. Thus, C. deuterogattii is a unique organism in which to study centromere structure and function. To elucidate centromeric organization, the native centromeres of chromosomes 10 and 9 were deleted, leading to characterization of the first neocentromeres in the Basidiomycota phylum of the fungal kingdom.
Results
Deletion of centromere 9 and 10 results in neocentromere formation
To determine if neocentromere formation occurs in the C. deuterogattii reference strain R265, either centromere 9 or 10 was deleted. Centromere 9 (CEN9) was deleted by CRISPR-Cas9-mediated transformation. Two guide RNAs flanking the centromere were used and CEN9 was replaced with a NAT dominant drug-resistance gene by homologous recombination. Biolistic transformation was used to replace centromere 10 (CEN10) with either the NAT or NEO dominant drug-resistance gene via homologous recombination. Viable transformants with the correct integration and deletion were obtained and confirmed by 5’ junction, 3’ junction, loss of deleted regions, and spanning PCRs as well as Southern blot analysis for cen10∆ (Figure 1—figure supplement 1, Figure 1—figure supplement 2). Multiple independent cen9∆ and cen10∆ deletion mutants (cen9∆-A to -F and cen10∆-A to -G) were obtained from independent transformations. Pulsed-field gel electrophoresis (PFGE) confirmed that cenΔ mutants had a wild-type karyotype and that chromosome 9 and 10 remained linear, because a circular chromosome would not have entered the gel (Figure 1—figure supplement 3).
The formation of neocentromeres on chromosome 10 in C. deuterogattii was infrequent. A total of 99 independent biolistic transformations resulted in only seven confirmed cen10∆ mutants (7/21 total candidate transformants, 33% homologous integration), suggesting that CEN10 deletion is lethal in most circumstances. In comparison, deletion of nonessential genes by homologous recombination in the C. deuterogattii R265 strain typically results in ~100 colonies with a high success rate (~80–90% homologous integration). We estimate that the likelihood of deleting a centromere and recovering a viable colony is at least 1000-fold lower than would be expected from the deletion of a non-essential gene. The deletion of CEN9 was more efficient as this was mediated by CRISPR-Cas9 cleavage with two guide RNAs and a repair allele.
Chromatin immunoprecipitation of mCherry-CENP-A followed by high-throughput sequencing (ChIP-seq) for six cen9∆ (-A to -F) and seven cen10∆ mutants (-A to -G) was performed (Figure 1). Prior to the ChIP-seq experiment, all of the centromere deletion mutants were streak purified from single colonies. The sequence reads were mapped to a complete whole-genome assembly, followed by the normalization of the reads by subtraction of the input from the ChIPed sample (Yadav et al., 2018). To quantify the ChIP-seq data, the CENP-A-enriched regions were compared with the centromeres previously identified based on CENP-C enrichment. Both the CENP-A- and CENP-C-enriched peaks were congruent for all of the native centromeres (Yadav et al., 2018). This analysis identified 13 of the 14 native centromeres (CEN1-8, CEN11-14 and depending on the centromere mutant either CEN9 or CEN10), indicating that, as expected, the native centromere of chromosome 9 or 10 was missing in all of the cen9∆ and cen10∆ deletion mutants respectively (Figure 1). Instead, neocentromeres were observed.
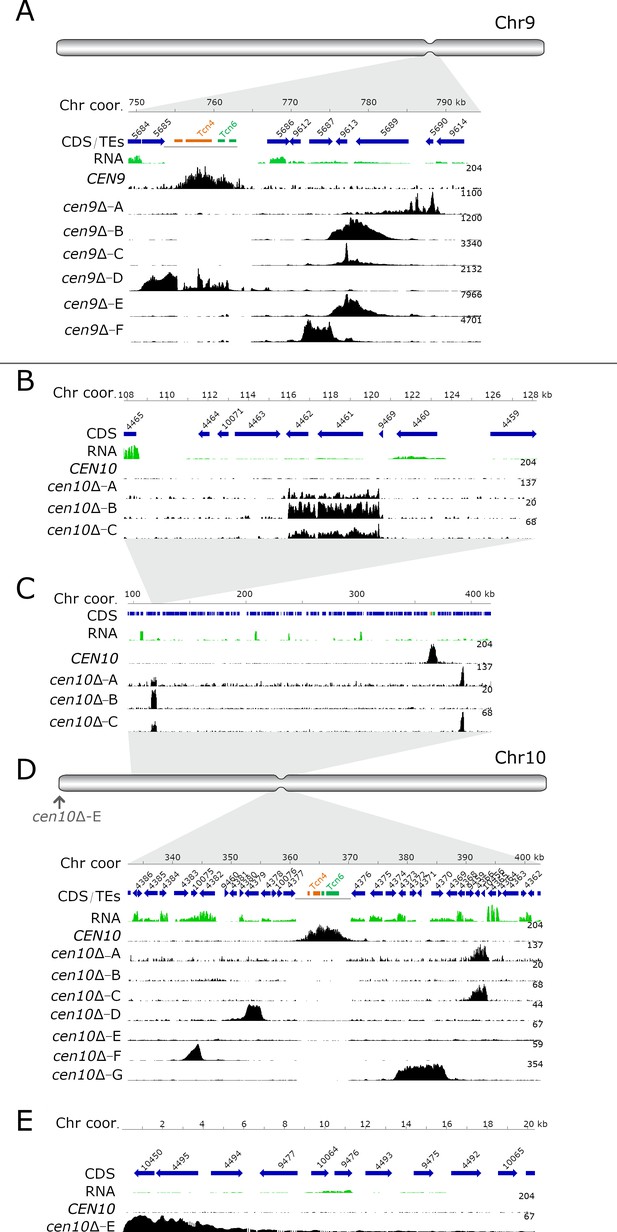
Centromere deletion leads to neocentromere formation.
For each panel, the chromosome coordinates are indicated. Genes (CDS) are shown in blue arrows and the truncated transposable elements, located in the native centromere (CEN9 or CEN10), are colored according to their class (Tcn4 in orange and Tcn6 in green). Previously generated RNA-sequencing obtained from wild-type cells was re-mapped and shown in green. In each panel, the wild-type CENP-A content is shown. In the wild type, CENP-A is only enriched at the native centromeres. For each cenΔ mutant, the neocentromeric region is shown by enrichment of CENP-A and the fold enrichment is indicated on the right of each ChIP-seq track. (A) Schematic full overview of chromosome 9, the indentation represents the native centromere 9 position. The light grey area points to the zoomed-in chromosomal region shown with the detailed view of the native centromere (CEN9) and the location of the cen9∆ mutant neocentromeres. Neocentromeres of cen9∆-B, cen9∆-C and cen9∆-E formed at the same chromosomal location. The dark gray line, below the transposable elements, indicates the deleted region in the cen9∆ mutants (B) Detailed view of the neocentromere of cen10∆-B and the secondary CENP-A peak of cen10∆-A and cen10∆-C. (C) Overview of the chromosomal 10 region spanning 100 to 410 kb. cen10∆-A and cen10∆-C have two regions enriched with CENP-A (primary and secondary). (D) Schematic full overview of the full chromosome 10, the indentation represents the chromosomal location of the native centromere (CEN10). The light grey areas point to the zoomed-in chromosomal regions shown in panel C and below. The neocentromere of cen10∆-E is indicated with an arrow. Lower panel, detailed view of the native centromere (CEN10) and the neocentromeres formed in cen10∆-A, cen10∆-C, cen10∆-D, cen10∆-F and cen10∆-G mutants. The dark gray line, below the transposable elements, indicates the deleted region in the cen10∆ mutants (E) Detailed view of the telocentric neocentromere of cen10∆-E.
Except for the neocentromere of isolate cen10∆-E, the neocentromeres formed in close proximity to the native centromere (CEN9 and CEN10). Almost all neocentromeres were shorter than the native centromere, with the exception of cen10∆-G which was larger than native centromere 10 (Table 1).
Genes located inside neocentromeres.
The chromosomal locations, sizes, and GC content (%) for the native centromere and cen∆ mutants are shown. For the neocentromeres, gene ID, predicted function, and the amount of CENP-A coverage are indicated.
Chr coor (bp) | Size (kb) | Size compared to native centromere (%) | GC% | Genes spanned by neocentromere | Gene ID | % covered by Neocentromere | Exons inside neocentromere | |
---|---|---|---|---|---|---|---|---|
Native centromere 9 | Chr9:755,771–762,621 | 6.84 | - | 43.6 | - | - | - | - |
cen9∆-A | Chr9:785,352–789,247 | 3.87 | 56.6 | 46.1 | Escrt-II complex subunit (VPS25) | CNBG_5690 | 100 | |
Iron regulator 1 | CNBG_9614 | 14.6 | Last exon | |||||
cen9∆-B | Chr9:775,164–780,756 | 4.41 | 64.5 | 46.6 | Xylosylphosphotransferase (XPT1) | CNBG_5687 | 6.9 | |
Transglycosylase SLT domain-containing protein | CNBG_9613 | 100 | ||||||
Glutamate synthase (NADPH/NADH) | CNBG_5689 | 33.7 | ||||||
cen9∆-C | Chr9:775,164–780,756 | 4.41 | 64.5 | 46.6 | Xylosylphosphotransferase (XPT1) | CNBG_5687 | 6.9 | |
Transglycosylase SLT domain-containing protein | CNBG_9613 | 100 | ||||||
Glutamate synthase (NADPH/NADH) | CNBG_5689 | 33.7 | ||||||
cen9∆-D | Chr9:750,902–755,294 | 4.37 | 63.9 | 41.9 | Hypothetical protein | CNBG_5684 | 92.8 | |
Derlin-2/3 | CNBG_5685 | 100 | ||||||
cen9∆-E | Chr9:775,164–780,756 | 5.56 | 81.3 | 50 | Xylosylphosphotransferase (XPT1) | CNBG_5687 | 6.9 | |
Transglycosylase SLT domain-containing protein | CNBG_9613 | 100 | Last exon | |||||
Glutamate synthase (NADPH/NADH) | CNBG_5689 | 33.7 | Last exon | |||||
cen9∆-F | Chr9:771,614–775,469 | 3.83 | 56.0 | 51.5 | Xylosylphosphotransferase (XPT1) | CNBG_5687 | 100 | |
Native centromere 10 | Chr10:362,876–369,657 | 6.77 | - | 42.6 | - | - | - | - |
cen10∆-A | Chr10:115,954–120,422 | 4.46 | 65.9 | 46.9 | CENPC/MIF2 | CNBG_4461 | 88.3 | 1, 2, 3, 4 (only5th is outside) |
Hypothetical protein | CNBG_4462 | 100 | ||||||
Chr10:391,090–393,946 | 2.85 | 42.1 | 48.9 | Serine/threonine-protein phosphatase 2A activator 2(RRD2) | CNBG_9459 | 10.6 | Last exon (5th) | |
Hypothetical protein | CNBG_4366 | 100 | ||||||
Hypothetical protein | CNBG_4365 | 23.4 | Last exon (3th) | |||||
cen10∆-B | Chr10:115,954–120,422 | 4.46 | 65.9 | 46.9 | CENPC/MIF2 | CNBG_4461 | 88.3 | 1, 2, 3, 4 (only 5th is outside) |
Hypothetical protein | CNBG_4462 | 100 | ||||||
cen10∆-C | Chr10:115,954–120,422 | 4.46 | 65.9 | 46.9 | CENPC/MIF2 | CNBG_4461 | 88.3 | 1, 2, 3, 4 (only 5th is outside) |
Hypothetical protein | CNBG_4462 | 100 | ||||||
Chr10:391,090–393,946 | 2.85 | 42.1 | 48.9 | Serine/threonine-protein phosphatase 2A activator 2(RRD2) | CNBG_9459 | 10.6 | Last exon (5th) | |
Hypothetical protein | CNBG_4366 | 100 | ||||||
Hypothetical protein | CNBG_4365 | 23.4 | Last exon (3th) | |||||
cen10∆-D | Chr10:352,648–355,154 | 2.51 | 37.1 | 48 | Ser/Thr protein kinase | CNBG_4379 | 88.4 | |
cen10∆-E | Chr10:1–4,385 | 4.38 | 64.7 | 53.2 | Hypothetical protein | CNBG_10450 | 100 | |
Hypothetical protein | CNBG_4495 | 100 | ||||||
cen10∆-F | Chr10:342,517–345,159 | 2.64 | 39.0 | 45.5 | Hypothetical protein | CNBG_4383 | 18.6 | Last two exons |
Hypothetical protein | CNBG_10075 | 100 | ||||||
Hexokinase (HXK1) | CNBG_4382 | 15.3 | Last three exons | |||||
cen10∆-G | Chr10:378,389–386,366 | 7.97 | 117.7 | 46.5 | High osmolarity signaling protein (SHO1) | CNBG_4373 | 100 | |
Hypothetical protein | CNBG_4372 | 100 | ||||||
Hypothetical protein | CNBG_4371 | 100 | ||||||
Hypothetical protein | CNBG_4370 | 100 |
In three of the independent cen9∆ mutants (cen9∆-B, -C and -E), neocentromeres formed at the same chromosomal location (Figure 1A). Interestingly, two independent cen10∆ mutants (cen10∆-A and cen10∆-C) contained two CENP-A-enriched regions on chromosome 10, with a primary peak and a smaller secondary peak with reduced levels of CENP-A (1.3- to 1.75-fold lower) compared to the primary CENP-A peak (Figure 1C). The chromosomal location of the secondary peak was similar to the neocentromere of cen10∆-B (which had only one neocentromere) (Figure 1B).
The two CENP-A-enriched regions suggest four possible models: 1) aneuploidy in which cells harbor two chromosomes, 2) a dicentric chromosome with two neocentromeres (neodicentric), 3) instability between two different neocentromere states (neocentromere switching), 4) or only one CENP-A-enriched region functions as a centromere and the second CENP-A-enriched region is not bound by the kinetochore (Figure 1).
The neocentromeres were located in unique, nonrepetitive sequences and were not flanked by repetitive regions. The GC content of neocentromeres is similar to the overall GC content of chromosome 9 and 10, whereas the native centromere has a lower GC content (Table 1). Comparing the reference genome with de novo genome assemblies of cen10∆-A, cen10∆-B, and cen10∆-E confirmed that transposable elements did not enter these genomic regions during neocentromere formation (Supplementary file 1). Instead of spanning repeats and transposable elements like the native centromeres, neocentromeres span genes.
All of the neocentromeres of chromosome 9 formed in a region within 26 kb of the chromosomal location of the native centromere 9 (Figure 1A). Interestingly, the neocentromeres of the independent cen9∆-B, cen9∆-C, and cen9∆-E mutants all formed at the same chromosomal location and had the same length (4.41 kb); these neocentromeres spanned three genes. One gene was completely covered by CENP-A and this gene encodes a transglycosylase SLT domain-containing protein. The two other genes (a gene encoding a xylosylphosphotransferase and a gene encoding glutamate synthase (NADPH/NADH)) were partially covered with CENP-A. Mutant cen9∆-A had a 3.87 kb long neocentromere located 26 kb 3’ to the native centromere and spanned two genes. The first gene was completely spanned by CENP-A and encodes an ESCRT-II complex subunit (Vps25) protein. The second gene was only partially covered and encodes an iron regulator protein. The neocentromere of cen9∆-D was located directly to the left of the native centromere and was 4.37 kb in length. This neocentromere spanned two genes, coding for a hypothetical protein (92% covered by CENP-A) and a gene encoding for Derlin-2/3 that was completely covered by CENP-A. Lastly, the neocentromere of mutant cen9∆-F was 3.83 kb in length and spanned one gene (encoding a xylosylphosphotransferase, XPT1), which was completely covered by CENP-A. This neocentromere was located 12 kb away (3’) from the native centromere 9.
Like the neocentromeres of cen9∆ mutants, the neocentromeres of cen10∆ mutants also spanned genes and interestingly, the kinetochore protein CENP-C was located inside the neocentromere of cen10∆-B and in the secondary peak of cen10∆-A and -C (Table 1, Figure 1B). The neocentromere in cen10∆-B spanned 4.46 kb, was located 242 kb away from the 3’ region of the native CEN10, and was located 115 kb from the telomere (Figure 1B). In addition to the gene encoding CENP-C, the CENP-A-enriched region spanned a hypothetical protein (Table 1). The primary CENP-A-enriched region of cen10∆-A and cen10∆-C spanned a gene encoding a serine/threonine-protein phosphatase 2A activator 2 (RRD2) and a hypothetical protein (Figure 1D). This neocentromere spanned 2.85 kb and was located closer to the native CEN10 (21 kb from the native centromere) than the neocentromere of cen10∆-B and the secondary CENP-A peak of cen10∆-A and cen10∆-C. The neocentromere of cen10∆-D was the smallest neocentromere (2.5 kb) and partially (88.4%) spanned a gene encoding a Ser/Thr protein kinase and formed 7.4 kb from the location of the native CEN10 (Figure 1D). The neocentromere of cen10∆-E spanned two hypothetical proteins, was 4.38 kb in length and was located directly adjacent to the right telomere (Figure 1E). Mutant cen10∆-F had a neocentromere of 2.64 kb, which spanned one hypothetical gene completely and two genes (hypothetical and a hexokinase (HXK1)) partially; the neocentromere formed at a chromosomal location 20 kb 5’ of the native centromere (Figure 1D). The neocentromere of cen10∆-G was the largest neocentromere with a CENP-A-enriched region of 7.97 kb, and was in fact larger than the native CEN10. This neocentromere spanned four genes, including a gene coding for a high osmolarity protein (Sho1) and three genes coding for hypothetical proteins (Figure 1D).
To test if the kinetochore was binding to the CENP-A-enriched regions of chromosomes 9 and 10, and to validate if the neocentromeres were fully functional as centromeres, two additional kinetochore proteins were epitope-tagged with GFP. cen9∆ mutants were transformed with an overlap PCR product expressing CENPC-GFP. As the neocentromeres of three cen10∆ mutants spanned the gene encoding CENP-C, all cen10∆ mutants were transformed with an overlap PCR product, expressing Mis12-GFP. In addition to the cen9∆ and cen10∆ mutants, the wild type was transformed with constructs expressing Mis12-GFP and CENP-C-GFP, and these served as controls. ChIP-qPCRs for cen9∆ mutants, cen10∆ mutants, and wild-type strains with Mis12-GFP or CENP-C-GFP were performed (Figure 1—figure supplement 4). Because Mis12 is an outer kinetochore protein, the formaldehyde cross-linking was extended to 45 min (15 min was used for CENP-A and CENP-C) for this protein. For all qPCR analyses, the native centromere 6 CEN6) was used as an internal control and for each neocentromere specific primer pairs were designed. For cen9∆ and cen10∆ mutants a similar level of Mis12 or CENP-C enrichment at the neocentromeres and (CEN6) was observed. This suggested that the CENP-A-enriched regions of chromosome 9 of the cen9∆ mutants and chromosome 10 of cen10∆ mutants identified by ChIP-seq were functional centromeres and indeed neocentromeres (Figure 1—figure supplement 4).
Previously generated RNA sequence data were remapped to the C. deuterogattii reference strain R265 and analyzed to determine if the regions where neocentromeres formed in the cenΔ mutants were transcribed in the wild type (Figure 1; Supplementary file 2; Schneider et al., 2012). In the wild-type strain, all genes spanned by neocentromeres in the cenΔ mutants were expressed (Figure 1). However, the expression levels of the neocentromeric genes were lower than their neighboring genes. For example, the expression level of the gene (CNBG_5686) flanking native centromere 9 was three times higher than the genes spanned by neocentromeres in cen9∆ mutants (Figure 1A). The same trend was observed in the cen10∆ mutants. Here, the expression level of the gene (CNBG_4365) 3’ flanking the neocentromere (primary CENP-A peak) of cen10∆-A and cen10∆-C was more than six times higher than the genes spanned by the neocentromere (Figure 1D). Also, the neocentromere of cen10∆-D is flanked by genes whose expression was two times higher than the genes spanned by the neocentromere (Figure 1D). This suggests that neocentromeres are formed in chromosomal regions with lower gene expression in C. deuterogattii. The majority of the genes (24/28) flanking native centromeres are transcribed in the direction towards the native centromere. All of the neocentromeres observed span one or more genes and most of the flanking genes are transcribed in the direction away from the neocentromere.
The expression levels of the neocentromeric genes in cen9∆ and cen10∆ mutants were assayed by qPCR (Figure 2). The neocentromeric genes of chromosome 9 were normalized to actin. To compensate for the ploidy levels of chromosome 10 in cen10∆ mutants, a housekeeping gene located on chromosome 10 was used to normalize the expression of genes spanned by neocentromeres located on chromosome 10. The expression levels of the CENP-A-associated neocentromeric genes were all found to be similar to the wild-type strain (Figure 2).
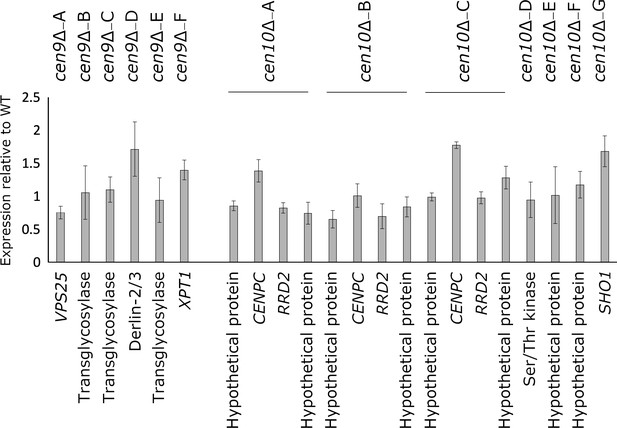
Expression of neocentromeric genes.
Expression of the neocentromeric genes was assessed by qPCR for all cenΔ mutants and expression is shown as Log2ΔΔCt. For cen10∆-A, cen10∆-B and cen10∆-C, two genes were selected from each neocentromeric region, all other cen∆ mutants are represented by one gene spanned by CENP-A. cen10∆-B has only one CENP-A-enriched region, and in this case, the genes located within primary peak of cen10∆-A and cen10∆-C served as controls. The qPCRs of cen10∆ mutants are normalized with a housekeeping gene located on chromosome 10. The qPCRs of cen9∆ mutants are normalized with actin. Error bars show standard deviation.
Neocentromere formation can reduce fitness
cen10∆ mutants were noted to grow more slowly than wild type. To investigate this, the growth of cen10∆ and wild-type strains was measured during the course of a 22-hour cell growth experiment (Figure 3A). The majority of cen10∆ mutants exhibited slower growth rates compared to the wild-type parental strain R265. Six of seven cen10∆ mutants exhibited significant fitness defects compared to the wild-type strain, with doubling times ranging from 101 to 111 min compared to 81 min for the wild type (Figure 3B). In contrast, one mutant, which has a telocentric neocentromere (cen10∆-E), grew similarly to the wild type and had a similar doubling time (84 min for the mutant vs 81 min for the wild-type strain). Compared to the wild type, cen10∆ mutants with increased doubling times produced smaller colonies during growth on non-selective media (Figure 1—figure supplement 5C).
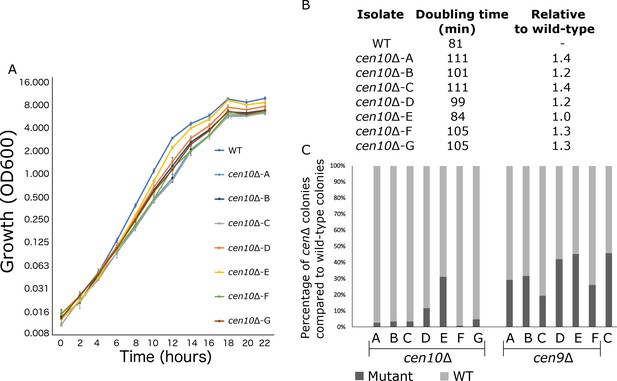
cen10∆ mutant strains have reduced fitness compared to the wild-type strain.
(A) Six out of seven cen10∆ mutants had a longer doubling time and slower growth than the wild-type strain. In contrast cen10∆-E grows similarly to the wild type. Error bars show standard deviation. (B) Doubling times and fold change compared to wild type are shown. (C) Competition assays with the wild type and cen9∆ and cen10∆ mutant strains. Mixed cultures (1:1) were grown overnight and plated with and without selection agents. After four days, colonies were counted and the percentage of cenΔ mutants (black) and wild type (grey) in each culture was plotted. As a control (C) a wild-type strain with a NAT marker was mixed with the wild type.
To compare fitness, a competition assay was performed with 1:1 mixtures of wild-type and cen9∆ or cen10∆ mutants grown in liquid YPD medium (Figure 3C). With no growth defect, the expectation was that the wild-type strain and centromere deletion mutants would grow at the same growth rate, resulting in a 1:1 ratio. In fact, fewer cen10∆ cells were found in the population after growth in competition with the wild-type strain, and this observation is consistent with the slower doubling time of cen10∆ mutants resulting in reduced fitness compared to wild type (Figure 3). Compared to the wild-type cells, there were fewer cen9∆ mutant cells in the population. However, the number was closer to a 1:1 ratio (Figure 3C). The ratio of the cen9∆ mutants in the population was similar to the ratio of the cen10∆-E mutant, which had a wild-type growth rate. Due to this observation, we hypothesize that the growth rate of the cen9∆ mutants is similar to wild type.
cen10∆ isolates are aneuploid
Because deletion of a centromere could lead to defects in chromosome segregation, cenΔ mutants were assessed for aneuploidy (Figure 4). Overall, cen10∆ mutants exhibited a mixture of large and small colony sizes during growth on YPD medium at 37°C, while cen9∆ mutants exhibited a uniform, wild-type like, colony size (Figure 1—figure supplement 5).
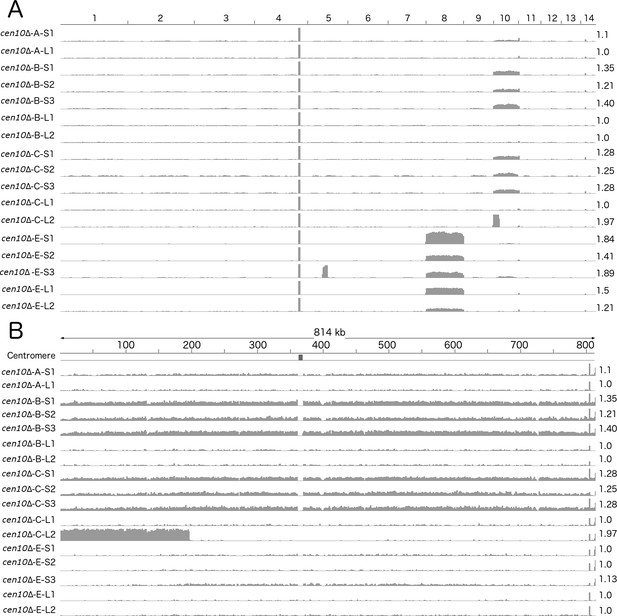
cen10∆ mutants are aneuploid.
The whole genomes of small and large colonies derived from four cen10∆ mutants were sequenced and read coverage (corresponding to ploidy levels) was plotted. Small colonies of cen10∆ mutants were partially aneuploid for chromosome 10, while the large colonies are euploid. (A) Genome-wide read depth coverage for small and large colonies. On the right, the fold coverage for the highest ploidy level is indicated for each sample. For example, chromosome 10 of cen10∆-B-S1 had an aneuploidy level of 1.35-fold compared to the wild-type strain. Chromosome 4 had a small region with increased read depth due to the ribosomal rDNA gene cluster and was excluded from the analysis. Chromosome 8 of cen10∆-E was duplicated. In addition, cen10∆-E-S3 had an additional duplicated region of 162 kb of chromosome 5 that spans the sequence of native centromere 5. (B) Detailed view of read depth of chromosome 10. As in panel A, read depth is indicated on the right. The native centromeric location is shown by a black square. Due to the deletion of centromere 10, the location of the native centromere lacks sequence reads for each sample.
Aneuploidy in C. neoformans often leads to a similar mixed colony size phenotype as that observed in the cen10∆ mutants (Sun et al., 2014). To exacerbate the aneuploidy-associated slow growth phenotype, four cen10∆ mutants were grown at elevated temperature (37°C), causing these isolates to produce smaller, growth-impaired and larger, growth-improved colonies (Figure 3—figure supplement 1). Three small and two large colonies were selected from each isolate and whole-genome analysis was performed based on Illumina sequencing. Sequences were mapped to the reference R265 genome, revealing that the small colonies were indeed aneuploid (Figure 4A). The small colonies of cen10∆-B and cen10∆-C had ploidy levels for chromosome 10 in the range of 1.25- to 1.36-fold higher compared to the other 13 chromosomes, which suggested that only a proportion of the cells (25% to 36%), were aneuploid (Figure 4B). The remainder of the genome was euploid. Chromosome 10 of the small colonies derived from isolate cen10∆-A and cen10∆-E exhibited ploidy levels ranging from 1.1- to 1.14-fold, reflecting less aneuploidy. Importantly, for all of the large colonies derived from isolates cen10∆-A, cen10∆-B, cen10∆-C, and cen10∆-E the fold coverage of chromosome 10 was restored to the wild-type euploid level (1.0 fold compared to wild type). The ploidy levels of chromosome 10 were 1-fold for all of the large colonies compared to wild type, indicating that the ploidy level of chromosome 10 of the large colonies was restored to euploid.
cen10∆ chromosome is rescued by chromosome fusion
Based on whole-genome sequencing and PFGE analysis, fusion of cen10∆ chromosome 10 to other chromosomes was a common event in the large colonies (Figure 5, Figure 6). Whole-genome sequence analysis revealed that sequences corresponding to the 3’ subtelomeric region of chromosome 10 (including one gene) were absent in the sequences obtained from all of the large colonies analyzed (Figure 4—figure supplement 1A). In addition, the large colonies of cen10∆-A were missing sequences for two genes in the 5’ subtelomeric region of chromosome 4 (Figure 4—figure supplement 1B). Large colonies of cen10∆-B were missing 18.5 kb at the 5’ subtelomere of chromosome 7 (including eight genes) (Figure 4—figure supplement 1C). The large colonies of cen10∆-E lacked a small part of one gene in the 3’ subtelomeric region of chromosome 1. In total, of the 14 subtelomeric genes that were lost in these three chromosome-fusion isolates, ten encoded hypothetical proteins and four encoded proteins with predicted functions. BlastN analysis in the de novo genome assemblies of the large colonies confirmed that the subtelomeric regions were not located on minichromosomes or inserted in other chromosomes. Seven genes have homologs in C. neoformans and are present in C. neoformans deletion libraries (Liu et al., 2008; Supplementary file 3). This observation suggested that either subtelomeric deletions occurred, or that chromosomal fusions led to the loss of subtelomeric regions. Notably, sequences from the small colonies spanned the entire genome with no evidence of these subtelomeric deletions (Figure 4—figure supplement 1F).
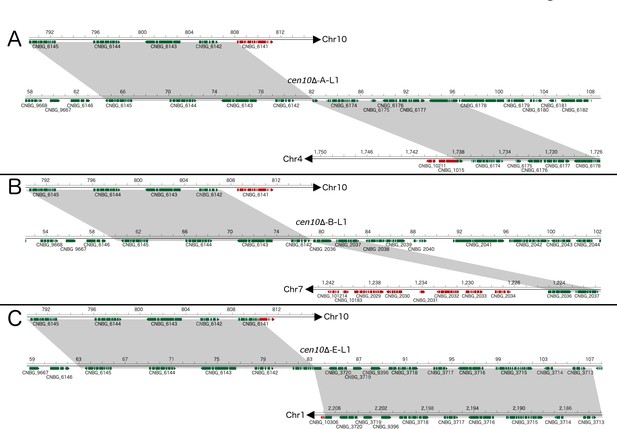
cen10∆ mutants undergo chromosome fusion leading to improved fitness at 37°C.
Chromosomal fusions were studied in detail for three cen10∆ mutants restored to wild-type growth levels at 37°C (large colonies). After chromosome fusion, the fused chromosomes of cen10∆-A-L and cen10∆-B-L lost the gene CNBG_6141, which is located in the 3’ subtelomeric region of chromosome 10. Genes present in the fused chromosome are depicted in green, and genes lost after chromosome fusion are indicated in red. Gray highlights indicate regions present in both the parental and fused chromosomes. Each fusion occurred in a unique nonrepetitive region. (A) cen10∆-A-L1, the fusion occurred between chromosome 10 and chromosome 4. (B) In cen10∆-B-L1, chromosomal fusion occurred between chromosomes 10 and 7. (C) cen10∆-E-L1 chromosomal fusion occurred between chromosomes 10 and 1.
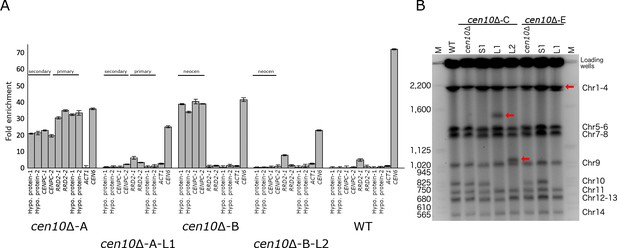
Chromosome fusion results in neocentromere inactivation and karyotype reduction.
(A) Neocentromeres are inactive after chromosomal fusion. For each neocentromere two qPCR primer pairs located in genes spanned by the neocentromere in cen10∆-A and cen10∆-B mutant were used in a ChIP-qPCR experiment. Analyzed is the CENP-A enrichment of 1) a cen10∆ mutant, 2) a large colony derived from the cen10∆ mutant, and 3) the wild-type strain. Centromere 6 (CEN6) was included as a positive control, and actin was included as a negative control. Data are shown for cen10∆-A, cen10∆-A-L1, cen10∆-B, cen10∆-B-L2, and wild type. For cen10∆-A and cen10∆-A-L1 mutants, the chromosomal regions investigated are indicated according to the primary and secondary CENP-A peaks of the cen10∆-A mutant. The cen10∆-B mutant has only one CENP-A-enriched region which co-localized with the secondary CENP-A peak of cen10∆-A and this region is labeled with neocen in cen10∆-B and cen10∆-B-L1. Error bars show standard deviation. (B) PFGE analysis shows that the band corresponding to chromosome 10 was lost in the large colonies and instead larger bands appear due to the fusion of chromosome 10 with other chromosomes. cen10∆ deletion mutants and small colonies derived from 37°C show a wild-type karyotype. Chromosome 10 of the large colonies was fused to chromosome 13, 10, or 1, respectively. Due to limitations of PFGE conditions, the chromosome 10–chromosome 1 fusion did not separate from chromosomes 2, 3, and 4. The positions of the fused chromosomes are indicated with arrows.
We hypothesized that the subtelomeric gene loss was due to chromosomal fusion and tested this hypothesis with de novo genome assemblies and PFGE (Figure 5 and Figure 6). Based on de novo genome assemblies for the large colonies of cen10∆-A, cen10∆-B, and cen10∆-E, chromosome 10 fused with chromosome 4, 7, or 1, respectively (Figure 5 and Supplementary file 3). In the large colony of cen10∆-A (cen10∆-A-L1), the fusion occurred between chromosome 10 and chromosome 4 (Figure 5A). Chromosomal fusion led to the loss of the CNBG_10211 gene (on chromosome 4), and the fusion junction was within the CNBG_6174 gene of chromosome 4. For cen10∆-B-L1, the chromosomal fusion occurred between chromosomes 10 and 7 (Figure 5B). Seven genes of chromosome 7 were lost in the fused chromosome. The chromosome fusion junction was intergenic on chromosome 7. cen10∆-E-L1 was due to a chromosomal fusion between chromosomes 10 and 1 (Figure 5C). The fusion was intragenic for both chromosomes. The fusion point occurred in CNBG_6141 on chromosome 10 and CNBG_10308 on chromosome 1.
Because all of the large cen10∆ colonies had chromosome 10 fusions, we examined the fusion location on chromosome 10 in detail. The fusions occurred 1.7, 0.3, and 3.6 kb from the chromosome 10 gene CNBG_6142, respectively (Figure 5—figure supplement 1). The fusion occurred in unique DNA sequences and was not flanked by repetitive regions. The overlapping region between chromosome 10 and the fused chromosome was at most 6 bp, suggesting that these fusions occurred via microhomology-mediated end joining (MMEJ) (also known as alternative nonhomologous end-joining [Alt-NHEJ]).
Chromosome fusion may result in loss of the neocentromeres, and the kinetochore may bind to the native centromere of the fused chromosome and function as the active centromere. This hypothesis was tested by performing ChIP-qPCR for CENP-A binding (Figure 6A). For each neocentromere (either of the cen10∆-A or cen10∆-B mutant), CENP-A enrichment was tested with four primer sets located in the neocentromere. The CENP-A enrichment for these four locations was tested in 1) the initial cen10∆ mutant, 2) a large colony derived from a specific cen10∆ mutant and 3) wild type (Figure 6A). As expected, the ChIP-qPCR analysis showed CENP-A enrichment for the neocentromeres of the initial cen10∆-A and cen10∆-B mutants. The neocentromeric regions of cen10∆-A and cen10∆-B were not enriched with CENP-A in the wild-type strain, showing there was no occupancy by CENP-A prior to neocentromere formation. For all analyzed cen10∆ chromosome 10 fusion isolates, the neocentromeres were not CENP-A-associated, and were similar to the wild-type background levels. Therefore, the neocentromeres were no longer active in the chromosome fusion strains (Figure 6A). This suggests that the native centromere of the fusion partner of chromosome 10 (i.e., chromosome 1, 4, or 7) was the active centromere of the Chr10-Chr1, Chr10-Chr4, and Chr10-Chr7 fusions.
In addition to cen10∆-A, -B, and -E mutants, whole-genome sequencing was performed for two large colonies of cen10∆-C. Although it was not possible to identify the chromosome fusion based on whole-genome sequencing data for either of the large colonies of the cen10∆-C mutant, PFGE analysis showed that cen10∆-C-L1 had a fusion between chromosomes 10 and 13 (Figure 6b). cen10∆-C-L2 had read coverage of 1.99-fold for a region of ~200 kb of chromosome 10 (Figure 4B). The rest of chromosome 10 was euploid, suggesting that the ~200 kb region was duplicated and was either a single chromosome or fused to another chromosome in this isolate. PFGE analysis suggested that this fragment was duplicated on chromosome 10, resulting in a larger chromosome (Figure 6B). In contrast to the other fused chromosomes, this chromosomal fragment did not fuse to a chromosome with a native centromere, and the fact that the mutant still exhibited a fitness defect was consistent with this interpretation. The larger chromosome was euploid, suggesting that the unstable neocentromere(s), rather than causing aneuploidy, resulted in a fitness cost in this isolate.
Discussion
Composition of neocentromeres in C. deuterogattii
The native centromeres of C. deuterogattii are found in repetitive regions and are flanked by, but do not contain, protein-encoding genes (Yadav et al., 2018). By contrast, neocentromeres of C. deuterogattii span genes, lack repetitive elements, and like the native centromeres, are flanked by genes. In general (with one exception), the neocentromeres of C. deuterogattii are significantly shorter than the native centromeres, whereas most neocentromeres in other species have similar lengths as the native centromeres.
Native centromeres of S. pombe have a central core that is enriched with CENP-A and flanked by repetitive pericentric regions (Ishii et al., 2008). While neocentromere formation in S. pombe favors repeats in the pericentric regions, neocentromere formation is possible without the repetitive pericentric regions (Ishii et al., 2008). The majority of the neocentromeres in C. albicans and chickens are formed close to native centromeres due to seeding of CENP-A that is located near the native centromere (the so-called CENP-A cloud) (Ketel et al., 2009; Shang et al., 2013). Our results and the earlier reports discussed, suggest that the chromosomal location of the native centromere is the main determinant of neocentromere formation. One exception was the neocentromere of cen10∆-E, which directly flanked the left telomere. Interestingly, this was the only cen10∆ mutant that had a growth rate similar to wild type.
Several C. deuterogattii neocentromeres formed in the same location; however, there is no apparent consensus between the different regions occupied by different neocentromeres. A similar trend has been observed in neocentromere formation in C. albicans (Ketel et al., 2009). Evolutionary new centromeres (ECNs) in the largest crucifer tribe Arabideae originated several times independently and are located in the same chromosomal location (Mandáková et al., 2020). Our results suggest that neocentromeres form by mechanisms that do not rely on nearby transposable elements/repeats to initiate de novo centromere assembly.
Neocentromeric genes are expressed
Neocentromeres induced in several species can span genes, resulting in silencing or reduced gene expression. For example, all genes within five independent neocentromeres in C. albicans that spanned nine genes were suppressed (Burrack et al., 2016). In S. pombe, neocentromeres span genes that are only expressed in response to nitrogen starvation in the wild-type strain, and neocentromere formation silences these genes during nitrogen starvation (Ishii et al., 2008). The native centromere 8 of rice contains an approximately 750 kb CENP-A-enriched region with four genes that are expressed in both leaf and root tissues of three closely related species (Fan et al., 2011; Nagaki et al., 2004). Neocentromeres of rice span genes that are expressed at similar levels as in the wild type (Zhang et al., 2013). Chicken neocentromeres have been induced on chromosome Z or 5 (Shang et al., 2013). Chromosome Z neocentromeres span eight genes, but in wild-type cells only MAMDC2 is expressed during normal growth. The other seven genes were either not expressed at any detectable level in all tested developmental stages or were only expressed during early embryonic stages (Shang et al., 2013). When a neocentromere formed, expression of the MAMDC2-encoding gene was reduced 20- to 100-fold. Chromosome 5 of chickens is diploid, and neocentromeres on this chromosome span genes that are expressed. The hypothesis behind this phenomenon is that one allele functions as a centromere, while the other allele codes for the genes.
Because the cen10∆ mutants of C. deuterogattii were aneuploid, the expression of genes spanned by chromosome 10 neocentromeres was normalized to expression levels of a housekeeping gene located on chromosome 10. The expression of genes enriched for CENP-A chromatin is similar to that of wild type, and if the allelic hypothesis, like in chickens, were valid, the expectation would be a 60% reduction in expression levels. The genes spanned by neocentromeres of cen9∆ mutants are also expressed at wild-type levels. As the cen9∆ mutants have uniform, wild-type colony sizes, the ploidy levels of these mutants were not tested and we hypothesize that these mutants are haploid/euploid.
Genes contained in regions in which C. deuterogattii neocentromeres formed in cenΔ mutants were actively expressed in the wild-type strain, and this is similar to human neocentromeres that can form in regions with or without gene expression (Alonso et al., 2010; Marshall et al., 2008). However, we have identified chromosomal regions that lack gene expression on chromosomes 9 and 10, although these regions were not close to the native centromere.
Of the C. deuterogattii genes spanned by the neocentromere region, one encodes the kinetochore component CENP-C. Several independent biolistic transformations were performed to delete the gene encoding CENP-C, but all attempts were unsuccessful. This suggests that CENPC is an essential gene. In fission yeast, deletion of the gene encoding the CENP-C homolog Cnp3 was lethal at 36°C, but mutants were still viable at 30°C (Suma et al., 2018). However, CENP-A was mislocalized in the cnp3∆ mutants. Another gene partially located inside a C. deuterogattii neocentromere encodes the serine/threonine-protein phosphatase 2A activator 2 (RRD2). The RRD2 homolog is not essential in S. cerevisiae (Higgs and Peterson, 2005).
Compared with other haploid fungi, the neocentromeric genes of C. deuterogattii are similar to the native centromeric genes of the haploid plant pathogenic fungus Zymoseptoria tritici. Z. tritici has short regional centromeres with an average size of 10.3 kb, and 18 out of 21 native centromeres have a total of 39 expressed genes (Schotanus et al., 2015).
cen10∆ mutants with two CENP-A-enriched regions
The appearance of two CENP-A-enriched regions of C. deuterogattii cen10∆ mutants could be explained in a few ways. First, neocentromere formation could lead to a dicentric chromosome 10 in which the centromeres may differ in functional capacity. Dicentric chromosomes are not by definition unstable, for example the dominant-negative mutation of the mammalian telomere protein TRF2 results in chromosome fusions, leading to the formation of dicentric chromosomes (Stimpson et al., 2010). The formation of dicentric chromosomes occurred in 97% of the fused mammalian chromosomes, which were stable for at least 180 cell divisions (Stimpson et al., 2010). Several microscopic studies showed that chromosomes with two regions of centromere-protein enrichment are stable (Higgins et al., 2005; Stimpson et al., 2012; Stimpson et al., 2010; Sullivan and Willard, 1998). This suggests that a dineocentric chromosome 10 could be stable in the population. Second, the two CENP-A-enriched peaks could be the result of a mixed population and either due to an unstable primary neocentromere and/or aneuploidy. The primary neocentromere could be associated with the majority of the cells, whereas the secondary CENP-A peak would be only found in a small number of cells (and the primary neocentromere is lost in these isolates). This is reflected by lower CENP-A enrichment for the secondary peak, and the hypothesis of putative dicentrics is due to a mixture of alleles in the population. Third, the neocentromeres could be unstable, which could lead to the formation of two CENP-A-enriched regions with centromere function switching between the regions. However, our data would argue against this latter model. Prior to the ChIP-seq analysis of the cen10∆ mutants, colonies were isolated by streak purification (eight times), suggesting that the presence of two distinct CENP-A peaks occurs continuously.
cen10∆ mutants are partially aneuploid
Neocentromere formation in chickens results in a low number of aneuploid cells (Shang et al., 2013). Based on whole-genome sequencing of a population of cells, the C. deuterogattii cen10∆ isolates are partially aneuploid for chromosome 10. For fully aneuploid isolates, the coverage of Illumina reads is expected to be 2-fold; the cen10∆ isolates with two CENP-A peaks showed aneuploidy levels up to 1.28-fold or were even euploid. This suggests that, like the chicken neocentromeric isolates, only a small number of cells in a population of C. deuterogattii cen10∆ isolates are aneuploid.
cen10∆ mutants have reduced fitness
In C. albicans, deletion of centromere 5 results in neocentromere formation, and these isolates have fitness similar to the wild-type strain (Ketel et al., 2009). Similar results were reported for neocentromeres in chicken and S. pombe, in which strains with neocentromeres or chromosome fusion have a growth rate similar to the wild-type strain (Ishii et al., 2008; Shang et al., 2013).
If centromere deletions occurred in nature, we hypothesize that the wild type would outcompete all of the cen∆ isolates. The virulence of the cen∆ mutants was not assayed. Based on reduced fitness of the cen∆ mutants, we hypothesize that pathogenicity of the cen∆ mutants would be lower than the wild type. However, when chromosome fusion occurs the growth rate is restored to a near wild-type level and we hypothesize that the isolates with 13 chromosomes could have virulence similar to the wild type. Several genes were lost due to the fusion events in the cen∆ mutants; to our knowledge these lost genes have not been associated with pathogenicity of C. deuterogattii.
Neocentromere stains exhibit impaired growth and chromosome fusion restores wild-type growth at elevated temperatures
Deletion of a centromere in S. pombe leads to either neocentromere formation or chromosome fusion due to a noncanonical homologous recombination pathway (Ishii et al., 2008; Ohno et al., 2016). This is in contrast to neocentromere formation in C. deuterogattii, which results in 100% neocentromere formation. Based on PFGE analysis, the karyotype of the cenΔ isolates is wild type at 30°C, but chromosome fusion can occur at 37°C within the cen10∆ mutants and lead to improved growth at 30°C.
The location of the cen10∆ neocentromere had no apparent influence on the ability to undergo chromosome fusion as we have shown with a telocentric neocentromere, a dicentric mutant, and a neocentromere located 118 kb away from the telomere.
The fused chromosomes have no or only short homology at the breakpoints that is insufficient for homologous recombination, suggesting that the chromosome fusions arise via MMEJ. Future experiments to test this hypothesis could involve deleting genes involved in the MMEJ pathway, such as CDC9 and DNL4 (Sinha et al., 2016).
A prominent chromosome fusion occurred during the speciation of humans. Compared to other great apes, humans have a reduced karyotype, which is due to the fusion of two ancestral chromosomes that resulted in chromosome 2 in modern humans, Denisovans, and Neanderthals (Miga, 2017). Human chromosome2 still harbors signatures of telomeric repeats at the fusion point (interstitial telomeric sequences [ITS]), suggesting that this chromosome is derived from a telomere-telomere fusion. By synteny analysis, the inactive centromere of chimpanzee chromosome 2b can be identified on human chromosome 2, and there are relics of α satellite DNA at this now extinct centromere (Miga, 2017). Moreover, a dominant-negative mutation of the human telomeric protein TRF2 leads to telomere-telomere fusions, mainly between acrocentric chromosomes (Stimpson et al., 2010; van Steensel et al., 1998). In the fungal species Malassezia, chromosome breakage followed by chromosome fusion has led to speciation (Sankaranarayanan et al., 2020). The short regional centromeres (3–5 kb) are fragile and this led most likely to chromosome reduction. By contrast in C. deuterogattii, the chromosomes involved in chromosomal fusion of the cen10∆ mutants were all metacentric, and fusion occurred in non-telomeric sequences.
Another example of telomeric fusions is the presence of ITS regions in several genomes. In budding yeast, the experimental introduction of an ITS into an intron of the URA3 gene resulted in four classes of chromosome rearrangements, including: 1) inversion, 2) gene conversion, 3) mini-chromosome formation due to deletion or duplication, and 4) mini-chromosome formation due to translocation (Aksenova et al., 2013). Based on our de novo genome assemblies of the C. deuterogattii large-colony cen10∆ mutants, chromosome fusions occurred with no signs of chromosome rearrangements. Thus, these chromosome fusions did not produce ITS regions, which would otherwise destabilize the genome.
Conclusions
Our work shows that, like in other model systems, neocentromeres can be induced in C. deuterogattii. However, C. deuterogattii neocentromeres have several unique characteristics, such as spanning genes whose expression is unaffected by centromere assembly. In some instances, deletion of CEN10 led to chromosome fusion, resulting in enhanced fitness and leading to inactivation of the neocentromere. Presumably, deletion of other centromeres could be carried out, leading to a C. deuterogattii strain with only one or a few chromosomes, as was recently reported in S. cerevisiae (Luo et al., 2018; Shao et al., 2018).
Materials and methods
Key Resources Table Template and Guidelines.
Reagent type (species) or resource | Designation | Source or reference | Identifiers | Additional information |
---|---|---|---|---|
Genetic reagent Cryptococcus deuterogattii | R265 | This study | R265 expressing mCherry-CENPA | |
Genetic reagent Cryptococcus deuterogattii | cen10△-A | This study | R265 centromere 10 deletion mutant with expressing mCherry-CENPA | |
Genetic reagent Cryptococcus deuterogattii | cen10△-B | This study | R265 centromere 10 deletion mutant with expressing mCherry-CENPA | |
Genetic reagent Cryptococcus deuterogattii | cen10△-C | This study | R265 centromere 10 deletion mutant with expressing mCherry-CENPA | |
Genetic reagent Cryptococcus deuterogattii | cen10△-D | This study | R265 centromere 10 deletion mutant with expressing mCherry-CENPA | |
Genetic reagent Cryptococcus deuterogattii | cen10△-E | This study | R265 centromere 10 deletion mutant with expressingmCherry-CENPA | |
Genetic reagent Cryptococcus deuterogattii | cen10△-F | This study | R265 centromere 10 deletion mutant with expressing mCherry-CENPA | |
Genetic reagent Cryptococcus deuterogattii | cen10△-G | This study | R265 centromere 10 deletion mutant with expressing mCherry-CENPA | |
Genetic reagent Cryptococcus deuterogattii | cen10△-A-S1 | This study | Small colony derived from R265 centromere 10A deletion mutant with expressing mCherry-CENPA | |
Genetic reagent Cryptococcus deuterogattii | cen10△-A-L1 | This study | Large colony derived from R265 centromere 10A deletion mutant with expressingmCherry-CENPA | |
Genetic reagent Cryptococcus deuterogattii | cen10△-B-S1 | This study | Small colony derived from R265 centromere 10B deletion mutant with expressing mCherry-CENPA | |
Genetic reagent Cryptococcus deuterogattii | cen10△-B-S2 | This paper | Small colony derived from R265 centromere 10B deletion mutant with expressing mCherry-CENPA | |
Genetic reagent Cryptococcus deuterogattii | cen10△-B-S3 | This study | Small colony derived from R265 centromere 10B deletion mutant with expressing mCherry-CENPA | |
Genetic reagent Cryptococcus deuterogattii | cen10△-B-L1 | This study | Large colony derived from R265 centromere 10B deletion mutant with expressing mCherry-CENPA | |
Genetic reagent Cryptococcus deuterogattii | cen10△-B-L2 | This study | Large colony derived from R265 centromere 10B deletion mutant with expressing mCherry-CENPA | |
Genetic reagent Cryptococcus deuterogattii | cen10△-C-S1 | This study | Small colony derived from R265 centromere 10C deletion mutant with expressing mCherry-CENPA | |
Genetic reagent Cryptococcus deuterogattii | cen10△-C-S2 | This study | Small colony derived from R265 centromere 10C deletion mutant with expressing mCherry-CENPA | |
Genetic reagent Cryptococcus deuterogattii | cen10△-C-S3 | This study | Small colony derived from R265 centromere 10C deletion mutant with expressing mCherry-CENPA | |
Genetic reagent Cryptococcus deuterogattii | cen10△-C-L1 | This study | Large colony derived from R265 centromere 10C deletion mutant with expressing mCherry-CENPA | |
Genetic reagent Cryptococcus deuterogattii | cen10△-C-L2 | This study | Large colony derived from R265 centromere 10C deletion mutant with expressing mCherry-CENPA | |
Genetic reagent Cryptococcus deuterogattii | cen10△-E-S1 | This study | Small colony derived from R265 centromere 10E deletion mutant with expressing mCherry-CENPA | |
Genetic reagent Cryptococcus deuterogattii | cen10△-E-S2 | This study | Small colony derived from R265 centromere 10E deletion mutant with expressing mCherry-CENPA | |
Genetic reagent Cryptococcus deuterogattii | cen10△-E-S3 | This study | Small colony derived from R265 centromere 10E deletion mutant with expressing mCherry-CENPA | |
Genetic reagent Cryptococcus deuterogattii | cen10△-E-L1 | This study | Large colony derived from R265 centromere 10E deletion mutant with expressing mCherry-CENPA | |
Genetic reagent Cryptococcus deuterogattii | cen10△-E-L2 | This study | Large colony derived from R265 centromere 10E deletion mutant with expressing mCherry-CENPA | |
Genetic reagent Cryptococcus deuterogattii | cen9△-A | This study | R265 centromere 9 deletion mutant with expressing mCherry-CENPA | |
Genetic reagent Cryptococcus deuterogattii | cen9△-B | This study | R265 centromere 9 deletion mutant with expressing mCherry-CENPA | |
Genetic reagent Cryptococcus deuterogattii | cen9△-C | This study | R265 centromere 9 deletion mutant with expressing mCherry-CENPA | |
Genetic reagent Cryptococcus deuterogattii | cen9△-D | This study | R265 centromere 9 deletion mutant with expressing mCherry-CENPA | |
Genetic reagent Cryptococcus deuterogattii | cen9△-E | This study | R265 centromere 9 deletion mutant with expressing mCherry-CENPA | |
Genetic reagent Cryptococcus deuterogattii | cen9△-F | This study | R265 centromere 9 deletion mutant with expressing mCherry-CENPA | |
Genetic reagent Cryptococcus deuterogattii | R265 MIS12 | This study | R265 expressing GFP-MIS12 and mCherry-CENPA | |
Genetic reagent Cryptococcus deuterogattii | cen10△-A MIS12 | This study | R265 Centromere 10 mutant with expressing GFP-MIS12 and mCherry-CENPA | |
Genetic reagent Cryptococcus deuterogattii | cen10△-B MIS12 | This study | R265 Centromere 10 mutant with expressing GFP-MIS12 and mCherry-CENPA | |
Genetic reagent Cryptococcus deuterogattii | cen10△-C MIS12 | This study | R265 Centromere 10 mutant with expressing GFP-MIS12 and mCherry-CENPA | |
Genetic reagent Cryptococcus deuterogattii | cen10△-D MIS12 | This study | R265 Centromere 10 mutant with expressing GFP-MIS12 and mCherry-CENPA | |
Genetic reagent Cryptococcus deuterogattii | cen10△-E MIS12 | This study | R265 Centromere 10 mutant with expressing GFP-MIS12 and mCherry-CENPA | |
Genetic reagent Cryptococcus deuterogattii | R265 CENPC | This study | R265 with expressing GFP-CENPC and mCherry-CENPA | |
Genetic reagent Cryptococcus deuterogattii | cen9△-A CENPC | This study | R265 Centromere 9 mutant with expressing GFP-CENPC and mCherry-CENPA | |
Genetic reagent Cryptococcus deuterogattii | cen9△-B CENPC | This study | R265 Centromere 9 mutant with expressing GFP-CENPC andmCherry-CENPA | |
Genetic reagent Cryptococcus deuterogattii | cen9△-C CENPC | This study | R265 Centromere 9 mutant with expressing GFP-CENPC andmCherry-CENPA | |
Genetic reagent Cryptococcus deuterogattii | cen9△-D CENPC | This study | R265 Centromere 9 mutant with expressing GFP-CENPC and mCherry-CENPA | |
Genetic reagent Cryptococcus deuterogattii | cen9△-E CENPC | This study | R265 Centromere 9 mutant with expressing GFP-CENPC and mCherry-CENPA | |
Antibody | Anti-mCherry antibody(Rabbit polyclonal) | Abcam | Cat. no. ab183628 | ChIP (1/5000) |
Antibody | Anti-GFP (Rabbit polyclonal) antibody | Abcam | Cat. no. ab290 | ChIP (5 µg for 1 µg of chromatin) |
Other | Dynabeads Protein A for Immunoprecipitation | Invitrogen | Cat. no. 10001D | ChIP (20 µl per500 µl fraction) |
Software, algorithm | Bowtie2 | Langmead, 2010 | ||
Software, algorithm | Spades | Bankevich et al., 2012 | ||
Software, algorithm | IGV | Thorvaldsdóttir et al., 2013 | ||
Software, algorithm | HISAT2 | Pertea et al., 2016 | ||
Sequence-based reagent | List of primers used in this study | Sigma | In Supplementary file 4 |
Strains, primers, and culture conditions
Request a detailed protocolPrimers are listed in Supplementary file 4. Strains used in this study are listed in Supplementary file 5. All strains were stored in glycerol at −80°C, inoculated on solid YPD (yeast extract, peptone, and dextrose) media, and grown for two days at 30°C. Liquid YPD cultures were inoculated from single colonies of solid media and grown, while shaking, at 30°C overnight.
Genetic manipulations
Request a detailed protocolDNA sequences (1 to 1.5 kb) of the CEN10-flanking regions were PCR-amplified with Phusion High-Fidelity DNA Polymerase (NEB, Ipswich MA, USA). Flanking regions were fused on both sides of either the NEO or NAT dominant selectable marker via overlap PCR, conferring G418 or nourseothricin resistance, respectively. Deletion of C. deuterogattii CEN10 was achieved through homologous recombination via biolistic introduction of an overlap-PCR product as previously described (Billmyre et al., 2017; Davidson et al., 2002). Deletion of CEN9 was performed by CRISPR-CAS9 mediated transformation with two guide RNAs flanking CEN9 and homologous recombination was mediated by the introduction of an overlap PCR product as previously described (Fan and Lin, 2018). Transformants were selected on YPD medium containing G418 (200 μg/mL) or nourseothricin (100 μg/mL).
Subsequently, the 5’ junction, 3’ junction, and spanning PCR and Southern blot analyses were performed to confirm the correct replacement of CEN10 by the appropriate drug resistance marker. To identify centromeres, the gene CNBG_0491, which encodes CENP-A, was N-terminally fused to the gene encoding the fluorescent mCherry protein by overlap PCR, and C. deuterogattii strains were biolistically transformed as previously described (Billmyre et al., 2017). A subset of cen9∆ mutants were biolistically transformed with an overlap PCR product containing CENPC C-terminally fused with GFP. As three cen10∆ mutants have a neocentromere that spans the gene encoding CENPC, a subset of cen10∆ mutants were transformed instead with an overlap PCR product containing MIS12 C-terminally fused with GFP. Both PCR products encoding CENPC-GFP and MIS12-GFP were randomly integrated in the genome and confirmed by a PCR spanning either CENPC-GFP or MIS12-GFP.
Growth and competition assays
Request a detailed protocolThree replicate cultures for seven independent cen10∆ deletion mutants and the wild-type strain were grown in liquid YPD at 30°C overnight. Cells were diluted to an OD600 of 0.01 and grown in 50 mL YPD at 30°C. The OD600 of the triplicate cultures was measured every two hours with a SmartSpec 3000 (BioRad) until stationary phase was reached (T = 22 hr).
For competition assays, three independent replicate cultures (cen9∆, cen10∆, control, and wild type) were grown overnight in 8 mL YPD. Subsequently, the cell density of the cultures was determined using a hemocytometer. For each independent cenΔ deletion mutant, 500,000 cells were co-cultured in a 1:1 ratio with wild-type cells. After 24 hr, the cultures were inoculated on 1) a YPD plate to determine the total colony-forming units (CFUs) and 2) a YPD plate containing G418 or nourseothricin to calculate the proportion of cen10∆ mutant CFUs compared to the wild-type CFUs. Plates were incubated at 30°C and the colonies were counted after 4 days. The cell morphology of >1000 cells of the wild type and of five cen10∆ mutant strains was analyzed, and the number of elongated cells was quantified (Figure 1—figure supplement 5).
Whole-genome sequencing, read mapping for aneuploidy/RNA-seq, and de novo genome assemblies
Request a detailed protocolGenomic DNA was isolated using the CTAB protocol and sent to the Duke University Sequencing and Genomic Technologies Shared Resource facility for library preparation and Illumina sequencing. Sequencing was performed with a HiSeq 4000 sequencer, and 150 bp paired-end reads were generated. The resulting DNA sequence reads were trimmed, quality-filtered, and subsequently mapped with Bowtie2 to a complete PacBio, Nanopore-based, Illumina Pilon-corrected, whole-genome assembly of the C. deuterogattii R265 reference genome (version R265_fin_nuclear). Reads were visualized with IGV (Langmead, 2010; Quinlan and Hall, 2010; Thorvaldsdóttir et al., 2013; Yadav et al., 2018). Previously generated RNA sequencing reads (NCBI, SRA: SRR5209627) were remapped to the C. deuterogattii R265 reference genome by HISAT2 according to the default settings (Schneider et al., 2012; Pertea et al., 2016).
Genomes were de novo assembled with Spades using the default conditions (Bankevich et al., 2012). Genome assemblies were confirmed with PCRs using primers flanking the chromosome fusions and the PCR products obtained span the chromosomal fusions (Figure 5—figure supplement 1). The read coverage at chromosome fusions was analyzed and compared to the average read coverage of the contig (Figure 5—figure supplement 1).
Chromatin immunoprecipitation (ChIP) followed by high-throughput sequencing or qPCR
Request a detailed protocolChIP analyses were performed as previously described with minor modifications (Schotanus et al., 2015; Soyer et al., 2015). In short, 500 mL YPD cultures (1000 ml YPD for Mis12 ChIPs) were grown overnight at 30°C, after which 37% formaldehyde was added to a final concentration of 0.5% for crosslinking. The cultures were then incubated for 15 min, formaldehyde was quenched with 2.5 M glycine (1/20 vol), and cells were washed with cold PBS. The crosslinking time of Mis12-GFP tagged isolates was extended to 45 min. Cells were resuspended in chromatin buffer (50 mM HEPES-NaOH, pH 7.5; 20 mM NaCl; 1 mM Na-EDTA, pH 8.0; 1% [v/v] Triton X-100; 0.1% [w/v] sodium deoxycholate [DOC]) containing protease inhibitors (cOmplet Tablets, mini EDTA-free EASYpack, Roche), followed by homogenization by bead beating with a miniBead beater (BioSpec products) using 18 cycles of 1.5 min on and 1.5 min off. The supernatant containing chromatin was sheared by sonication (24 cycles of 15 s on, 15 s off, burst at high level) (Bioruptor UCD-200, Diagenode). Chromatin was isolated by centrifugation, and the supernatant was divided into a sample fraction and a sonication control. The sample fraction was precleared with protein-A beads (1 to 3 hr) and subsequently divided into two aliquots. One tube served as the input control, and a mCherry or GFP antibody (MCherry: ab183628, Abcam, GFP: ab290, Abcam) was added to the remaining half of the sample. The samples were incubated overnight at 4°C and then processed according to a previously published protocol (Soyer et al., 2015). After completing the ChIP experiment, the samples were analyzed by ChIP-qPCR or sent to the Duke University Sequencing and Genomic Technologies Shared Resource facility for library preparation and Illumina sequencing. Samples cen10∆-A, cen10∆-B and cen10∆-C were sequenced with a HiSeq 2500 sequencer, and single reads of 50 bp were obtained. All other ChIP-seq samples were sequenced with a NovaSeq 600 sequencer and 50 bp PE reads were obtained. For each centromere mutant and the wild type, a ChIPed and input sample were sequenced. Reads were mapped to the reference genome, similar to the whole-genome sequencing reads. To analyze the ChIP-seq data the ChIPed sample was normalized with the input sample and visualized with the IGV viewer.
RNA isolation and qPCRs
Request a detailed protocolCells were grown in an overnight culture of 25 mL YPD at 30°C. RNA was isolated with TRIzol LS (Thermo Fisher Scientific) according to the manufacturer’s instructions. Subsequently, cDNA was synthesized with the SuperScriptFirst-Strand Synthesis System (Thermo Fisher Scientific) according to the manufacturer’s instructions. qPCRs were performed in triplicate with Brilliant III Ultra-Fast SYBR Green qPCR Master Mix (Agilent Technologies) on an ABI 1900HT qPCR machine.
Pulsed-field gel electrophoresis (PFGE)
Request a detailed protocolIsolation of whole chromosomes and conditions for PFGE and chromoblot analysis were performed as previously described (Findley et al., 2012).
Data availability
ChIP and whole-genome sequencing reads and de novo genome assemblies were deposited under NCBI BioProject Accession ID: PRJNA511460.
-
NCBI BioProjectID PRJNA511460. Neocentromere formation in Cryptococcus deuterogattii.
-
NCBI Sequence Read ArchiveID SRR5209627. Cryptococcus gattii R265 mRNA RNA-Seq.
References
-
A paucity of heterochromatin at functional human neocentromeresEpigenetics & Chromatin 3:6.https://doi.org/10.1186/1756-8935-3-6
-
SPAdes: a new genome assembly algorithm and its applications to single-cell sequencingJournal of Computational Biology 19:455–477.https://doi.org/10.1089/cmb.2012.0021
-
Neocentromeres and Epigenetically inherited features of centromeresChromosome Research 20:607–619.https://doi.org/10.1007/s10577-012-9296-x
-
The kinetochoreCold Spring Harbor Perspectives in Biology 6:a015826.https://doi.org/10.1101/cshperspect.a015826
-
Centromeres and Kinetochores85–109, Centrochromatin of fungi, Centromeres and Kinetochores, Springer International Publishing, 10.1007/978-3-319-58592-5.
-
Mouse centric and pericentric satellite repeats form distinct functional heterochromatinJournal of Cell Biology 166:493–505.https://doi.org/10.1083/jcb.200403109
-
Epigenetic inheritance of centromeresCold Spring Harbor Symposia on Quantitative Biology 75:51–60.https://doi.org/10.1101/sqb.2010.75.001
-
Engineered human dicentric chromosomes show centromere plasticityChromosome Research 13:745–762.https://doi.org/10.1007/s10577-005-1009-2
-
Phylogenetic analysis of the formin homology 2 domainMolecular Biology of the Cell 16:1–13.https://doi.org/10.1091/mbc.e04-07-0565
-
Aligning short sequencing reads with bowtieCurrent Protocols in Bioinformatics 32:11.https://doi.org/10.1002/0471250953.bi1107s32
-
Neocentromeres: new insights into centromere structure, disease development, and karyotype evolutionThe American Journal of Human Genetics 82:261–282.https://doi.org/10.1016/j.ajhg.2007.11.009
-
Alpha satellite DNA biology: finding function in the recesses of the genomeChromosome Research 26:115–138.https://doi.org/10.1007/s10577-018-9582-3
-
Centromere size and position in Candida albicans are evolutionarily conserved independent of DNA sequence heterogeneityMolecular Genetics and Genomics 278:455–465.https://doi.org/10.1007/s00438-007-0263-8
-
Sequencing of a rice centromere uncovers active genesNature Genetics 36:138–145.https://doi.org/10.1038/ng1289
-
Neocentromeres: a place for everything and everything in its placeTrends in Genetics 30:66–74.https://doi.org/10.1016/j.tig.2013.11.003
-
Risky business: microhomology-mediated end joiningMutation Research/Fundamental and Molecular Mechanisms of Mutagenesis 788:17–24.https://doi.org/10.1016/j.mrfmmm.2015.12.005
-
Heterochromatin is required for normal distribution of Neurospora crassa CenH3Molecular and Cellular Biology 31:2528–2542.https://doi.org/10.1128/MCB.01285-10
-
Stable dicentric X chromosomes with two functional centromeresNature Genetics 20:227–228.https://doi.org/10.1038/3024
-
Fission yeast CENP-C (Cnp3) plays a role in restricting the site of CENP-A accumulationG3: Genes|Genomes|Genetics 8:2723–2733.https://doi.org/10.1534/g3.118.200486
-
Integrative genomics viewer (IGV): high-performance genomics data visualization and explorationBriefings in Bioinformatics 14:178–192.https://doi.org/10.1093/bib/bbs017
-
Epigenetic origin of evolutionary novel centromeresScientific Reports 7:41980.https://doi.org/10.1038/srep41980
-
Chromosomal dynamics of human neocentromere formationChromosome Research 12:617–626.https://doi.org/10.1023/B:CHRO.0000036585.44138.4b
Article and author information
Author details
Funding
NIH (AI050113-15)
- Joseph Heitman
NIH (AI039115-22)
- Joseph Heitman
CIFAR (Fungal Kingdom: Threats and Opportunities)
- Joseph Heitman
The funders had no role in study design, data collection and interpretation, or the decision to submit the work for publication.
Acknowledgements
We thank Tom Petes, Beth Sullivan, Kaustuv Sanyal, Sue Jinks-Robertson, Vikas Yadav, Shelby Priest, Shelly Clancey, and Inge van der Kloet for comments on the manuscript. We would like to thank all of the members of the Heitman and Sanyal labs who contribute to the bi-weekly Skype meeting. These studies were supported by NIH/NIAID grants R01 AI050113-15 and R37 MERIT award AI039115-22 to JH. JH is co-director and fellow of the CIFAR program Fungal Kingdom: Threats and Opportunities.
Copyright
© 2020, Schotanus and Heitman
This article is distributed under the terms of the Creative Commons Attribution License, which permits unrestricted use and redistribution provided that the original author and source are credited.
Metrics
-
- 1,656
- views
-
- 163
- downloads
-
- 24
- citations
Views, downloads and citations are aggregated across all versions of this paper published by eLife.