Intravascular flow stimulates PKD2 (polycystin-2) channels in endothelial cells to reduce blood pressure
Abstract
PKD2 (polycystin-2, TRPP1), a TRP polycystin channel, is expressed in endothelial cells (ECs), but its physiological functions in this cell type are unclear. Here, we generated inducible, EC-specific Pkd2 knockout mice to examine vascular functions of PKD2. Data show that a broad range of intravascular flow rates stimulate EC PKD2 channels, producing vasodilation. Flow-mediated PKD2 channel activation leads to calcium influx that activates SK/IK channels and eNOS serine 1176 phosphorylation in ECs. These signaling mechanisms produce arterial hyperpolarization and vasodilation. In contrast, EC PKD2 channels do not contribute to acetylcholine-induced vasodilation, suggesting stimulus-specific function. EC-specific PKD2 knockout elevated blood pressure in mice without altering cardiac function or kidney anatomy. These data demonstrate that flow stimulates PKD2 channels in ECs, leading to SK/IK channel and eNOS activation, hyperpolarization, vasodilation and a reduction in systemic blood pressure. Thus, PKD2 channels are a major component of functional flow sensing in the vasculature.
Introduction
Endothelial cells line the lumen of all blood vessels and regulate multiple functions, including contractility. A wide variety of different stimuli act through endothelial cells to control arterial contractility, including receptor ligands, such as acetylcholine (ACh), and mechanical force, including intravascular flow. Mechanisms by which endothelial cells regulate arterial contractility include the production of diffusible substances, such as nitric oxide (NO) and hydrogen sulfide, and the release of endothelium-derived hyperpolarizing factors, including potassium (K+) (Vane, 1994; Edwards et al., 1998; Leffler et al., 2006). Due to the presence of myoendothelial gap junctions, endothelial cells can also directly control smooth muscle cell membrane potential to regulate arterial contractility (Garland et al., 2011). Less well defined are signaling mechanisms by which physiological stimuli activate these processes in endothelial cells to produce vasodilation. In particular, the regulatory mechanisms, physiological functions and in vivo significance of many ion channels that are expressed in endothelial cells are poorly understood.
Endothelial cells express several different families of ion channels, including multiple transient receptor potential (TRP), small-conductance Ca2+-activated K+ (SK3, KCa2.3) and intermediate-conductance Ca2+-activated K+ (IK, KCa3.1) proteins (Jackson, 2016). TRP channels are a family of ~28 proteins that are subdivided into six different classes, including polycystin (TRPP), melastatin (TRPM), ankyrin (TRPA), canonical (TRPC) and vanilloid (TRPV) (Earley and Brayden, 2015). Studies performed using whole arteries and veins, which contain multiple different cell types, and cultured and non-cultured cells have proposed that approximately twenty different TRP channels may be expressed in endothelial cells (Sullivan and Earley, 2013; Bulley et al., 2018). A significant body of work indicates that TRPV4 channels present in endothelial cells regulate the contractility of vasculature, including resistance-size arteries (Earley et al., 2009; Sonkusare et al., 2012; Zhang et al., 2009; Köhler et al., 2006; Marrelli et al., 2007). Evidence also suggests that endothelial cell TRPA1, TRPC3, TRPC4, TRPV1 and TRPV3 channels modulate vascular contractility (Liu et al., 2006; Gao et al., 2012; Freichel et al., 2001; Yang et al., 2010; Bratz et al., 2008; Earley et al., 2010; Sullivan et al., 2015). In many of these previous studies, TRP channel expression and or function was reported in endothelial cells of ex vivo vasculature that does not control systemic blood pressure, including conduit vessels, cerebral arteries, mammary arteries and umbilical vein (Earley and Brayden, 2015; Gao et al., 2012). Physiological functions of many TRP channels that are proposed to be expressed in endothelial cells are poorly understood, particularly in small resistance-size arteries that regulate regional organ blood flow and systemic blood pressure.
PKD2, which is also termed Transient Receptor Potential Polycystin 1 (TRPP1), PC-2 and polycystin-2, is encoded by the Pkd2 gene (Mochizuki et al., 1996). PKD2 contains six transmembrane domains, cytoplasmic N and C termini and a characteristic extracellular polycystin domain (Shen et al., 2016). PKD2 protein is expressed in a wide variety of different cell types, including endothelium, arterial smooth muscle, renal epithelia, cardiac myocytes and neurons, (Bulley et al., 2018; Semmo et al., 2014). Mutations in Pkd2 lead to Autosomal Dominant Polycystic Kidney Disease (ADPKD), the most prevalent monogenic human disease worldwide (Torres et al., 2007). ADPKD is typically characterized by the growth of renal cysts, although a significant proportion of patients develop hypertension prior to kidney dysfunction, suggesting PKD2 channels perform physiological functions in vascular wall cell types (Torres et al., 2007; Valero et al., 1999; Martinez-Vea et al., 2004). We have previously shown that intravascular pressure and α1-adrenoceptors activate PKD2 channels in arterial smooth muscle cells of different organs, leading to depolarization, vasoconstriction and an increase in systemic blood pressure (Bulley et al., 2018). In contrast, regulatory mechanisms and physiological functions of PKD2 channels in endothelial cells are unclear.
Here, we developed an inducible, cell-specific, knockout mouse model to study physiological functions of PKD2 channels in endothelial cells. We show that intravascular flow stimulates PKD2 channels in endothelial cells and that this mechanism is a major contributor to flow-mediated vasodilation over a broad shear stress range. In contrast, PKD2 channels do not contribute to ACh-induced dilation, suggesting stimulus-specific function. Flow-mediated PKD2 channel activation leads to Ca2+ influx, which activates SK and IK channels, and stimulates eNOS. These mechanisms induce arterial hyperpolarization, vasodilation and a reduction in blood pressure. Thus, PKD2 channels are a major contributor to functional flow-sensing in endothelial cells.
Results
Generation of tamoxifen-inducible, endothelial cell-specific PKD2 knockout mice
Mice with loxP sites flanking exons 11 and 13 (Pkd2fl/fl) of the Pkd2 gene were crossed with tamoxifen-inducible, endothelial cell-specific Cre (Cdh5-creERT2) mice, producing a Pkd2fl/fl:Cdh5-creERT2 line. Genomic PCR confirmed that tamoxifen stimulated Pkd2 recombination in mesenteric arteries of Pkd2fl/fl:Cdh5-creERT2 mice, but not in arteries of Pkd2fl/fl mice (Figure 1—figure supplement 1). Genomic PCR also amplified an identical product in tamoxifen-treated Pkd2fl/fl and Pkd2fl/fl:Cdh5-creERT2 mouse arteries due to Pkd2 in cells such as smooth muscle, where DNA would not undergo recombination (Figure 1—figure supplement 1; Bulley et al., 2018).
Western blotting was performed to quantify proteins in lysate collected from second- through fifth-order mesenteric artery branches. PKD2 protein in mesenteric arteries of tamoxifen-treated Pkd2fl/fl:Cdh5-creERT2 mice was ~ 67.2% of that in tamoxifen-treated Pkd2fl/fl controls (Figure 1A,B). This reduction in total arterial protein is expected given that smooth muscle cells, which also express PKD2, are far more abundant than endothelial cells in vessels of this size (Bulley et al., 2018). These data are also consistent with our previous observation that smooth muscle-specific PKD2 knockout reduced total mesenteric arterial wall PKD2 protein by ~ 75% (Bulley et al., 2018). In contrast, SK3, IK, TRPV4, Piezo1, GPR68 and PKD1 (polycystin-1, PC-1), which can form a complex with PKD2 (Qian et al., 1997; Tsiokas et al., 1997), were similar in arteries of both genotypes (Figure 1a and b). Immunofluorescence demonstrated that PKD2 protein was present in endothelial cells of intact arteries from tamoxifen-treated Pkd2fl/fl mice, but absent in endothelial cells of tamoxifen-treated Pkd2fl/fl:Cdh5-creERT2 mice (Figure 1C). These results indicate that PKD2 is expressed in endothelial cells and suggest that tamoxifen treatment of Pkd2fl/fl:Cdh5-creERT2 mice abolishes PKD2 protein. Tamoxifen-treated Pkd2fl/fl:Cdh5-creERT2 mice will thus be referred to as Pkd2 ecKO mice. Tamoxifen-treated Pkd2fl/fl mice were used as controls in all experiments.
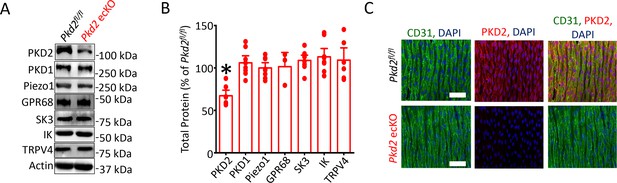
Generation and validation of Pkd2 ecKO mice.
(A) Representative Western blots illustrating the effect of tamoxifen-treatment of Pkd2fl/fl and Pkd2fl/fl: Cdh5(PAC)-creERT2 mice on PKD2, PKD1, Piezo1, GPR68, eNOS, SK3, IK and TPRV4, proteins in mesenteric arteries. (B) Mean data for proteins in mesenteric arteries of tamoxifen-treated Pkd2fl/fl: Cdh5(PAC)-creERT2 mice when compared to those in tamoxifen-treated Pkd2fl/fl mice. n = 3–8. * indicates p<0.05 versus Pkd2fl/fl. (C) En-face immunofluorescence imaging illustrating that PKD2 protein (Alexa Fluor 555) is abolished in endothelial cells of mesenteric arteries in tamoxifen-treated Pkd2fl/fl: Cdh5(PAC)-creERT2 mice (representative of 6 mesenteric arteries). CD31 (Alexa Fluor 488) and DAPI are also shown. Scale bars = 50 µm.
Endothelial cell PKD2 channels contribute to flow-, but not ACh-, mediated vasodilation
To investigate physiological functions of endothelial cell PKD2 channels, diameter responses to vasoactive stimuli were measured in pressurized (80 mmHg) third-order mesenteric arteries of Pkd2fl/fl and Pkd2 ecKO mice. Vasodilation to ACh, a muscarinic receptor agonist, was similar in control and Pkd2 ecKO arteries, suggesting that endothelial cell PKD2 channels do not contribute to this response (Figure 2A and C). Repetitive intravascular flow (15 dyn/cm2) stimuli produced sustained, reproducible and fully reversible vasodilation in pressurized (80 mmHg) mesenteric arteries (Figure 2—figure supplement 2A–D). In pressurized Pkd2 ecKO arteries, mean vasodilation to single on-off flow stimuli were ~35.1% of those in Pkd2fl/fl arteries (Figure 2B and C). Endothelial cell-denudation abolished vasodilation to both flow and ACh (Figure 2—figure supplement 1A and B). In contrast, endothelial denudation did not alter dilation to sodium nitroprusside, a NO donor, indicating that smooth muscle function was not altered by this procedure (Figure 2—figure supplement 1A and B). To determine the range over which endothelial cell PKD2 channels function, we measured vasoregulation to flow rates that produced shear stress between 3 and 35 dyn/cm2. Cumulative increases in flow caused progressive dilation in Pkd2fl/fl arteries, with a maximum at 27 dyn/cm2 (Figure 2D,E). Further increasing flow partially reduced this maximal vasodilatory response (Figure 2D and E). Flow stimulated less vasodilation in Pkd2 ecKO arteries over the range studied (Figure 2D,E; Figure 2—figure supplement 3). Specifically, flow-mediated vasodilation was between ~ 45.5% and 60.1% of that in Pkd2fl/fl arteries, regardless of rate (Figure 2D and E, Figure 2—figure supplement 3). These data indicate that endothelial cell PKD2 channels function over a broad flow range to stimulate vasodilation in pressurized arteries.
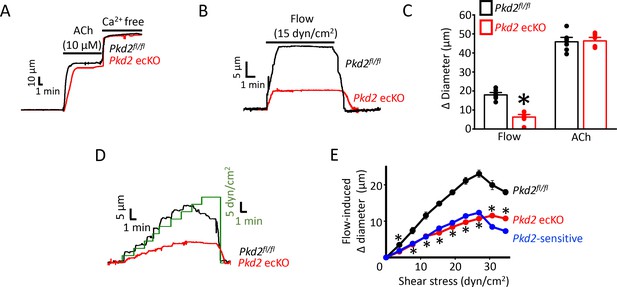
PKD2 channels contribute to intravascular flow-, but not ACh-, mediated vasodilation.
(A) Original traces illustrating responses to ACh (10 µM) and Ca2+-free solution (passive diameter) in pressurized (80 mmHg) mesenteric arteries from Pkd2fl/fl and Pkd2 ecKO mice. (B) Original trace of flow-mediated dilation in pressurized (80 mmHg) mesenteric arteries from Pkd2fl/fl and Pkd2 ecKO mice. (C) Mean diameter changes in response to flow (15 dyn/cm2) or ACh (10 µM). *p<0.05 vs. Pkd2fl/fl. n = 8 for each. # p<0.05 vs. flow in the same genotype. (D) Original traces illustrating diameter responses to stepwise increases in intravascular flow in pressurized (80 mmHg) mesenteric arteries from Pkd2fl/fl and Pkd2 ecKO mice. (E) Mean data. The Pkd2-sensitive component of flow-mediated vasodilation is illustrated in blue. *p<0.05 vs. Pkd2fl/fl. n = 5 for Pkd2fl/fl, n = 4 for Pkd2 ecKO.
Experiments were performed to examine the hypothesis that endothelial cell PKD2 channel knockout modifies smooth muscle cell contractility, thereby indirectly altering responses to flow. An increase in extracellular potassium (60 mm K+) or intravascular pressure (80 mmHg) similarly constricted arteries of Pkd2fl/fl and Pkd2 ecKO mice, indicating that endothelial cell PKD2 channels or their knockout does not influence depolarization-induced vasoconstriction or myogenic tone, respectively (Figure 2—figure supplement 4A–D). Similarly, arterial passive diameter, determined by removal of extracellular Ca2+ from the bath solution, was similar in Pkd2fl/fl and Pkd2 ecKO arteries (Figure 2—figure supplement 4E). Thus, knockout of endothelial cell PKD2 channels does not modify smooth muscle cell function.
Endothelial cell PKD2 channels contribute to flow-mediated arterial hyperpolarization
To investigate mechanisms by which endothelial cell PKD2 channels regulate contractility, membrane potential was measured in pressurized mesenteric arteries using glass microelectrodes. At 10 mmHg, the mean membrane potential of Pkd2fl/fl and Pkd2 ecKO arteries were similar at ~ −62.1 and −61.1 mV, respectively (Figure 3A,B). Increasing intravascular pressure to 80 mmHg similarly depolarized Pkd2fl/fl and Pkd2 ecKO arteries by ~ 19.3 and 17.7 mV, respectively (Figure 3A,B). Intravascular flow stimulated a mean hyperpolarization of ~ 11 mV in Pkd2fl/fl arteries (Figure 3A,B). In contrast, flow only hyperpolarized Pkd2 ecKO arteries by ~ 3 mV, or ~ 25.5% of that in controls (Figure 3A,B). These data suggest that flow modulates PKD2 channels in endothelial cells, leading to arterial hyperpolarization and vasodilation.
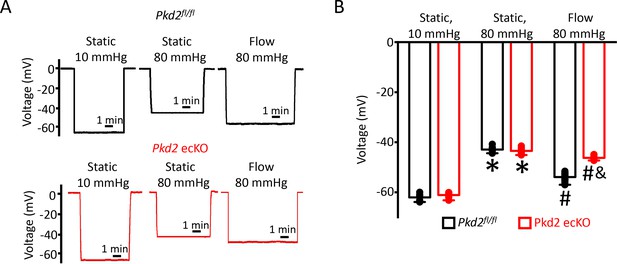
EC PKD2 channels contribute to flow-mediated arterial hyperpolarization.
(A) Original membrane potential recordings obtained from microelectrode impalements in pressurized mesenteric arteries of Pkd2fl/fl and Pkd2 ecKO mice at static (10 and 80 mmHg) and with 80 mmHg and flow (15 dyn/cm2). All three impalements in Pkd2fl/fl and Pkd2 ecKO were from the same two arteries. (B) Mean data (Pkd2fl/fl: 10 mmHg, n = 8; 80 mmHg, n = 10; 80 mmHg + flow, n = 18; Pkd2 ecKO: 10 mmHg, n = 7; 80 mmHg, n = 12; 80 mmHg + flow, n = 16). *p<0.05 for 80 mmHg static versus 10 mmHg static in same genotype. # p<0.05 for 80 mmHg + flow versus 80 mmHg static in the same genotype. and indicates p<0.05 versus Pkd2fl/fl under the same condition.
Flow activates a PKD2-mediated reduction in inward current in endothelial cells
The contribution of PKD2 channels to currents was investigated in mesenteric artery endothelial cells using patch-clamp electrophysiology. Temporal responses to flow were recorded using the whole-cell configuration with physiological ionic gradients and steady-state voltage of −60 mV. In a static bath, Pkd2fl/fl endothelial cells generated a mean steady-state inward current of ~−80 pA (Figure 4A,C). Flow stimulated an initial, transient peak increase in mean inward current of ~−21 pA that was followed by a sustained reduction in inward current that plateaued at ~−11 pA in Pkd2fl/fl cells (Figure 4-C). In the continuous presence of flow, the removal of bath Ca2+ increased mean inward current to ~−53 pA in Pkd2fl/fl cells (Figure 4A,C). In a static bath, mean steady-state inward current was similar in Pkd2fl/fl and Pkd2 ecKO cells (Figure 4A,C). In contrast, flow activated a transient peak inward current in Pkd2 ecKO cells that was only ~ 15% of that in Pkd2fl/fl cells (Figure 4A,B). Similarly, the sustained flow-mediated reduction in inward current in Pkd2 ecKO cells was ~ 40% of that in Pkd2fl/fl cells (Figure 4A,C). In the continuous presence of flow, removal of bath Ca2+ resulted alsoin a smaller increase in inward current in Pkd2 ecKO cells than in Pkd2fl/fl cells (Figure 4A,C). Specifically, under flow Ca2+ removal increased inward current only ~ 25 pA in Pkd2 ecKO cells, which was ~ 48.8% of the response in Pkd2fl/fl cells (Figure 4A,C). This differential response to Ca2+ removal in Pkd2fl/fl and Pkd2 ecKO endothelial cells was due to flow, as inward current in a static condition was similar in cells of both genotypes regardless of whether the bath solution contained Ca2+ or was Ca2+-free (Figure 4C). These data demonstrate that flow stimulates a biphasic current response that is composed of an initial transient inward current followed by a sustained Ca2+-dependent reduction in inward current in endothelial cells. Data also indicate that PKD2 channels contribute to both of these flow-mediated phases.
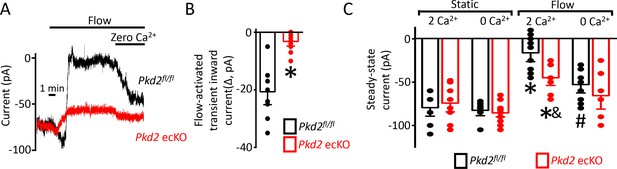
Flow reduces steady-state inward current through a PKD2-mediated, Ca2+ influx-dependent mechanism in voltage-clamped mesenteric artery endothelial cells.
(A) Original recordings of steady-state current modulation by flow (10 ml/min) and effect of removing bath Ca2+ at −60 mV in endothelial cells from Pkd2fl/fl and Pkd2 ecKO mice. (B) Mean data for flow-induced transient inward current. n = 9 for Pkd2fl/fl and n = 10 for Pkd2 ecKO. * indicates p<0.05 versus Pkd2fl/fl.(C) Mean data for steady-state currents in the presence and absence of flow and in the presence and absence of extracellular Ca2+ (Pkd2fl/fl: static + Ca2+, n = 9; static with zero Ca2+, n = 6; flow + Ca2+, n = 9; flow with zero Ca2+, n = 9 and Pkd2 ecKO: static + Ca2+, n = 9; static with zero Ca2+, n = 15; flow + Ca2+, n = 8; flow with zero Ca2+, n = 8). *p<0.05 versus static + Ca2+ conditions in the same genotype, and indicates p<0.05 vs Pkd2fl/fl under the same condition, # p<0.05 versus flow + Ca2+ in the same genotype.
PKD2-mediated Ca2+ influx activates SK/IK channels in endothelial cells
Attenuation of the flow-mediated sustained reduction in steady-state inward current by both PKD2 knockout and extracellular Ca2+ removal suggests the involvement of IK and SK channels. Under flow, the co-application of apamin and Tram-34, SK and IK channel blockers respectively, increased mean inward current by ~ 23.2 pA in Pkd2fl/fl cells (Figure 5A,B,D). In contrast, the apamin/tram-34-mediated increase in inward current under flow in Pkd2 ecKO cells was only ~ 11.6 pA or ~50% of that in Pkd2fl/fl cells (Figure 5A,B,D). In a static bath, apamin/Tram-34 produced a far smaller and similar increase in inward current in Pkd2fl/fl and Pkd2 ecKO cells (Figure 5C,D). These data suggest that flow stimulates PKD2-mediated Ca2+ influx that activates SK/IK channels in endothelial cells, leading to a steady-state reduction in inward current.
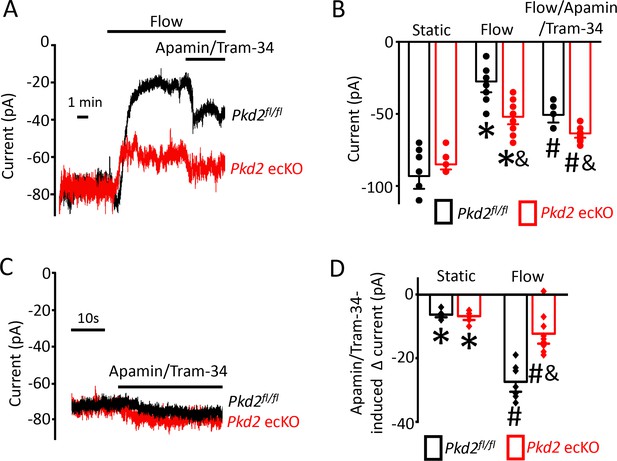
Flow-mediated PKD2 channel activation stimulates SK/IK channels in mesenteric artery endothelial cells, leading to vasodilation.
(A) Original recordings of steady-state current modulation by flow (10 ml/min) and flow plus apamin/Tram-34 (300 nM of each) at −60 mV in mesenteric artery ECs from Pkd2fl/fl and Pkd2 ecKO mice. (B) Mean data (Pkd2fl/fl: static, n = 8; flow, n = 8; flow + apamin/Tram-34, n = 7. Pkd2 ecKO: static, n = 9, flow, n = 10; flow + apamin/Tram-34, n = 9). * indicates p<0.05 versus static in the same genotype and p<0.05 vs Pkd2fl/fl in the same conditions. # p<0.05 versus flow in the same genotype. (C) Original recordings of steady-state current modulation by apamin/Tram-34 (300 nM of each) in the absence of flow at −60 mV in ECs from Pkd2fl/fl and Pkd2 ecKO mice. (D) Mean data comparing responses to apamin/Tram-34 in static and flow conditions at −60 mV (Pkd2fl/fl: static, n = 6; flow, n = 7. Pkd2 ecKO: static, n = 6; flow, n = 9). *p<0.05 versus static control. # p<0.05 versus static + apamin/Tram-34 in the same genotype. and indicates p<0.05 for Pkd2 ecKO vs Pkd2fl/fl in the same condition.
Next, we tested the hypothesis that flow-stimulates vasodilation through PKD2-mediated SK/IK channel activation. Apamin/Tram-34 reduced both flow- and ACh -induced vasodilation in Pkd2fl/fl arteries to ~ 77% and 57% of those that occurred in the control condition (Figure 6A,C). Apamin/Tram-34 reduced mean ACh-induced vasodilation to ~ 54% of that in control in Pkd2 ecKO arteries, which was a similar reduction to that in Pkd2fl/fl arteries (Figure 6A,C). In contrast, apamin/Tram-34 did not alter flow-mediated vasodilation in Pkd2 ecKO arteries (Figure 6A,C). With static intravascular solution, bath application of apamin/Tram-34 did not alter the diameter of pressurized, myogenic Pkd2fl/fl or Pkd2 ecKO mesenteric arteries, indicating that SK and IK channels are not active in the absence of flow or ACh (Figure 6B,C). These data indicate that flow stimulates PKD2-mediated SK/IK channel activation in endothelial cells to induce vasodilation. In contrast, ACh stimulates vasodilation via a PKD2-independent SK/IK channel-mediated mechanism.
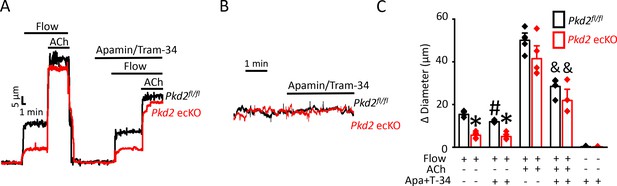
PKD2 channels contribute to intravascular flow-mediated SK/IK channel activation and vasodilation.
(A) Representative traces illustrating responses to flow (15 dyn/cm2) and flow (15 dyn/cm2) + ACh (10 µM) in the presence and absence of apamin/Tram-34 (300 nM of each) in pressurized (80 mmHg) mesenteric arteries from Pkd2fl/fl and Pkd2 ecKO mice. (B) Representative traces illustrating responses to apamin/Tram-34 (300 nM of each) in the absence of intravascular flow in pressurized (80 mmHg) mesenteric arteries. (C) Mean data (Pkd2fl/fl: flow, n = 5; flow + apamin/Tram-34, n = 5; flow + ACh (10 µM), n = 5; flow + ACh (10 µM) + apamin/Tram-34, n = 5; static + apamin/Tram-34, n = 5. Pkd2 ecKO: flow, n = 5; flow + apamin/Tram-34, n = 5; flow + ACh (10 µM), n = 5; flow + ACh (10 µM) + apamin/Tram-34, n = 4; static + apamin/Tram-34, n = 5). * indicates p<0.05 versus Pkd2fl/fl in the same condition. # indicates p<0.05 for flow + apamin/Tram-34 versus flow in the same genotype. and indicates p<0.05 for flow + ACh versus flow + ACh + apamin/Tram-34 in the same genotype.
PKD2 channel activation is essential for flow-mediated eNOS activation in endothelial cells
Flow stimulates nitric oxide synthase (NOS) in endothelial cells, but the significance of PKD2 channels to this activation mechanism is unclear (Fleming, 2010; Balligand et al., 2009; Garcia and Sessa, 2019). Phosphorylation of bovine eNOS at serine 1179 and human eNOS at serine 1177 leads to activation (Fulton et al., 1999; Dimmeler et al., 1999). Western blotting was performed to measure both eNOS protein phosphorylated at serine 1176 (p-eNOS (S1176)) and total eNOS protein in mouse mesenteric arteries. Intravascular flow (15 dyn/cm2, 5 min, 37°C) increased mean p-eNOS (S1176) protein ~ 1.4 fold in Pkd2fl/fl arteries, but only ~ 1.08 fold in Pkd2 ecKO arteries (Figure 7A,B). In contrast, flow did not alter total eNOS in either genotype (Figure 7A,B). L-NNA, a NOS inhibitor, reduced flow-mediated vasodilation to ~ 64% of control in pressurized Pkd2fl/fl arteries and to ~ 83% of control in Pkd2 ecKO arteries (Figure 7C,D). Thus, the L-NNA-induced reduction in flow-mediated vasodilation in Pkd2 ecKO arteries was ~ 47% of that in Pkd2fl/fl arteries (Figure 7C,D). These data indicate that PKD2 channels are key for flow to activate eNOS in endothelial cells and to elicit vasodilation through this mechanism in mesenteric arteries.
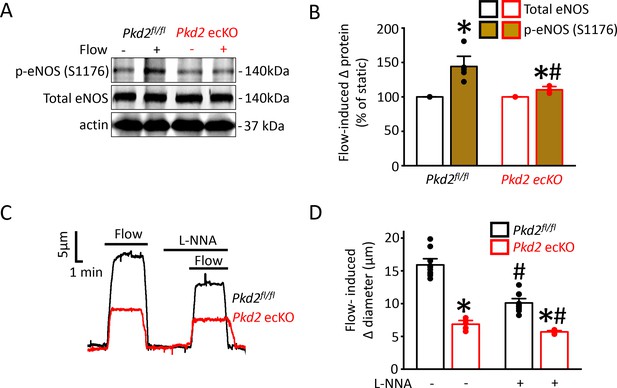
Flow-mediated PKD2 channel activation in ECs stimulates eNOS serine 1176 phosphorylation, leading to vasodilation.
(A) Original Western blots illustrating effects of flow (15 dyn/cm2) and Pkd2 ecKO on p-eNOS (S1176) and total eNOS proteins in Pkd2fl/fl and Pkd2 ecKO mesenteric arteries. (B) Mean data for flow-induced change (Δ) in proteins. n = 5 for Pkd2fl/fl. n = 4 for Pkd2 ecKO. * indicates p<0.05 versus static. # indicates p<0.05 versus same protein in Pkd2fl/fl. (C) Representative traces demonstrating flow (15 dyn/cm2)-mediated vasodilation in pressurized (80 mmHg) mesenteric arteries of Pkd2fl/fl and Pkd2 ecKO mice in the presence and absence of L-NNA (10 µM). (D) Mean data. n = 10 for Pkd2fl/fl. n = 5 for Pkd2 ecKO. * indicates p<0.05 versus Pkd2fl/fl in the same condition. # indicates p<0.05 versus flow in the absence of L-NNA (10 µM) in the same genotype.
Pkd2 ecKO mice are hypertensive
In vitro evidence that endothelial cell PKD2 channels contribute to flow-mediated vasodilation suggests that these proteins may regulate blood pressure. Telemetry measurements were performed using implanted probes to measure systemic blood pressure in Pkd2fl/fl and Pkd2 ecKO mice. Diastolic and systolic blood pressures were ~ 9 and 14 mmHg higher, respectively, in Pkd2 ecKO than Pkd2fl/fl mice, which translated to a mean arterial pressure (MAP) that was raised by ~ 11% (Figure 8A,B). Locomotion was similar between genotypes, indicating that the higher blood pressure in Pkd2 ecKO mice was not due to higher activity (Figure 8—figure supplement 1). Echocardiography measurements indicated that cardiac output, fractional shortening, ejection fraction and heart rate were all similar in Pkd2fl/fl and Pkd2 ecKO mice (Figure 8C–F). Proximal tubule diameter and glomerular area were also similar in kidneys of Pkd2fl/fl and Pkd2 ecKO mice, indicating no renal dysfunction (Figure 8G–I). These results demonstrate that flow stimulates PKD2 channels in endothelial cells to induce vasodilation and reduce systemic blood pressure.
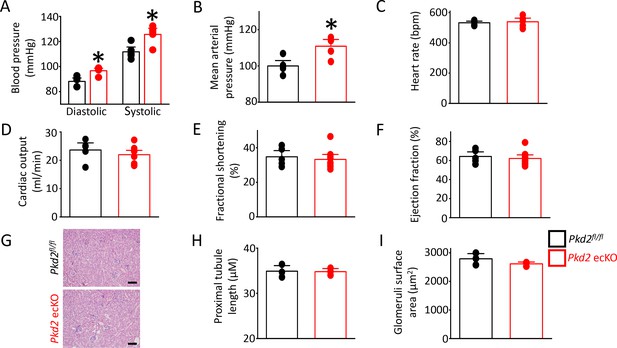
Pkd2 ecKO elevates systemic blood pressure, but does not alter cardiac function or kidney histology.
(A) Mean diastolic and systolic blood pressures in Pkd2fl/fl and Pkd2 ecKO mice (n = 5 for each). * indicates p<0.05 versus Pkd2fl/fl. (B) Mean arterial blood pressure (MAP) (n = 5 for each). * indicates p<0.05 versus Pkd2fl/fl. (C–F) Mean echocardiography data. Heart rate (HR), Cardiac output (CO), fractional shortening (FS) and ejection fraction (EF) (n = 5 Pkd2fl/fl and n = 10 for Pkd2 ecKO). (G) Representative images of H and E stained kidney cortex used for histological assessment. Scale bars = 100 µm. (H) Mean proximal tubule length (n = 15 proximal tubules measured for each group from three individual mice). (I) Mean glomeruli surface area (n = 75 glomeruli measured per group from three individual mice).
Discussion
Here, we investigated mechanisms of regulation and physiological functions of PKD2 channels in endothelial cells by using an inducible, conditional knockout mouse model. Endothelial cell PKD2 knockout robustly inhibits flow-mediated vasodilation, but does not alter dilation to ACh, in resistance-size arteries, suggesting stimulus-specific signaling and function. Flow stimulates PKD2 channels, leading to both Ca2+ influx-dependent SK/IK channel activation and eNOS phosphorylation and activation in endothelial cells (Figure 9). These mechanisms induce arterial hyperpolarization and vasodilation. Endothelial cell PKD2 channel knockout increased both diastolic and systolic blood pressure in mice, without effects on cardiac function or kidney anatomy. Thus, by coupling intravascular flow to vasodilation, endothelial cell PKD2 channels reduce blood pressure.
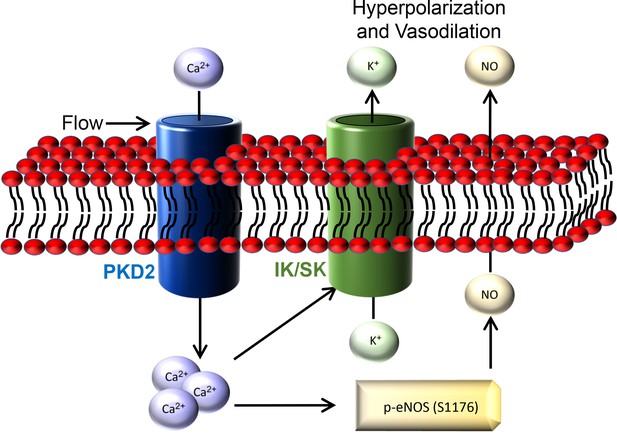
Schematic illustration of the mechanisms by which endothelial cell PKD2 channels elicit flow-mediated vasodilation.
Global knockout of a gene can lead to compensatory expression of other genes that produce contrasting and contradictory results to those expected from studies on isolated cells and tissues. Whether endothelial cell TRP channels are functional could not be determined from global TRPC6, TRPM4 and TRPV4 channel knockout mice, which generated complex findings associated with compensatory mechanisms (Earley et al., 2009; Mathar et al., 2010; Dietrich et al., 2005; Nishijima et al., 2014). Global knockout of TRPM4, which is expressed in multiple cell types, including arterial smooth muscle, increased catecholamine secretion that elevated blood pressure in mice (Mathar et al., 2010; Earley et al., 2004). TRPC6 knockout resulted in upregulation of constitutively active TRPC3 channels in arterial smooth muscle cells that caused vasoconstriction and elevated blood pressure (Dietrich et al., 2005). Global knockout of TRPV4 channels, which are expressed in both arterial smooth muscle cells and endothelial cells, resulted in either the same or lower blood pressure than controls (Earley et al., 2009; Nishijima et al., 2014). Here, inducible, endothelial cell-specific PKD2 knockout did not alter the expression of PKD1, Piezo1, GPR68, SK3, IK or TRPV4 channels in mesenteric arteries. Flow stimulated plasma membrane Ca2+ influx that activated SK/IK channels, producing a steady-state reduction in inward current in Pkd2fl/fl mouse endothelial cells. PKD2 knockout reduced both the flow-mediated transient inward current and the steady-state reduction in inward current that occurred, in part, due to Ca2+-dependent SK/IK channel activation. Flow elevated intracellular Ca2+ concentration and activated eNOS in a Ca2+/calmodulin–dependent manner (Fleming, 2010; Michel and Vanhoutte, 2010; Zhou et al., 2014). The ion channel(s) responsible for these Ca2+-dependent signaling mechanisms were unclear. Here, we show that PKD2 channels are essential to both flow-mediated Ca2+ influx that activates SK/IK channels and to eNOS activation. PKD2 channel properties have been debated for almost two decades, particularly their ionic permeability. Recent evidence suggests that PKD2 homotetramers are voltage-dependent, outwardly rectifying and primarily permeant to Na+ and K+, with low Ca2+ permeability (Shen et al., 2016; Wang et al., 2019). PKD1 and PKD2 in a 1 to 3 ratio, respectively, can also form a heterotetrameric channel that is far more permeant to Ca2+ than PKD2 homotetramers (Wang et al., 2019; Su et al., 2018; Zhu et al., 2011; Yu et al., 2009). Whether flow stimulates PKD2 homotetramers and/or a PKD1/PKD2 heterotetramers to generate the Ca2+ signal that activates SK/IK channels remains to be established. PKD2 has also been proposed to interact with TRPC1, TRPC3, TRPC5, TRPC7 and TRPV4 channels, but whether heterotetrameric channel formation occurs between these different proteins in native endothelial cells is poorly understood (Miyagi et al., 2009; Tsiokas et al., 1999; Köttgen et al., 2008; Sutton et al., 2006; Du et al., 2014; Du et al., 2008). Future studies should test these hypotheses.
Increasing intravascular flow produced progressive vasodilation, with the relative contribution of endothelial cell PKD2 channels to this response approximately 50%, regardless of the magnitude of shear stress. These results indicate that PKD2 channel activity is flow-dependent and show that PKD2 channels function over a broad range of shear stress to elicit vasodilation. PKD2 channels do not appear to be inherently flow-sensitive. Potential mechanisms by which flow stimulates PKD2 channels include coupling to PKD1, regulation by microtubules and/or the actin cytoskeleton, and through interaction with TRPV4 and TRPC1 (Du et al., 2014; Li et al., 2006; Hardy and Tsiokas, 2020; Nauli et al., 2003). PKD2 channel knockout did not abolish flow-mediated current modulation in endothelial cells or vasodilation in pressurized arteries, suggesting that PKD2 channel-independent mechanisms also contribute to these responses. A previous study demonstrated that flow activates Piezo1 channels in endothelial cells, leading to vasodilation and a reduction in blood pressure (Wang et al., 2016). In contrast, another study published that endothelial cell Piezo1 does not regulate blood pressure during inactivity, but is activated by an increase in flow during exercise, resulting in arterial depolarization and vasoconstriction (Rode et al., 2017). Global knockout of GPR68, a class A rhodopsin-like G protein-coupled receptor, reduced flow-mediated vasodilation in third-order mesenteric arteries, but did not alter effects of flow in first- or second-order mesenteric arteries where it is not expressed (Xu et al., 2018). Other proposed flow-mediated mechanisms include those via TRPV4, angiotensin type II, histamine, and bradykinin type two receptors, although recombinant expression of these proteins has also been shown to not produce flow-mediated responses (Zhang et al., 2009; Chachisvilis et al., 2006; Mendoza et al., 2010; Ramkhelawon et al., 2013). Signaling mechanism described include the release of ATP, which binds in an autocrine manner to purinergic receptors, adrenomedullin, acting via cell surface receptors containing CALCRL, and ACh that is released by organic cation transporters and activates muscarinic receptors (Wang et al., 2016; Iring et al., 2019; Wilson et al., 2016). These pathways can also involve eNOS activation (Wang et al., 2016; Iring et al., 2019). Which of these mechanisms are PKD2-dependent and which are PKD2-independent remains to be established.
Endothelial cell Pkd2 knockout attenuated flow-mediated vasodilation, but did not alter vasodilation to ACh, indicating differential signaling mechanisms. Intracellular signals and kinases that regulate PKD2 channels in endothelial cells are poorly understood. As such, it is not clear whether signaling mechanisms activated by muscarinic receptors are incapable of activating PKD2 channels. Muscarinic receptor agonists activate TRPV4 channels, leading to Ca2+ influx, and promote endoplasmic reticulum Ca2+ release, both of which can stimulate SK and IK channels and eNOS to produce vasodilation (Sonkusare et al., 2012; Edwards et al., 2010; Fleming and Busse, 1999). The relative proportion of each of these pathways to muscarinic receptor-mediated signaling differs depending on the arterial bed that is studied. Here, we show that PKD2 channel activation also stimulates IK/SK channels and eNOS. Thus IK/SK and eNOS are common downstream targets for both TRPV4 and PKD2 channels. A reasonable explanation for differential signaling elicited by flow and muscarinic receptors is that flow activates PKD2 channels in endothelial cells via a compartmentalized mechanism that excludes signaling from muscarinic receptors. Homozygous knockout of Pkd2 is lethal in mice, precluding study of the global absence of this gene product on vascular function. ACh-induced vasodilation was attenuated due to a decrease in the availability of nitric oxide in mesenteric arteries of Pkd2 heterozygous (Pkd2+/-) mice aged between 16 and 20 weeks (Brookes et al., 2013). This result is in marked contrast to observations we made here where ACh-induced vasodilation was similar in mesenteric arteries of Pkd2fl/fl and Pkd2 ecKO mice. These different observations likely reflect the effects of studying short-term, endothelial cell specific PKD2 knockout versus a global and prolonged reduction in PKD2 protein that was present since gestation.
Autosomal Dominant Polycystic Kidney Disease (ADPKD) occurs due to mutations in Pkd1 or Pkd2 and is the most prevalent monogenic human disease worldwide, affecting 1 in 400–1000 individuals (Torres et al., 2007). More than 275 variants in human Pkd2 have been identified (http://pkdb.pkdcure.org). Although ADPKD is characterized by the appearance of renal cysts, patients can develop hypertension prior to any kidney dysfunction (Torres et al., 2007; Valero et al., 1999; Martinez-Vea et al., 2004). Here, short-term endothelial cell PKD2 knockout increased systemic blood pressure without inducing cardiac or renal abnormalities. These data suggest that ADPKD patients may develop hypertension due to dysfunctional endothelial cell PKD2 channels and attenuated flow-mediated vasodilation. During an increase in sustained blood flow, human ADPKD patients display loss of nitric oxide release and an associated reduction in endothelium-dependent dilation in conduit arteries, consistent with the results obtained in our mouse model (Lorthioir et al., 2015) As the polycystin mutation was global in these human subjects, it was not clear if the vascular deficiency was due to dysfunctional signaling in endothelial cells or another cell type that regulates endothelial cell function. Prolonged polycystin dysregulation in ADPKD patients may alter responses to a wide variety of other stimuli that were not studied. Future studies should investigate the effects of ADPKD-associated Pkd2 mutations on endothelial cell function, arterial contractility and systemic blood pressure. Our demonstration that endothelial cell PKD2 channels contribute to flow-mediated vasodilation and reduce blood pressure is a step forward in understanding the physiological significance of this protein and its dysfunction in patients with ADPKD and other cardiovascular diseases.
In summary, using an inducible, conditional Pkd2 knockout mouse, we demonstrate that intravascular flow stimulates PKD2 channels in endothelial cells, leading to Ca2+-dependent SK/IK channel and eNOS activation, arterial hyperpolarization, vasodilation and a reduction in systemic blood pressure. These results indicate that endothelial cell PKD2 channels are a major mechanistic component of functional flow-sensing in the vasculature.
Materials and methods
Reagent type (species) or resource | Designation | Source or reference | Identifiers | Additional information |
---|---|---|---|---|
Strain, strain background (M. musculus) | Pkd2fl/fl | Baltimore PKD Core Center | PMID:20862291 | Mice with Pkd2 gene flanked by loxP regions. |
Strain, strain background (M. musculus) | Cdh5(PAC)-creERT2 | Cancer Research UK | RRID:MGI:3848984 | Mice with tamoxifen-inducible Cre recombinase that is expressed specifically in endothelial cells. |
Strain, strain background (M. musculus) | Pkd2fl/fl: Cdh5(PAC)-creERT2 | This paper | Mouse line created in-house by mating Pkd2fl/fl with Cdh5(PAC)-creERT2. Mice with inducible endothelial cell-specific deletion of PKD2. | |
Antibody | Anti-PKD2 (rabbit polyclonal) | Baltimore PKD Core | Rabbit mAB 3374 CT-14/4 | IF 1:200 dilution |
Antibody | Anti-PKD2 (mouse monoclonal) | Santa Cruz | Cat. # sc-100415 RRID:AB_1127284 | WB 1:100 dilution |
Antibody | Anti-PKD1 (mouse monoclonal) | Santa Cruz | Cat. # sc-130554 RRID:AB_2163355 | WB 1:100 dilution |
Antibody | Anti-Piezo1 (rabbit polyclonal) | Proteintech | Cat.# 15939–1-AP. | WB 1:100 dilution |
Antibody | Anti-SK3 antibody | Abcam | Cat. # ab28631 RRID:AB_775888 | WB 1:100 dilution |
Antibody | Anti-IK1 Antibody (D-5) (mouse monoclonal) | Santa Cruz | Cat. # sc-365265 RRID:AB_10841432 | WB 1:100 dilution |
Antibody | Anti-eNOS (mouse monoclonal) | Abcam | Cat. # ab76198 RRID:AB_1310183 | WB 1:100 dilution |
Antibody | Anti-p-eNOS (rabbit monoclonal) | Cell signaling Technology | Cat. # 9571 RRID:AB_329837 | WB 1:100 |
Antibody | Anti-GPR68 | NOVUS Biologicals | Cat. # NBP2-32747 | WB 1:100 |
Antibody | Anti-TRPV4 (clone 1B2.6) (mouse monoclonal) | Millipore Sigma | Cat. # MABS466 | WB 1:100 |
Antibody | Anti-Actin (mouse monoclonal) | Millipore Sigma | Cat. # MAB1501 RRID:AB_2223041 | WB 1:5000 dilution |
Antibody | Alexa 555 secondary antibodies (anti rabbit and anti mouse) | Thermo Fisher | Cat. # A-21429 (RRID:AB_141761) and # A-31570 (RRID:AB_2536180) | IF 1:400 dilution |
Antibody | Alexa 488 secondary antibodies (anti rat) | Thermo Fisher | Cat. # A-21470 RRID:AB_2535873 | IF 1in 400 dilution |
Other | Nuclear staining (DAPI) | Thermo Fisher | Cat. # 3571 RRID:AB_2307445 | IF 1:1000 dilution |
Animals
All procedures were approved by the Animal Care and Use Committee of the University of Tennessee (protocol 17–068.0). Pkd2fl/fl mice were obtained from the Baltimore PKD Core Center. Cdh5(PAC)-creERT2 mice were a kind gift from Cancer Research UK (Wang et al., 2010). Pkd2fl/fl mice with loxP sites flanking exons 11–13 of the Pkd2 gene were obtained from the John Hopkins PKD Core. Pkd2fl/fl mice were crossed with tamoxifen-inducible endothelial cell-specific Cre mice (Cdh5(PAC)-CreERT2, Cancer Research UK) to generate PKD2fl/fl:Cdh5(PAC)-CreERT2 mice. Male Pkd2fl/fl:Cdh5(PAC)-CreERT2 or Pkd2fl/fl mice (8–14 weeks of age) were injected with tamoxifen (1 mg/ml, i.p.) once per day for 5 days and studied 7–14 days after the last injection.
Tissue preparation and endothelial cell isolation
Request a detailed protocolMale mice were euthanized with isoflurane (1.5%), followed by decapitation. Mesenteric artery branches from first- to fifth-order were removed, cleaned of adventitial tissue and placed into ice-cold physiological saline solution (PSS) that contained (in mM): 112 NaCl, 6 KCl, 24 NaHCO3, 1.8 CaCl2, 1.2 MgSO4, 1.2 KH2PO4 and 10 glucose, gassed with 21% O2, 5% CO2 and 74% N2 to pH 7.4. Endothelial cells were dissociated by introducing endothelial cell basal media (Endothelial cell GM MV2, Promocell) containing 2 mg/ml collagenase type 1 (Worthington Biochemical) into the arterial lumen and left to incubate for 30–40 min at 37°C. Cells isolated from mesenteric arteries contain multiple different types that exhibit similar visual phenotypes upon enzymatic isolation. To obtain a population of endothelial cells, cell isolate was placed into endothelial cell basal media containing growth supplements (Promocell) that support only endothelial cell survival. Endothelial cells were then studied < 5 days later.
Genomic PCR
Request a detailed protocolGenomic DNA was isolated from mesenteric arteries using a Purelink Genomic DNA kit (Thermo Fisher Scientific). Reaction conditions used are outlined in the Baltimore PKD Center genotyping protocol (http://baltimorepkdcenter.org/mouse/PCR%20Protocol%20for%20Genotyping%20PKD2KO%20and%20PKD2%5Eneo.pdf). Genotyping was performed using a 3-primer strategy, with primers a (5’-CCTTTCCTCTGGTTCTGGGGAG), b (5’-GTTGATGCTTAGCAGATGATGGC) and c (5’-CTGACAGGCACCTACAGAACAGTG) used to identify floxed and deleted alleles.
Western blotting
Request a detailed protocolMesenteric artery segments comprising second- to fifth-order vessels were used for Western blotting. For experiments examining flow-mediated regulation of NOS and p-eNOS proteins, a glass cannula was inserted into the first-order branch of a mesenteric artery segment and flow introduced through to fifth-order arteries. Proteins were separated on 7.5% SDS-polyacrylamide gels and blotted onto nitrocellulose membranes. Membranes were blocked with 5% milk and incubated with one of the following primary antibodies: Piezo1 (Proteintech), PKD1 (Santa Cruz), PKD2 (Santa Cruz), SK3 (Abcam), eNOS (Abcam), IK (Alomone), p-eNOS (Cell Signaling), GPR68 (NOVUS), TRPV4 (MilliporeSigma) or actin (MilliporeSigma) overnight at 4°C. Membranes were washed and incubated with horseradish peroxidase-conjugated secondary antibodies at room temperature. Protein bands were imaged using a ChemiDoc Touch Imaging System (Bio-Rad), quantified using ImageJ software and normalized to actin.
En-face arterial immunofluorescence
Request a detailed protocolArteries were cut longitudinally and fixed with 4% paraformaldehyde in PBS for 1 hr. Following a wash in PBS, arteries were permeabilized with 0.2% Triton X-100, blocked with 5% goat serum and incubated overnight with PKD2 primary antibody (Rabbit mAB 3374 CT-14/4: Baltimore PKD Center) at 4°C. Arteries were then incubated with Alexa Fluor 555 rabbit anti-mouse secondary antibody (1:400; Molecular Probes) and 4’,6-diamidino-2-phenylindole, dihydrochloride (DAPI) (1:1000; Thermo Scientific) for 1 hr at room temperature. Arteries were washed with PBS and mounted in 80% glycerol solution. DAPI and Alexa 555 were excited at 350 nm and 555 nm with emission collected at ≤ 437 nm and ≥ 555 nm, respectively, using a Zeiss LSM 710 laser-scanning confocal microscope.
Pressurized artery myography
Request a detailed protocolExperiments were performed using isolated third- and fourth-order mesenteric arteries using PSS gassed with 21% O2/5% CO2/74% N2 (pH 7.4). Arterial segments 1–2 mm in length were cannulated at each end in a perfusion chamber (Living Systems Instrumentation) continuously perfused with PSS and maintained at 37°C. Intravascular pressure was altered using a Servo pump model PS-200-P (Living systems) and monitored using pressure transducers. Following development of stable myogenic tone, luminal flow was introduced during experiments using a P720 peristaltic pump (Instech). Arterial diameter was measured at 1 Hz using a CCD camera attached to a Nikon TS100-F microscope and the automatic edge-detection function of IonWizard software (Ionoptix). Myogenic tone was calculated as: 100 x (1-Dactive/Dpassive) where Dactive is active arterial diameter and Dpassive is the diameter determined in the presence of Ca2+-free PSS supplemented with 5 mM EGTA.
Pressurized artery membrane potential measurements
Request a detailed protocolMembrane potential was measured by inserting sharp glass microelectrodes (50–90 MΩ) filled with 3 M KCl into the adventitial side of pressurized third- and fourth-order mesenteric arteries. Membrane potential was recorded using a WPI FD223a amplifier and digitized using a MiniDigi 1A USB interface, pClamp 9.2 software (Axon Instruments) and a personal computer. Criteria for successful intracellular impalements were: (Vane, 1994) a sharp negative deflection in potential on insertion; (Edwards et al., 1998) stable voltage for at least 1 min after entry; (Leffler et al., 2006) a sharp positive voltage deflection on exit from the recorded cell and (Garland et al., 2011) a < 10% change in tip resistance after the impalement.
Patch-clamp electrophysiology
Request a detailed protocolThe conventional whole-cell configuration was used to measure steady-state currents in isolated endothelial cells at a holding potential of −60 mV. The bath solution contained (in mM): NaCl 134, KCl 6, HEPES 10, MgCl2 1, CaCl2 2 and glucose 10 (pH 7.4, NaOH). Ca2+-free bath solution was the same composition as bath solution except Ca2+ was omitted and 1 mM EGTA added. The pipette solution contained (in mM): K aspartate 110, KCl 30, HEPES 10, glucose 10 and EGTA 1, with total MgCl2 and CaCl2 adjusted to give free concentrations of 1 mM and 200 nM, respectively. Free Mg2+ and Ca2+ were calculated using WebmaxC Standard (http://www.stanford.edu/~cpatton/webmaxcS.htm). The osmolarity of solutions was measured using a Wescor 5500 Vapor Pressure Osmometer (Logan, UT, USA). Currents were filtered at 1 kHz and digitized at 5 kHz using an Axopatch 200B amplifier and Clampex 10.4 (Molecular Devices. Offline analysis was performed using Clampfit 10.4. Flow-activated transient inward current was measured at its peak in each cell. Steady-state inward currents were calculated as the average of at least 45 s of continuous data.
Telemetric blood pressure and locomotion measurements
Request a detailed protocolTelemetric blood pressure recordings were performed by the University of Cincinnati Mouse Metabolic Phenotyping Center. Briefly, transmitters (PA-C10, Data Sciences International) were implanted subcutaneously into anesthetized mice, with the sensing electrode placed in the aorta via the left carotid artery. Blood pressures were measured prior, during and following tamoxifen injections (1 mg/ml, i.p) using a PhysioTel Digital telemetry platform (Data Sciences International). Dataquest A.R.T. software was used to acquire and analyze data.
Echocardiography
Request a detailed protocolAge- and sex-matched mice were anesthetized with isoflurane and placed on a warm pad on a recording stage of a Vevo 2100 ultrasound machine. The anterior chest was shaved and ultrasound coupling gel applied. Electrodes were connected to each limb and an electrocardiogram was recorded. Two-dimensional (short axis-guided) M-mode measurements were taken at the level of the papillary muscles using an 18–32 MHz MS400 transducer, as previously described (Parks et al., 2016). Images were also recorded in the parasternal long-axis. For analysis purposes, three or more beats were averaged using measurements within the same HR interval (450 ± 50 bpm) for analysis.
Kidney histology
Request a detailed protocolKidney sections were stained with H and E and examined by Probetex, Inc (San Antonio, Texas). Briefly, image analysis was performed to measure glomerular size and tubular cross-sectional diameter. Glomerular size was measured by tracing the circumference of each of 25 random glomeruli and surface area calculated using the polygonal area tool of Image-Pro 4.5 image analysis software calibrated to a stage micrometer. Tubular size was measured using the linear length tool of Image-Pro 4.5 imaging software. The tracing tool was applied at the diameter of cross-sectional profiles of 5 proximal tubules/image (total of 25/section). Glomerular and tubular images were calibrated to a stage micrometer and data was transferred to an Excel spreadsheet and statistical analysis performed by Excel analysis pack.
Statistical analysis
Request a detailed protocolOriginLab and GraphPad InStat software were used for statistical analyses. Values are expressed as mean ± SEM. Student t-test was used for comparing paired and unpaired data from two populations and ANOVA with Holm-Sidak post hoc test used for multiple group comparisons. p<0.05 was considered significant. Power analysis was performed to verify that the sample size gave a value of > 0.8 if P was > 0.05. Kidney histology, blood pressure and cardiac function experiments were all done single blind, wherein the person performing both the experiments and analysis of the results was not aware of the mouse genotype.
Data availability
All data generated or analysed during this study are included in the manuscript and supporting files.
References
-
Impaired capsaicin-induced relaxation of coronary arteries in a porcine model of the metabolic syndromeAmerican Journal of Physiology-Heart and Circulatory Physiology 294:H2489–H2496.https://doi.org/10.1152/ajpheart.01191.2007
-
Pkd2 mesenteric vessels exhibit a primary defect in endothelium-dependent vasodilatation restored by rosiglitazoneAmerican Journal of Physiology-Heart and Circulatory Physiology 304:H33–H41.https://doi.org/10.1152/ajpheart.01102.2011
-
Increased vascular smooth muscle contractility in TRPC6-/- miceMolecular and Cellular Biology 25:6980–6989.https://doi.org/10.1128/MCB.25.16.6980-6989.2005
-
Mediation of angiotensin II-induced Ca2+ signaling by polycystin 2 in glomerular mesangial cellsAmerican Journal of Physiology. Renal Physiology 294:F909–F918.https://doi.org/10.1152/ajprenal.00606.2007
-
TRPV4, TRPC1, and TRPP2 assemble to form a flow-sensitive heteromeric channelThe FASEB Journal 28:4677–4685.https://doi.org/10.1096/fj.14-251652
-
TRPV4-dependent dilation of peripheral resistance arteries influences arterial pressureAmerican Journal of Physiology-Heart and Circulatory Physiology 297:H1096–H1102.https://doi.org/10.1152/ajpheart.00241.2009
-
Transient receptor potential channels in the vasculaturePhysiological Reviews 95:645–690.https://doi.org/10.1152/physrev.00026.2014
-
Endothelium-derived hyperpolarising factors and associated pathways: a synopsisPflügers Archiv - European Journal of Physiology 459:863–879.https://doi.org/10.1007/s00424-010-0817-1
-
Molecular mechanisms underlying the activation of eNOSPflügers Archiv - European Journal of Physiology 459:793–806.https://doi.org/10.1007/s00424-009-0767-7
-
NO: the primary EDRFJournal of Molecular and Cellular Cardiology 31:5–14.https://doi.org/10.1006/jmcc.1998.0839
-
Role of TRPC3 channel in human internal mammary arteryArchives of Medical Research 43:431–437.https://doi.org/10.1016/j.arcmed.2012.08.010
-
Endothelial NOS: perspective and recent developmentsBritish Journal of Pharmacology 176:189–196.https://doi.org/10.1111/bph.14522
-
EDHF: spreading the influence of the endotheliumBritish Journal of Pharmacology 164:839–852.https://doi.org/10.1111/j.1476-5381.2010.01148.x
-
Shear stress-induced endothelial adrenomedullin signaling regulates vascular tone and blood pressureJournal of Clinical Investigation 129:2775–2791.https://doi.org/10.1172/JCI123825
-
Vascular Ion Channels in Physiology and DiseaseEndothelial cell ion channel expression and function in arterioles and resistance arteries, Vascular Ion Channels in Physiology and Disease, 3, Springer, 10.1007/978-3-319-29635-7_1.
-
Evidence for a functional role of endothelial transient receptor potential V4 in shear stress-induced vasodilatationArteriosclerosis, Thrombosis, and Vascular Biology 26:1495–1502.https://doi.org/10.1161/01.ATV.0000225698.36212.6a
-
TRPP2 and TRPV4 form a polymodal sensory channel complexJournal of Cell Biology 182:437–447.https://doi.org/10.1083/jcb.200805124
-
Carbon Monoxide and hydrogen sulfide: gaseous messengers in cerebrovascular circulationJournal of Applied Physiology 100:1065–1076.https://doi.org/10.1152/japplphysiol.00793.2005
-
Polycystin-2 cation channel function is under the control of microtubular structures in primary cilia of renal epithelial cellsJournal of Biological Chemistry 281:37566–37575.https://doi.org/10.1074/jbc.M603643200
-
TRPC3 is involved in flow- and bradykinin-induced vasodilation in rat small mesenteric arteries1Acta Pharmacologica Sinica 27:981–990.https://doi.org/10.1111/j.1745-7254.2006.00354.x
-
PLA2 and TRPV4 channels regulate endothelial calcium in cerebral arteriesAmerican Journal of Physiology. Heart and Circulatory Physiology 292:H1390–H1397.https://doi.org/10.1152/ajpheart.01006.2006
-
Increased catecholamine secretion contributes to hypertension in TRPM4-deficient miceJournal of Clinical Investigation 120:3267–3279.https://doi.org/10.1172/JCI41348
-
TRPV4-mediated endothelial Ca2+ influx and vasodilation in response to shear stressAmerican Journal of Physiology. Heart and Circulatory Physiology 298:H466–H476.https://doi.org/10.1152/ajpheart.00854.2009
-
Cellular signaling and NO productionPflügers Archiv - European Journal of Physiology 459:807–816.https://doi.org/10.1007/s00424-009-0765-9
-
A pathogenic C terminus-truncated polycystin-2 mutant enhances receptor-activated Ca2+ entry via association with TRPC3 and TRPC7Journal of Biological Chemistry 284:34400–34412.https://doi.org/10.1074/jbc.M109.015149
-
PKD1 interacts with PKD2 through a probable coiled-coil domainNature Genetics 16:179–183.https://doi.org/10.1038/ng0697-179
-
Handbook of Experimental Pharmacology675–711, The TRPP subfamily and polycystin-1 proteins, Handbook of Experimental Pharmacology, 222, Springer, 10.1007/978-3-642-54215-2_27.
-
Structure of the human PKD1-PKD2 complexScience 361:eaat9819.https://doi.org/10.1126/science.aat9819
-
TRP channel ca(2+) sparklets: fundamental signals underlying endothelium-dependent hyperpolarizationAmerican Journal of Physiology. Cell Physiology 305:C999–C1008.https://doi.org/10.1152/ajpcell.00273.2013
-
Functional characterization of PKDREJ, a male germ cell-restricted polycystinJournal of Cellular Physiology 209:493–500.https://doi.org/10.1002/jcp.20755
-
Autosomal dominant polycystic kidney diseaseThe Lancet 369:1287–1301.https://doi.org/10.1016/S0140-6736(07)60601-1
-
Ambulatory blood pressure and left ventricular mass in normotensive patients with autosomal dominant polycystic kidney diseaseJournal of the American Society of Nephrology : JASN 10:1020–1026.
-
The croonian lecture, 1993. the endothelium: maestro of the blood circulationPhilosophical Transactions of the Royal Society of London. Series B, Biological Sciences 343:225–246.https://doi.org/10.1098/rstb.1994.0023
-
Endothelial cation channel PIEZO1 controls blood pressure by mediating flow-induced ATP releaseJournal of Clinical Investigation 126:4527–4536.https://doi.org/10.1172/JCI87343
-
Acetylcholine released by endothelial cells facilitates flow-mediated dilatationThe Journal of Physiology 594:7267–7307.https://doi.org/10.1113/JP272927
-
Shear stress-initiated signaling and its regulation of endothelial functionArteriosclerosis, Thrombosis, and Vascular Biology 34:2191–2198.https://doi.org/10.1161/ATVBAHA.114.303422
Article and author information
Author details
Funding
National Institutes of Health (HL133256)
- Jonathan H Jaggar
National Institutes of Health (HL137745)
- Jonathan H Jaggar
American Heart Association (16SDG27460007)
- Simon Bulley
American Heart Association (15SDG22680019)
- M Dennis Leo
American Heart Association (20POST35210200)
- Charles E MacKay
American Heart Association (16POST30960010)
- Raquibul Hasan
The funders had no role in study design, data collection and interpretation, or the decision to submit the work for publication.
Acknowledgements
This study was supported by NIH/NHLBI grants HL133256 and HL137745 to JHJ, American Heart Association (AHA) Scientist Development Grants to SB (16SDG27460007) and MDM (15SDG22680019) and AHA Postdoctoral Fellowships to C M (20POST35210200) and R H (16POST30960010).
Ethics
Animal experimentation: All procedures were approved by the Animal Care and Use Committee of the University of Tennessee (protocol 17-068.0).
Copyright
© 2020, MacKay et al.
This article is distributed under the terms of the Creative Commons Attribution License, which permits unrestricted use and redistribution provided that the original author and source are credited.
Metrics
-
- 2,095
- views
-
- 356
- downloads
-
- 33
- citations
Views, downloads and citations are aggregated across all versions of this paper published by eLife.
Citations by DOI
-
- 33
- citations for umbrella DOI https://doi.org/10.7554/eLife.56655